DOI:
10.1039/B913828E
(Paper)
Green Chem., 2010,
12, 87-93
Hydrogenation of nitrile in supercritical carbon dioxide: a tunable approach to amine selectivity
Received 10th July 2009, Accepted 15th September 2009
First published on 23rd October 2009
Abstract
The use of supercritical carbon dioxide (scCO2) on the hydrogenation of benzonitrile was investigated over Pd and other metal catalysts. Without any additive, benzonitrile was hydrogenated to benzylamine with high conversion (90.2%) and selectivity (90.9%) using the Pd/MCM-41 catalyst. A strong influence of CO2 pressure on the conversion and selectivity were observed. As the CO2 pressure increases, the conversion was increased, and after reaching the maximum at around 8–10 MPa, it decreased. Moreover, simply by tuning the CO2 pressure, it is possible to obtain benzylamine or dibenzylamine. For instance, at lower pressure CO2 acts as a protecting agent, leading to the formation of the primary amine, but at higher pressure, the yield of primary amine as well as the solubility of the imine intermediate in CO2 increases, which results high selectivity for dibenzylamine. A plausible mechanism has been proposed to show the role of CO2 on the selectivity toward primary and secondary amines. The results confirm that the presence of CO2 is mandatory for the formation of benzylamine with high selectivity. Furthermore, the other reaction parameters, such as reaction time, H2 pressure, temperature etc., also affect the conversion as well as selectivity of benzylamine. This process has been extended to the hydrogenation of a series of different nitrile compounds.
Introduction
Supercritical carbon dioxide (scCO2) is a promising “green” reaction medium for rapid and selective organic synthesis. Over the past few years, a number of heterogeneously-catalyzed reactions have been successfully carried out in scCO2 medium, often with higher reaction rates and different product distributions, as well as high selectivity, compared to those in conventional organic solvents.1 The origin of the observed selectivity is of importance in view of the current developments on the understanding of the solvent attributes2 and the interesting supramolecular interactions3 reported for CO2. It has great potential to overcome disadvantages associated with conventional homogeneous and heterogeneous catalysts, such as mass transfer limitation, which frequently occur between reactants in the gas phase and liquid phase. The reactions in CO2 are tuned mainly by the pressure and temperature. Chemical interaction of CO2 with substrate or catalyst offers an attractive potential for selectivity control.4 For example, amines could react with CO2 to form carbamic acid or ammonium carbamate. It is thus reasonable to consider the possibility of protecting the amine group, which can improve the selectivity of a reaction, and also prevent catalyst deactivation.The hydrogenation of nitrile to amine is an industrially important process and generally carried out in the liquid phase with elevated hydrogen pressure.5 Amines are used as intermediates for the pharmaceutical, agrochemical and plastics industries. For example, benzylamine is utilized in several drugs like vitamin H (biotin), in the production of amide, isocyanate, intermediates for photographic material.6 On the other hand, dibenzylamine is used as a corrosion inhibitor, production of gasoline additives, pharmaceuticals, and rubber and tire compounding in the manufacture of SBR rubbers.7–9
The hydrogenation of benzonitrile has been studied over homogeneous10 and heterogeneous11 catalysts. For homogenous catalysts, the selectivity for the primary amine was high, but involved the difficulty of catalyst/product separation. Compared to the homogeneous catalysts, heterogeneous catalysts exhibit poor selectivity towards the primary amine. Thus, application of NH3 to increase the selectivity for the primary amine is the most used technique12 for heterogeneously-catalyzed reactions. However, NH3 has several disadvantages such as pressurized storage and the amount required is large, which results environmental and economical concern. Furthermore, different acids such as HCl, CH3COOH, and acid anhydride were used over Pd/C or RANEY® Ni catalysts13 to achieve high selectivity for the primary amine. Besides that, high temperature and harsh reaction conditions, and the use of different types of metal like Ni, Co, Fe, Pt, Pd, Ru and Rh are also part of the selectivity enhancement for the primary amine.14 Volf and Pasek15 reviewed the hydrogenation of nitrile in the liquid phase and indicated that Ni and Co are the best choices for producing primary amine, but require some additive. Recently, Hegedűs et al.15 also accomplished high selectivity of benzylamine (95%) with the use of NaH2PO4 as an acidic additive over 10% Pd/C catalyst in dichloromethane–water. Clearly, the use of additives to increase selectivity creates a waste problem (if the additives cannot be recycled), makes the process more expensive, and interferes with product separation, thus reducing the efficiency of the catalyst after certain runs. Hence, it is difficult to implement such a process at an industrial scale.
Instead of the liquid phase hydrogenation, Xie et al. used supercritical carbon dioxide at 3 MPa + ethanol (gas expanded liquid) medium, using heterogeneous (solution of NiCl2/NaBH4 catalyst) and homogeneous (RhH(P-i-Pr3)3) catalysts for benzonitrile hydrogenation16 and explained the protective role of CO2. It has to be mentioned that the use of expanded solvent enhanced the amount of organic substances used in the reaction (possibly leading to more waste production) but reduces the amount of pressure required and is sometimes preferable for industrial purposes.
The objective of our work is to carry out the hydrogenation of benzonitrile in supercritical carbon dioxide (scCO2) over a heterogeneous catalyst, without using any additive or any organic solvent.
Results and discussion
Scheme 1 depicts the possible reaction path of the hydrogenation of benzonitrile in scCO2. Benzonitrile is hydrogenated to benzylamine followed by the formation of dibenzylazamine with the elimination of NH3, depending on the reaction conditions.The nature of the active metal ion and catalyst supports are the most important parameters to describe the activity and the product distribution of a specific reaction. Thus, at first, the hydrogenation of benzonitrile was carried out over different catalysts, and the results are shown in Table 1. From the results, under the studied reaction conditions (catalysts = 0.1 g, substrate = 1.0 g; PCO2= 10 MPa, PH2 = 2 MPa, T = 50 °C, reaction time = 4 h), Pd catalysts appear more efficient than any other metal for benzonitrile hydrogenation. On the other hand, the conversions of benzonitrile on Pt, Rh and Ni catalysts are low, between 15–30% (Table 1, entries 4, 5 and 9), whereas the conversions over Pd catalysts are comparatively high, reaching 100% for Pd/C catalyst (Table 1, entry 1). However, the most impressive selectivity of primary amine (90.9%) was obtained over Pd/MCM-41 (Table 1, entry 3) and secondary amine was formed exclusively over Pd/C. Hence, we have chosen Pd/MCM-41 for further study and the results will be compared with the Pd/C catalyst in the following section. Notably, no reaction proceeded without catalyst.
Entry | Catalyst | Conversion/% | Selectivity/% | TOF/h−1a |
---|
Benzylamine | Dibenzylamine |
---|
Reaction conditions: catalyst = 0.1 g, substrate = 1.0 g; PCO2 = 10 MPa, PH2= 2 MPa, T = 50 °C, reaction time = 4 h. Turnover frequency (TOF) = number of moles reacted/moles of metal × time. |
---|
1 | 1% Pd/C | 100 | 0.0 | 100.0 | 2983 |
2 | 5% Pd/Al2O3 | 46.0 | 50.0 | 50.0 | 340.4 |
3 | Pd/MCM-41 | 90.2 | 90.9 | 9.1 | 4151 |
4 | 5% Pt/C | 8.5 | 0.0 | 100.0 | 178.8 |
5 | Pt/MCM-41 | 20.6 | 19.8 | 80.2 | 552.5 |
6 | 5% Rh/C | 28.8 | 25.0 | 75.0 | 180.6 |
7 | 5% Rh/Al2O3 | 21.0 | 22.4 | 77.6 | 291.3 |
8 | Rh/MCM-41 | 5.1 | 25.0 | 75.0 | 42.4 |
9 | Ni/MCM-41 | 15.7 | 37.5 | 62.5 | 5.1 |
Recycle 1 | Pd/MCM-41 | 90.4 | 91.0 | 9.0 | — |
Recycle 2 | | 89.9 | 90.9 | 9.1 | — |
Recycle 3 | | 89.8 | 90.8 | 9.2 | — |
Recycle 4 | | 90.0 | 90.8 | 9.2 | — |
Recycle 5 | | 88.5 | 90.5 | 9.5 | — |
Recycle 1 | Pd/C | 96.4 | 0.0 | 100.0 | — |
Recycle 2 | | 82.1 | 0.0 | 100.0 | — |
Effect of CO2 pressure on benzonitrile hydrogenation
Fig. 1a and b show the effect of CO2 pressure on the hydrogenation of benzonitrile using Pd/MCM-41 and Pd/C catalysts, respectively, at 50 °C with constant hydrogen pressure of 2 MPa. The results show that increasing CO2 pressure from 8 to 10 MPa, leads to an increase in the conversion of benzonitrile from 64.9 to 90.2%, but a significant decrease in conversion to 51.5% was observed when the pressure reaches 14 MPa. This scenario can be explained by visual observation of the phase behavior of CO2 and reactants through the view cell. In the temperature and pressure range considered here, the reaction mixture exists as a gas–liquid (hydrogen and CO2–substrate) biphase. In the low pressure region, the reactants mainly exist in the liquid phase and mass transfer limitation of H2 causes low conversion. With increasing CO2 pressure, the density and solubility of the reactants increase and, accordingly, conversion of benzonitrile increases. However, further increase of the pressure at a constant volume forces more molecules of CO2 between the reactant molecules, thus mimicking the enhanced dilution effects of the conventional solvent, and conversion decreases. The selectivity for primary amine was also strongly influenced by the CO2 pressure. With increasing CO2 pressure, the selectivity was enhanced from 64 to 90.9% (10 MPa), decreasing to 20%, when the pressure reaches 14 MPa. Thus, in the higher pressure region, the product distribution changes and dibenzylamine becomes the primary product, with ∼95% selectivity. The change in selectivity from benzylamine to dibenzylamine with CO2 pressure can be accounted for as follows. It was proposed that the hydrogenation of nitriles (1) occurs in steps:15,17 in the first step, the primary amine (3) is obtained from the imine intermediate (2) followed by the formation of secondary amine (7) through the nucleophilic attack of the primary amine (3) on the imine (2). Here, we propose a reaction scheme to explain the product selectivity depending on the reaction condition, mainly the CO2 pressure (Scheme 2). To describe the reaction mechanism, the following points need to be considered: (i) results obtained over the Pd/MCM-41 catalyst are the basis for describing the mechanism because of the exclusive formation of 3, which is the main interest of this work, (ii) the experimental evidence (see Catalyst screening section) showed that the presence of catalyst was essential for the reaction to occur, and (iii) significant information on product selectivity can be obtained from phase behavior studies of 1 and products. It has to be mentioned that after calcination in air, Pd mainly exists as Pd(II) in the Pd/MCM-41 catalyst. From the mechanism perspective, the reaction may start with an oxidative addition between 1 and the catalyst,18 which results in the formation of chemisorbed species 1a. The presence of H2 would afford the generation of catalytically active Pd(0). Now, the phase behavior of 1 revealed that in the lower pressure region, the reaction mixture exists as a gas (CO2 and H2)–liquid (substrate) biphase. Thus, more 1 was available in the liquid phase rather than in the gas phase. As a result, mass transfer limitations take place and decrease the conversion of 1. Therefore, the formation of 3 was very low, which can readily react with the imine 2 and 7 was formed. On the other hand, when CO2 pressure increased, the solubility of 1 in CO2 was enhanced and the reaction mixture changes from a biphase to a single phase, where maximum selectivity of 3 was observed. This scenario can be explained by path A of Scheme 2. The phase behavior study of 3 (as described in the Experimental section) suggested the formation of carbamate 4 was preferred when PCO2 < 12 (Fig. 4a), which could improve the selectivity of 3 by preventing the formation of 7 because 4 cannot act as a nucleophile to interact with 2. Alternatively, at higher pressure (PCO2≥ 12 MPa; Fig. 4b), 3 exhibited solubility in CO2 and favored the nucleophilic addition between 2 and 3 (because the imine also has high solubility in CO2), followed by the formation of 7 through path B. This observation agrees with the results of Goemz et al., which suggested that the condensation reaction proceeds homogeneously.11 Hence, simply by tuning the CO2 pressure, it is possible to tune the selectivity from 3 to 7.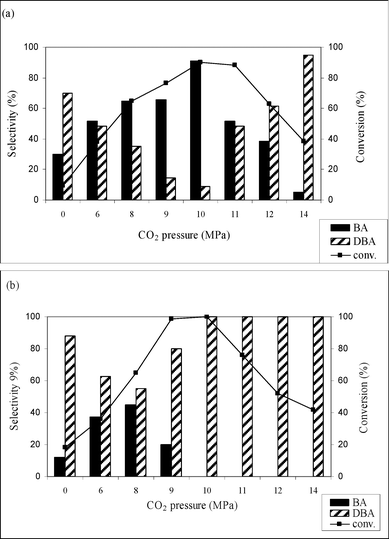 |
| Fig. 1 Effect of CO2 pressure on the conversion and selectivity of benzonitrile hydrogenation over (a) Pd/MCM-41 and (b) Pd/C. Reaction conditions: catalyst = 0.1g, substrate = 1.0 g, T = 50 °C, PH2= 2 MPa, time = 4 h. | |
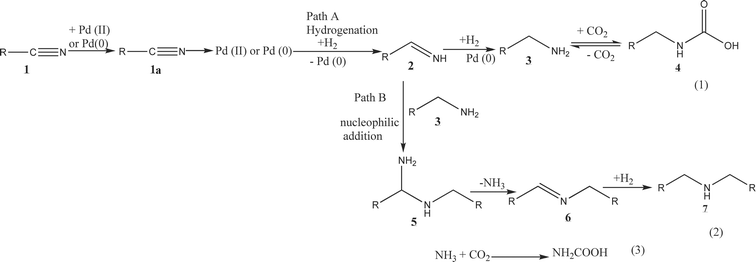 |
| Scheme 2 Proposed reaction mechanism of benzonitrile hydrogenation depending on the reaction conditions. | |
Fig. 1a also depicts the conversion and the selectivity of amines in solvent-free conditions. The conversion of benzylamine was low (∼10%) with dibenzylamine as the main product, which is attributed to the presence of CO2 being necessary for primary amine formation.
Similarly, Fig. 1b reveals that the Pd/C catalyst was selective to the dibenzylamine. The highest selectivity (44.7%) for primary amine was achieved at 8 MPa but decreases to 0.0% as the pressure increases to 14 MPa. Following these results, the reaction was conducted without CO2 (Fig. 1b) and a striking decrease in the conversion (18%) was evident, probably due to mass transfer limitations.
This difference in activity between the Pd/MCM-41 and Pd/C catalysts under the same reaction conditions may originate from the support effect. It is well-known that a C support is much more acidic compared to MCM-41.19 The effect of support acidity on the hydrogenation of nitrile is a controversial issue. Some authors have claimed that acidity favors the formation of secondary amine, while others report no effect on selectivity. The results presented here suggest that the effect of CO2 pressure is more prominent for secondary amine formation when an acidic support was used. Therefore, the role of acid sites to initiate the condensation between primary amine and the imine cannot be ignored.20
Effect of H2 pressure on benzonitrile hydrogenation
Fig. 2a and b present the effect of change in hydrogen pressure from 1–4 MPa, on the hydrogenation of benzonitrile using Pd/MCM-41 and Pd/C catalysts, respectively. The temperature and CO2 pressure were kept constant at 50 °C and 10 MPa, respectively, in accordance with the highest observed activity and selectivity. As expected, the conversion of benzonitrile was increased from 44.2 to 95% with an increase in hydrogen pressure from 1 to 4 MPa over Pd/MCM-41, but the selectivity for benzylamine was reduced to 60%. In a similar way, increasing the hydrogen pressure from 1–4 MPa also changes the conversion for the Pd/C catalyst, from 41.9 to 100%. However, the selectivity for benzylamine drops from 70 to 0%. Thus, according to the selectivity for benzylamine, 2 MPa was chosen as optimum pressure for both of the catalysts for benzonitrile hydrogenation.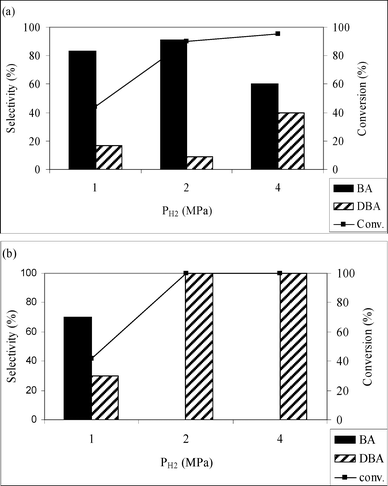 |
| Fig. 2 Effect of variation of H2 pressure on the hydrogenation of benzonitrile over (a) Pd/MCM-41 and (b) Pd/C. Reaction conditions: catalyst = 0.1g, substrate = 1.0 g, T = 50 °C, PCO2 = 10 MPa, time = 4 h. | |
Reaction time
It is well-known that the product distribution of a reaction is closely related to the working conditions of the catalytic system and the stability of the intermediate species on the catalyst surface. Reaction time is an important parameter which can provide an idea about the kinetics of the reaction. The effect of reaction time has also been studied over Pd/MCM-41 and Pd/C for benzonitrile hydrogenation. Fig. 3a and b show the change in catalytic activity with time. Evidently, the rate of hydrogenation was faster on Pd/C compared to Pd/MCM-41. For instance, within the fixed reaction time of 2 h, 74 and 44% conversion was achieved over Pd/C and Pd/MCM-41, respectively.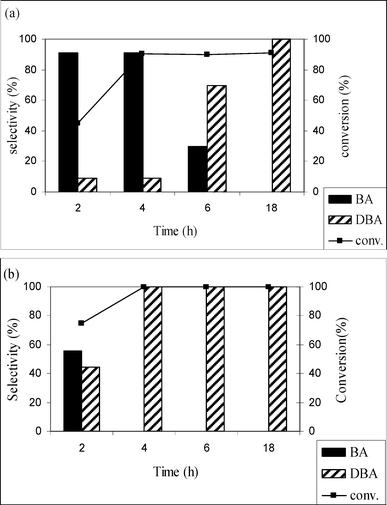 |
| Fig. 3 Effect of variation of reaction time on benzonitrile hydrogenation over (a) Pd/MCM-41 and (b) Pd/C. Reaction conditions: catalyst = 0.1g, substrate = 1.0 g, T = 50 °C, PCO2 = 10 MPa, PH2= 2 MPa. | |
However, the calculation of the turnover frequency (TOF) changes the scenario. The calculated TOF over the Pd/MCM-41 catalyst within 2 h is 4138 h−1, but changes to 4151 h−1 after 4 h, whereas the Pd/C catalyst exhibits a TOF of 4445 h−1 in 2 h, which decreases to 2983 h−1 as the reaction time was extended to 4 h. This decrease in TOF for the Pd/C catalyst during the course of the reaction might be related to the deactivation of the catalyst.
Effect of temperature
The effect of temperature on the hydrogenation of benzonitrile was studied over Pd/MCM-41 and Pd/C catalysts at 35, 50 and 70 °C, with constant H2 and CO2 pressures of 4 and 10 MPa, respectively. As expected, the rate of the reaction was enhanced as the temperature changes from 35 to 70 °C, accompanied by decreased selectivity for benzylamine from 91.6 to 40% on Pd/MCM-41. For Pd/C, the conversion of benzonitrile, as well as selectivity for dibenzylamine, also increased along with the temperature.For both cases, the influence of reaction temperature on conversion and selectivity can be explained by the phase behavior of the substrate–hydrogen–CO2 system. In scCO2, the alteration of temperature changes the density of the medium and there is a possibility of a single phase separating into two phases, because the CO2 phase become less dense at higher temperatures. Thus, at lower densities, scCO2 is more gas-like and, therefore, a poorer solvent. The increase in conversion with temperature may be attributed to the dramatic increase in the reaction rate in the liquid phase and also in the gaseous phase, which more than compensates for the negative effect of phase separation.21
The optimized reaction conditions (50 °C, 4 h, PCO2 = 10 MPa and PH2 = 4 MPa) of benzonitrile has been applied to the hydrogenation of different nitrile substrate and the results are shown in Table 2. These results show that the application of the Pd/MCM-41 catalyst can be extended to nitriles with different functional groups. For example, –CH3, –Br and –OMe (Table 2, entries 1–3) produce the corresponding primary amines with high selectivities, between 65–100%. For aromatic nitriles, a clear trend in conversion and selectivity was found, depending on the presence of electron-withdrawing and electron-donating groups. From the results, it can be seen that in the presence of an electron-donating group, the conversion was high, but the selectivity for the primary amine was low (Table 2, entries 1 and 2). The situation is reversed in the presence of an electron-withdrawing group, however (Table 2, entries 3 and 4). The potential of the catalyst was also described by the hydrogenation of aliphatic nitrile (Table 2, entries 6–8). It has to be mentioned that in comparison with the aromatic nitriles, higher temperatures were needed for the conversion of benzyl cyanide and 3-phenylpropionitrile (Table 2, entries 5 and 6) to their corresponding primary amines, with selectivities of 98.3 and 80.1%, respectively. No additive was used to increase the yield of the primary amine for any of the above-mentioned substrates.
Entry | Substrate | Conversion/% | Selectivity of Primary amine/% |
---|
Reaction conditions: catalyst = 0.1 g, substrate = 1.0 g, T = 50 °C, PCO2= 10 MPa, PH2 = 2 MPa, time = 4h. T = 70 °C. |
---|
1 | 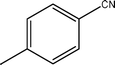 | 89.2 | 82.1 |
2 | 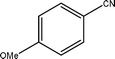 | 80.1 | 75.2 |
3 | 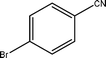 | 64.5 | 100.0 |
4 |  | 61.5 | 100.0 |
5a | 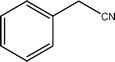 | 81.5 | 98.3 |
6a | 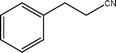 | 94.5 | 80.1 |
7 | 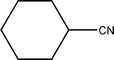 | 98.6 | 100.0 |
8 |  | 60.5 | 100.0 |
As heterogeneous catalysts have claimed to offer the advantage of recycling, we separated the catalysts for reuse by simple filtration. The results up to the fifth recycle are shown in Table 1 and suggest that the reuse of catalyst was possible for Pd/MCM-41. This fact could be justified by considering the high solubility of the imine intermediate in scCO2, which is one main reason for catalyst deactivation during nitrile hydrogenation by the blocking of metal sites. However, the Pd/C catalyst shows deactivation after the first recycle.Conclusions
In conclusion, it has been demonstrated that scCO2 is a potential medium to facilitate the hydrogenation of benzonitrile to the corresponding primary amine. Without using any additive, high conversion (90.2%) and selectivity for the primary amine (∼91%) were achieved over the Pd/MCM-41 catalyst. The product distribution was found to depend on different reaction parameters such as CO2 and H2 pressure, temperature and the nature of the support. Simple tuning of the CO2 pressure resulted in the formation of the secondary amine, dibenzylamine, which was assisted by the interaction of CO2 with the reactant and also by the acidic support of C, instead of neutral MCM-41. In the studied experimental conditions, Pd/C was deactivated easily, whereas Pd/MCM-41 can be recycled. Preliminary experiments with other substituted nitriles show a strong influence of the nature of the substituted group on the activity and selectivity. The simple methodology presented here could be highly relevant for further development of a clean chemical process for hydrogenation of nitriles.Experimental
Materials
Benzonitrile (Wako Pure Chemicals) was used as received. Carbon dioxide (>99.99%) was supplied by Nippon Sanso Co. Ltd. The 1% Pd/C, 5% Pd/Al2O3, 5% Pt/C and 5% Rh/C were from Aldrich, 5% Rh/Al2O3 from Wako Pure chemicals and Pd, Pt, Ni and Rh/MCM-41 were synthesized in our lab.The hydrogenation of benzonitrile was studied at 50 °C over 1% Pd/MCM-41 catalyst. All reactions were carried out in a 50 ml stainless steel batch reactor placed in a hot air circulating oven. The details are given elsewhere.22 Briefly, 0.1 g of catalyst and 1.0 g of the reactant were introduced into the reactor. After the required temperature was attained, H2, followed by CO2, was charged into the reactor using a high-pressure liquid pump and then compressed to the desired pressure. The product liquid was separated from the catalyst simply by filtration and identified by NMR and GC-MS, followed by quantitative analysis using a GC (HP 6890) equipped with capillary column and flame ionization detector. For all results reported, the selectivity is as follows:
Phase behavior
The phase behavior of benzonitrile was studied in a 10 ml high pressure view cell fitted with a sapphire window. The cell is placed over a magnetic stirrer and connected to a pressure controller, to regulate the pressure inside the view cell. In addition, a temperature controller was also used to maintain the desired temperature of 50 °C. The substrate was introduced into the view cell at a constant hydrogen pressure of 2 MPa while CO2 pressure was varied between 7–14 MPa and the phase behavior was monitored. The reaction mixture exhibited a biphase at P < 10 MPa, but with increasing CO2 pressure the solubility of benzonitrile increases and a single phase was reached. The phase behavior of benzylamine and dibenzylamine was also checked. Benzylamine showed an interesting behavior as described in Fig. 4, which represents the carabamate formation at P < 12 MPa (Fig. 4a) and solubility at higher pressure (P≥ 12 MPa; Fig. 4b). Similarly, dibenzylamine also shows solubility in CO2 at higher pressure.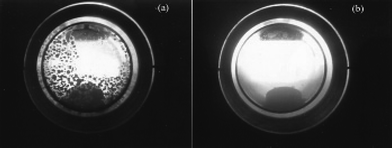 |
| Fig. 4 Phase behavior study of benzylamine (a) P < 12 MPa (b) P≥ 12 MPa. | |
Notes and references
- Green Chemistry using Liquid and Supercritical Carbon Dioxide, ed. J. M. De Simone, W. Tumas, Oxford Press, 2003 Search PubMed; Modern Solvent in Organic Synthesis, ed. P. Knochel, Springer, 1999 Search PubMed; A. Baiker, Chem. Rev., 1999, 99, 453 Search PubMed; A. Fürstner, L. Ackermann, K. Beck, H. Hori, D. Koch, K. Langeman, M. Liebl, C. Six and W. Leitner, J. Am. Chem. Soc., 2001, 123, 9000 Search PubMed; K. Wittmann, W. Wisniewski, R. Mynott, W. Leitner, C. L. Kranemann, T. Rische, P. Eilbracht, S. Kluwer, J. M. Ernsting and C. J. Elsevier, Chem.–Eur. J., 2001, 7, 4584 CrossRef CAS; P. Stephenson, B. Kondor, P. Licence, K. Scovell, S. K. Ross and M. Poliakoff, Adv. Synth. Catal., 2006, 348, 1605 CrossRef CAS; J. R. Hyde and M. Poliakoff, Chem. Commun., 2004, 1482 CrossRef CAS; J. R. Hyde, B. Walsh, P. Singh and M. Poliakoff, Green Chem., 2005, 7, 357 CrossRef CAS.
- S. G. Kazarian, M. F. Vincent, F. V. Bright, C. L. Liotta and C. A. Eckert, J. Am. Chem. Soc., 1996, 118, 1729 CrossRef CAS; P. Raveendran and S. L. Wallen, J. Am. Chem. Soc., 2002, 124, 12590 CrossRef; P. Raveendran, Y. Ikushima and S. L. Wallen, Acc. Chem. Res., 2005, 38, 478 CrossRef CAS; L. Reynolds, J. A. Gardecki, S. J. V. Frankland, M. L. Horng and M. Maroncelli, J. Phys. Chem., 1996, 100, 10337 CrossRef CAS; S. Saharay and S. Balasubramanian, ChemPhysChem, 2004, 5, 1442 CrossRef.
- D. M. Rudkevitch, Angew. Chem., Int. Ed., 2004, 43, 558 CrossRef CAS.
- W. Leitner, Angew. Chem., 1995, 107, 2391 CrossRef; W. Leitner, Angew. Chem., Int. Ed. Engl., 1995, 34, 2207 CrossRef CAS; M. Chatterjee, A. Chatterjee, P. Raveendran and Y. Ikushima, Green Chem., 2006, 8, 445 RSC.
- Ullmann's Encyclopedia of Industrial Chemistry, fifth rev. edn, Vol. A2, VCH Verlag, Weinheim, 1985, p. 1 Search PubMed.
- A. Kleemann, J. Engel, B. Kutscher, D. Reichert, Pharmaceutical Substances: Syntheses, Patents, Applications, fourth edn, Stuttgart Georg Verlag, New York, 2001 Search PubMed.
- B. Wanderott, Z. Metallkd., 1965, 56, 63 Search PubMed.
- S. Gomez, J. A. Peters, J. C. Van der Waal, W. Zhou and T. Maschmeyer, Catal. Lett., 2002, 84, 1 CrossRef CAS.
- D. R. Buehler, G. P. Keister, I. F. Long, Du Pont 1969 US Pat. 3 461 167.
- C. Bianchini, V. Dal Santo, A. Meli, W. Oberhauser, R. Psaro and F. Vizza, Organometallics, 2000, 19, 2433 CrossRef CAS; C. S. Chin and B. N. Lee, Catal. Lett., 1992, 14, 135 CrossRef CAS; S. Nishimura, Handbook of Heterogeneous Catalytic Hydrogenation for Organic Synthesis, John Wiley & Sons, New York, 2001, Chapter 7, p 254 Search PubMed; T. Li, I. Bergner, F. Nipa Haque, M. Zimmer De-Iuliis, D. Song and R. H. Morris, Organometallics, 2007, 26, 5940 Search PubMed; S. Enthalaer, D. Addis, K. Junge, G. Erre and M. Beller, Chem.–Eur. J., 2008, 14, 9491 CrossRef CAS.
- P. Kukula, M. Studer and H. U. Blaser, Adv. Synth. Catal., 2004, 346, 1487 CrossRef CAS; H. U. Blaser, C. Malan, B. Pugin, F. Spindler, H. Steiner and M. Studer, M., Adv. Synth. Catal., 2003, 345, 103 CrossRef CAS; S. Gomez, J. A. Peters and T. Maschmeyer, Adv. Synth. Catal., 2002, 344, 1037 CrossRef CAS.
- F. Winnas, J. Am. Chem. Soc., 1939, 61, 3566 CrossRef; A. M. C. F. Castelijns, P. J. D. Maas, EP Patent 0644177, 1995C. F. Winans, US Patent 2,217,630, 1940.
- W. H. Hartung, J. Am. Chem. Soc., 1928, 50, 3370 CrossRef CAS; E. Miller, J. M. Sprague, L. W. Kissinger and L. F. McBurney, J. Am. Chem. Soc., 1940, 62, 2099 CrossRef CAS; W. H. Carothers and G. A. Jones, J. Am. Chem. Soc., 1925, 47, 3051 CrossRef CAS.
- S. Galvagno, A. Donato, G. Neri and R. Pietropaolo, J. Mol. Catal., 1990, 58, 215 CrossRef CAS; C. V. Rode, M. Arai, M. Shirai and Y. Nishiyama, Appl. Catal., A, 1997, 148, 405 CrossRef CAS.
- Volf and J. Pasek, Stud. Surf. Sci. Catal., 1986, 27, 105; L. Hegedűs and T. Máthé, Appl. Catal., A, 2005, 296, 209 CrossRef.
- X. Xie, C. L. Liotta and C. A. Eckert, Ind. Eng. Chem. Res., 2004, 43, 7907 CrossRef CAS.
- J. von Braun, J., G. Blessing and F. Zobel, Chem. Ber., 1923, 36, 1988; C. de Bellefon and P. Fouilloux, Catal. Rev. Sci. Eng., 1994, 36, 459 CrossRef CAS; H. Greenfield, Ind. Eng. Chem. Prod. Res. Dev., 1967, 6, 142 Search PubMed; L. D. Volkova, H. B. Kagaarlickaya and G. D. Zakumbaeva, Izv. Akad. Naukkaz. SSR., Ser; Khim., 1970, 23, 70 Search PubMed; J. L. Dallons, A. Van Gysel and G. Jannes, in “Catalysis of Organic Reactions” (W. E. Pascoe, Ed.), p. 93, Dekker, New York, 1992 Search PubMed.
- Y. Huang, W. M. H. Schtler and J. Catal, J. Catal., 1999, 184, 247 CrossRef CAS; A. J. Deeming, D. W. Owen and N. I. Powell, J. Organomet. Chem., 1990, 398, 299 CrossRef CAS; C. M. P. Ferreria, M. F. C. Guedes de Silva, V. Y. Kukushkin, J. J. R. Fraúto da Silva and A. J. L. Pombeiro, J. Chem. Soc., Dalton Trans., 1998, 325 RSC; H. Tsutsui and K. Narasaka, Chem. Lett., 1999, 45 CrossRef CAS.
- J. Hájek, N. Kumar, P. Mäki-Arvela, T. Salmi, D. Yu. Murzin, I. Paseka, T. Heikkilä, E. Laine, P. Laukkanen and J. Väyryne, Appl. Catal., A, 2003, 251, 385 CrossRef CAS.
- F. M. Cabello, D. Tichit, B. Coq, A. Vaccari and N. T. Dung, J. Catal., 1997, 167, 142 CrossRef CAS.
- R. Tschan, R. Wandeler, M. S. Schneider, M. M. Schubert and A. Baiker, J. Catal., 2001, 204, 219 CrossRef CAS.
- M. Chatterjee, F. Y. Zhao and Y. Ikushima, Adv. Synth. Catal., 2004, 346, 459 CrossRef CAS; M. Chatterjee, A. Chatterjee and Y. Ikushima, Green Chem., 2004, 6, 114 RSC; M. Chatterjee, F. Zhao and Y. Ikushima, Appl. Catal., A, 2004, 262, 93 CrossRef CAS.
|
This journal is © The Royal Society of Chemistry 2010 |
Click here to see how this site uses Cookies. View our privacy policy here.