DOI:
10.1039/C0FO00134A
(Paper)
Food Funct., 2010,
1, 301-307
Garcinol inhibits cell growth in hepatocellular carcinoma Hep3B cells through induction of ROS-dependent apoptosis
Received
10th September 2010
, Accepted 11th October 2010
First published on
27th October 2010
Abstract
Garcinol, derived from Garcinia indica and other related species, has been found to modulate several cell signalling pathways involved in apoptosis and cancer development. Growth arrest and DNA damage-inducible gene 153 (GADD153) is a member of the CCAAT/enhancer-binding protein (C/EBP) family of transcription factors; it is expressed at low levels under normal conditions but strongly induced upon growth arrest, DNA damage, and endoplasmic reticulum (ER) stress. This study investigated the effect of garcinol on Hep3B cells, a human hepatocellular cancer cell line lacking functional p53, with the goal of elucidating the molecular mechanisms of p53-independent apoptosis in hepatocellular cancer. Overall, garcinol activated not only the death receptor and the mitochondrial apoptosis pathways but also the ER stress modulator GADD153. Garcinol treatment led to the accumulation of reactive oxygen species (ROS), increased GADD153 expression, and reduced mitochondrial membrane potential. An increase in the Bax/Bcl-2 ratio resulted in enhanced apoptosis. Caspase-8 and tBid (truncated Bid) expression also increased in a time-dependent manner. The enzymatic activities of caspase-3 and caspase-9 increased approximately 13-fold and 7.8-fold, respectively. In addition, the proteolytic cleavage of poly-(ADP-ribose)-polymerase (PARP) and DNA fragmentation factor-45 (DFF-45) increased in dose- and time-dependent manners. Our data suggest a promising therapeutic application of garcinol in p53-independent apoptosis in cancers.
1. Introduction
Garcinol (Fig. 1A) is a polyisoprenylated benzophenone derived from Garcinia indica and other related species.1 The dried rind of G. indica (cv. Kokum) is used as a garnish in cooking and a constituent in folk medicine in India. Garcinol, similar to curcumin in structure, contains both a phenolic hydroxyl unit and a β-diketone moiety. Many studies have indicated that garcinol is a pleiotropic agent2 whose functions might include antioxidant activity,3 scavenging of free radicals,4 antibacterial activity,5 and inhibition of inflammation.6 Animal studies have shown that garcinol may inhibit colon tumorigenesis7 and 4-nitroquinoline 1-oxide-induced tongue carcinogenesis.8 In addition, our previous studies demonstrated that garcinol could inhibit cell growth in human leukemia HL-60 cells9 and attenuate cell proliferation in nicotine-induced human breast cancer.10 Others have found that garcinol is a potent inducer of apoptosis involved in the regulation of caspases,11 death receptors, and anti-apoptotic proteins.12 Furthermore, garcinol may modulate cell invasion,13 histone acetyltransferases, and global gene expression in cancer.14 Taken together, garcinol can modulate several cell signalling pathways involved in apoptosis and cancer development.
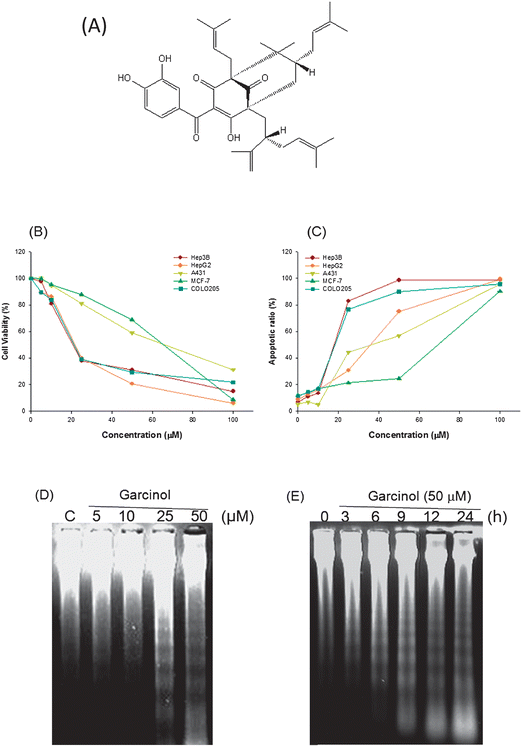 |
| Fig. 1 Garcinol induces apoptosis in human cancer cells. (A) Chemical structure of garcinol. (B, C) Garcinol inhibits cell viability and induces apoptosis in a dose-dependent manner. Hep3B, HepG2, A431, MCF-7, and COLO205 cells (2 × 105 cells per mL) were treated with various concentrations of garcinol for 24 h. Cell viability was determined by MTT assay as described in Materials and Methods. The quantification of sub-G1 cells and apoptotic ratios (%) of the cells were detected by flow cytometry. Each experiment was independently performed three times, and the results are expressed as the mean ± SE. (D, E) Induction of DNA fragmentation by garcinol. Hep3B cells were treated with either various doses of garcinol for 24 h or 50 μM garcinol for the indicated durations. Internucleosomal DNA fragmentation was analyzed by agarose electrophoresis. These experiments were performed at least in triplicate; a representative result is presented. | |
Hepatocellular cancer (HCC) is one of the most common cancers worldwide.15 Hepatocellular carcinoma typically occurs in patients with chronic liver diseases due to hepatitis B or C infection, alcohol abuse, or impaired differentiation function resulting in, for example, reduced albumin expression.15b,16 At present, some HCC patients are not eligible for surgical resection procedures due to the metastatic nature of the disease.17
In this study, we investigated the molecular mechanisms of apoptosis by treating Hep 3B cells with garcinol. The mechanism by which garcinol inhibits p53-negative cell proliferation is not clearly understood. The present study provides novel evidence that garcinol may have potent chemopreventive and antitumor effects in human hepatocellular carcinoma cells.
2. Materials and methods
2.1 Cell culture and chemicals
The human epidermoid carcinoma cell line A431 (American Type Culture Collection [ATCC]) was cultured in Dulbecco's modified Eagle's medium (Life Technologies, Inc.) supplemented with 10% fetal bovine serum. The Hep 3B and Hep G2 cell lines were derived from human HCC (ATCC HB-8064 and HB-8065). The COLO 205 cell line (CCL-222; American Type Culture Collection) was developed from a poorly differentiated human colon adenocarcinoma. The A431, Hep 3B, Hep G2, and MCF-7 cell lines were grown in Dulbecco's modified Eagle's medium, and the COLO 205 cells were grown in RPMI. Both media were supplemented with 10% heat-inactivated fetal bovine serum (Gibco, Grand Island, NY), 100 units per mL of penicillin, 100 μg mL−1 of streptomycin, and 2 mM l-glutamine (Gibco BRL). Cell lines were maintained at 37 °C in 5% carbon dioxide. Garcinol was isolated from the dried rind of G. indica9 to 98% purity. It was dissolved in dimethyl sulfoxide (DMSO). Propidium iodide was obtained from Sigma Chemical Co. (St. Louis, MO).
2.2. Cell viability assay
Cell viability was assayed by 3-(4,5-dimethylthiazol-2-yl)-2,5-diphenyltetrazolium bromide (MTT). Briefly, cells were plated into 24 well plates at a density of 1 × 105 cells per well. After overnight growth, cells were treated with a series of garcinol concentrations for 24 h. The final DMSO concentration in the culture medium was <0.05%. At the end of treatment, 30 μL of MTT was added, and the cells were incubated for 4 h. Cell viability was determined by scanning with an enzyme-linked immunosorbent assay reader at 570 nm (Dynatech MR-7000; Dynatech Laboratories, Chantilly VA).9
The cells were harvested, washed with PBS, resuspended in 200 μL of PBS, and fixed in 800 μL of 100% ethanol at −20 °C. After being left to stand overnight, the pellets were collected by centrifugation, resuspended in 1 mL of hypotonic buffer (0.5% Triton X-100 in PBS and 0.5 μg mL−1 RNase), and incubated at 37 °C for 30 min. Next, 1 mL of propidium iodide solution (50 μg mL−1) was added, and the mixture was allowed to stand in ice water for 30 min. Fluorescence emitted from the propidium iodide-DNA complex was quantitated by FACScan cytometry (Becton Dickinson, San Jose, CA). Quantitation of the number of cells in each stage of the cell cycle was performed with ModFit LT for Mac 3.0 software (Becton Dickinson, San Jose, CA).
2.4. Acridine orange staining assay
Cells (5 × 105) were seeded into 60-mm Petri dishes in RPMI-1640 medium and incubated at 37 °C for 24 h. After harvesting, 5 μL of suspended cells were mixed on a glass coverslip with an equal volume of acridine orange solution (10 μg mL−1 in PBS). Apoptotic cells, showing characteristic chromatic condensation, were easily distinguished from viable cells, showing homogeneously bright staining in the nucleus.
Hep 3B cells (5 × 105 cells per mL) were harvested, washed with PBS, and then lysed with digestion buffer (0.5% sarkosyl, 0.5% mg mL−1 proteinase K, 50 mM tris (hydroxy methyl) aminomethane (pH 8.0), 10 mM EDTA) at 56 °C for 3 h. Lysates were then treated with RNase A (0.5 μg mL−1) for another 2 h at 56 °C. The DNA was extracted by phenol/chloroform/isoamyl (25/24/1) and analyzed by 1.8% agarose gel electrophoresis. Approximately 20 μg of DNA was loaded into each well of an agarose gel and separated by electrophoresis at 50 V for 120 min in TBE (Tris-borate/EDTA electrophoresis buffer). Gels were visualized under UV light and photographed.
2.6. Determination of ROS production
ROS production was monitored by flow cytometry using dichlorodihydrofluorescein diacetate (DCFH-DA). This dye is a stable, nonpolar compound that readily diffuses into cells. It is hydrolyzed by intracellular esterase to yield DCFH, which remains trapped within the cells. Hep3B cells were treated with garcinol (50 μM) for 10 min. DCFH-DA (20 μM) and dihydroethidium (DHE) (20 μM) were added to the medium for a further 30 min at 37 °C. Histograms were analyzed using Cell Quest software and were compared with histograms of untreated control cells.18
2.7. Analysis of mitochondrial transmembrane potential
The change in the mitochondrial transmembrane potential was monitored by flow cytometry. Briefly, Hep3B cells were treated with garcinol (50 μM) for 15 min. The mitochondrial transmembrane potential was measured directly using 40 nM 3,3′-dihexyloxacarbocyanine [DiOC6(3)] (Molecular Probes, Eugene, OR). Fluorescence was measured after staining the cells for 30 min at 37 °C. Histograms were analyzed using Cell Quest software and were compared with histograms of untreated control cells.
Mitochondrial and cytosolic (S100) fractions were prepared as described previously.9 Briefly, after treatment with 50 μM of garcinol, cells were washed twice in ice cold PBS and then resuspended in homogenizing buffer (250 mM sucrose, 20 mM HEPES, 10 mM KCl, 1.5 mM MgCl2, 1 mM EDTA, 1 mM EGTA, 1 mM dithiolthione, 17 μg mL−1 phenylmethylsulfonyl fluoride, 8 μg mL−1 aprotinin, and 2 μg mL−1 leupeptin [pH 7.4]). After a 30 min incubation on ice, cells were passed through a needle 10 times. Unbroken cells and nuclei were pelleted by centrifugation at 750g for 10 min.18 Cytochrome c release was assessed by Western blotting as follows.
2.9. Western blotting
Equal amounts of total cellular proteins (50 μg) were resolved by SDS-polyacrylamide gel electrophoresis (SDS-PAGE). Proteins were transferred onto polyvinylidene difluoride (PVDF) membranes (Immobilon P, Millipore, Bedford, MA) with transfer buffer composed of 25 mM Tris-HCl (pH 8.9), 192 mM glycine and 20% methanol. Membranes were then probed with primary antibodies followed by secondary antibodies conjugated to horseradish peroxidase. The immunocomplexes were visualized with an enhanced chemiluminescence kit and used film to develop it (Amersham, UK). The intensity of the band was scanned and quantified by densitometry. The primary antibodies used include anti-caspase-3 and -9 (PharMingen, San Diego, CA), anti-Bcl-2, anti-Bid, anti-Bax (Santa Cruz Biotechnology, Santa Cruz, CA), anti- PARP (UBI Inc., Lake Placid, NY), anti-β-actin and GADD 153 (Transduction Laboratory, Lexington, KY), and anti-DFF45/inhibitor of caspase activated DNase (ICAD) (MBL, Naka-Ku, Nagoya, Japan) antibodies.
2.10. Caspase activity
After garcinol treatment, cells were collected and washed with PBS and suspended in 25 mM HEPES (pH 7.5), 5 mM MgCl2, 5 mM EDTA, 5 mM dithiothione, 2 mM phenylmethanesulfonyl fluoride, 10 μg mL−1 pepstatin A, and 10 μg mL−1 leupeptin. Cell lysates were clarified by centrifugation at 12
000g for 20 min at 4 °C. Caspase activity in the supernatant was determined as described previously.9
2.11. Isolation of RNA and RT-PCR
Total cellular RNA was isolated with a TRIzol Reagent kit (Invitrogen, Life Technologies, Carlsbad, CA) according to the manufacturer's instructions. Changes in the steady-state concentration of GADD153 mRNA were determined as described.18
2.12. Statistical analysis
Data are presented as the mean ± SE Student's t-test was used to determine statistical significance of each experimental test condition compared to respective controls. A P-value of <0.05 was considered statistically significant.
3. Results
3.1. Cytotoxic and apoptotic effects of garcinol in Hep3B, HepG2, A431, MCF-7, and COLO205 cells
To investigate the effect of garcinol on cell growth, we applied various concentrations of garcinol for 24 h to Hep3B, HepG2, A431, MCF-7, and COLO205 cells. Cell viability was determined by the MTT assay. Sub-G1 cells and apoptotic ratios were quantified by flow cytometry. As shown in Fig. 1B and 1C, garcinol exhibited cytotoxic and apoptotic effects on selected human cancer cells in a concentration-dependent manner. Moreover, when treated with 50 μM of garcinol, Hep3B cells exhibited the highest apoptotic ratio among the cell lines studied (Fig. 1C). Therefore, we used Hep3B cells for the subsequent studies to investigate the effects of garcinol on caspases, Bcl-2 family members, and the mechanism of apoptotic cell death.
3.2. Induction of DNA fragmentation in Hep3B cells by garcinol
Apoptosis induces morphological changes including membrane blebbing, cell shrinkage, chromatin condensation, and DNA fragmentation.19 We studied the effect of garcinol on DNA fragmentation by treating Hep3B cells with various concentrations of garcinol for 24 h. DNA ladders emerged at 25 μM of garcinol treatment. The DNA fragmentation response followed a dose-dependent pattern (Fig. 1D). When Hep3B cells were treated with 50 μM of garcinol in time-course experiments, DNA ladders became visible after 3 h of treatment, and the fragmentation pattern continued up to 24 h (Fig. 1E). In addition, garcinol-induced morphological changes in Hep3B cells were observed by light microscopy. As shown in Fig. 2A–E, Hep3B cells exhibited apoptotic bodies after treatment with garcinol in a dose-dependent pattern. To further characterize garcinol-induced cell death, we examined the nuclear morphology of dying cells with acridine orange, a fluorescent DNA-binding agent. Hep3B cells exhibited significant chromosomal condensation, indicative of apoptotic cell death, after 50 μM of garcinol treatment for 24 h (Fig. 2G).
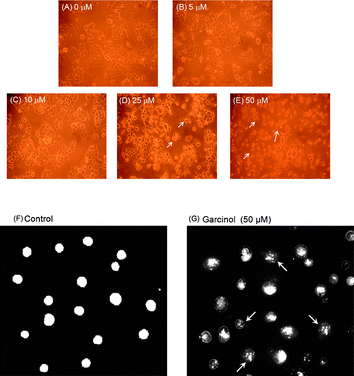 |
| Fig. 2 Induction of chromatin condensation by garcinol in Hep 3B cells. (A–E) Induction of apoptotic bodies by garcinol. Hep3B cells were treated with 0 μM, 5 μM, 10 μM, 25 μM, 50 μM of garcinol for 24 h. The morphological changes of Hep3B cells were observed by light microscopy. 0 μM (vehicle). (F, G) Induction of chromatin condensation by garcinol. Hep3B cells were treated with 0.05% DMSO as vehicle control or 50 μM garcinol for 24 h, followed by acridine orange treatment. The nuclear staining was examined by fluorescence microscopy (200×). Arrowheads indicate nuclear condensation. These experiments were performed at least in triplicate; a representative result is presented. | |
3.3. Induction of reactive oxygen species (ROS) generation, mitochondrial dysfunction, and cytochrome c release in garcinol-induced apoptosis
To verify whether garcinol has the ability to generate ROS, Hep3B cells were treated with 50 μM garcinol for 15 min followed by HE, DCFH-DA, or DiOC6 for an additional 30 min. The generation of ROS, including superoxide anion (O2−) and hydrogen peroxide (H2O2), and the mitochondrial transmembrane potential (ΔΨm) were analyzed by measuring log fluorescence intensity with flow cytometry. Fig. 4 shows that garcinol induced ROS in Hep3B cells. In addition, the mitochondrial membrane potential was reduced in the garcinol-treated cells compared to the control cells (MG
:
40.87 vs. MC
:
133.19) (Fig. 4C). Mitochondria are specialized organelles containing an outer membrane and an inner membrane separated by an inter-membrane space that contains many pro-apoptotic proteins, such as cytochrome c. Fig. 4D illustrates that garcinol treatment gradually increased the release of cytochrome c into the cytosol of Hep3B cells. These observations suggest that garcinol induces apoptosis in Hep3B cells via the mitochondrial pathway. The expression of Bax, Bcl-2 and tBid was evaluated at different time points after garcinol treatment of cells. As shown in Fig. 3A, an apparent increase in Bax, a reduction in Bcl-2 protein expression, and the cleavage of Bid were detected after 1 h treatment with garcinol. The increase in the Bax/Bcl-2 ratio resulted in an enhancement of apoptosis. These data suggest that the cleavage of Bid by active caspase-8 may be one of the mechanisms that contribute to the activation of the mitochondrial pathway during garcinol-induced apoptosis.
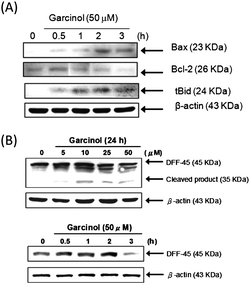 |
| Fig. 3 Effect of garcinol on Bcl-2 protein family and DFF-45 degradation in garcinol-treated Hep3B cells. (A)The expression of Bax, Bcl-2, and tBid were analyzed by Western blotting as described in the Materials and Methods. These experiments were performed at least in triplicate, and a representative experiment is presented. (B) Induction of DFF-45 degradation by garcinol. Hep3B cells were treated with various concentrations of garcinol for 24 h or 50 μM of garcinol at various time points. DFF-45 protein was detected by Western blot analysis using specific antibodies against DFF-45. These experiments were performed at least in triplicate, and a representative experiment is presented. | |
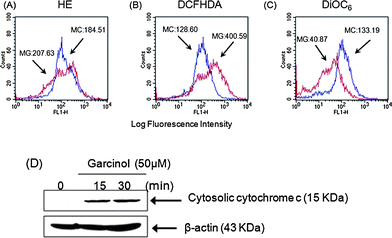 |
| Fig. 4 Induction of reactive oxygen species (ROS) generation, mitochondrial dysfunction, and cytochrome c release by garcinol-induced apoptosis. (A–C) Hep3B cells were treated with 50 μM garcinol for 15 min, incubated with (A) HE, (B) DCFH-DA, or (C) DiOC6 for 30 min, then analyzed by flow cytometry. Data are presented as log fluorescence intensity and expressed as the mean ± SE. “MC” indicates the control group; “MG” represents the garcinol group. (D) Hep3B cells were treated with 50 μM garcinol for the indicated durations. Subcellular fractions were prepared and lysed as described, and cytosolic cytochrome c was detected by Western blots. These experiments were performed at least in triplicate, and a representative experiment is presented. | |
3.4. Induction of caspase activity, PARP cleavage, and DFF-45 degradation in garcinol-induced apoptosis
To observe the effect of garcinol on the caspases activation, the activities of caspases-1,-3,-8, and -9 were measured in Hep3B cells after various durations of treatment with 50 μM of garcinol. As illustrated in Fig. 5A, both caspase-3 and -9 were activated by garcinol in a time-dependent manner, whereas a very low level of caspase-1 activity was detected after the garcinol treatment. Garcinol induced a rapid increase in caspase-3 and caspase-9 activity with approximately 13-fold and 7.8-fold maximal induction, respectively (Fig. 5A). Protein cleavage of pro-caspase-9 and pro-caspase-3 occurred after 0.5 h exposure to garcinol (Fig. 5B).
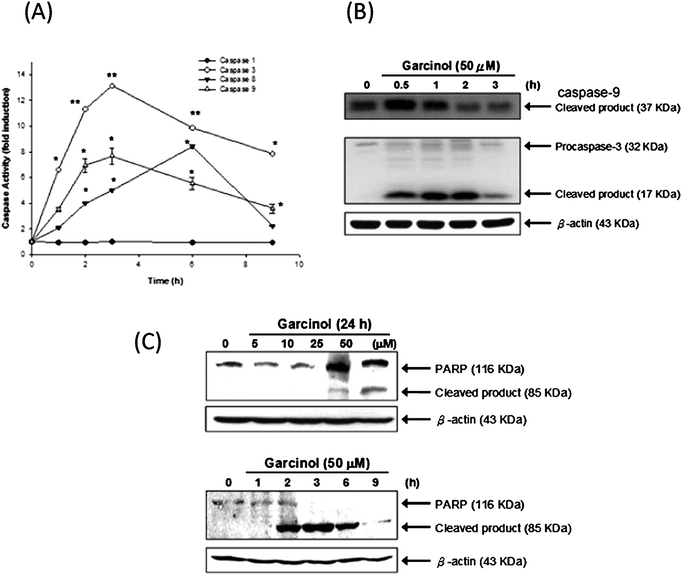 |
| Fig. 5 Induction of caspase activity, PARP cleavage, and DFF-45 degradation by garcinol. (A) Enzymatic activities of caspase-1, -3, -8, and -9 were determined by incubating 50 μg of total protein with corresponding fluorogenic, caspase-specific substrates for 1 h at 30 °C. The release of 7-amino-4-methylcoumarin (AMC) was monitored by fluorescence spectrophotometry (excitation = 360 nm; emission = 460 nm). These experiments were performed at least in triplicate, and a representative experiment is presented. Asterisk denotes a statistically significant increase compared with values of control, *P < 0.01, **P < 0.001. (B) Hep3B cell were treated with garcinol for the indicated durations, and the expression of caspases-9 and -3 were analyzed by Western blot. Hep3B cells were treated with either various concentrations of garcinol for 24 h or 50 μM of garcinol for various durations. PARP expression (C) was detected by Western blot. These experiments were performed at least in triplicate, and a representative result is presented. | |
To detect PARP and DFF-45 cleavage as a result of caspase-3 activation, Hep3B cells were treated either with various concentrations of garcinol for 24 h or with 50 μM for varying durations. As shown in Fig. 5C, garcinol induced dose- and time-dependent proteolytic cleavage of PARP, resulting in a reduction of the 116 kDa protein and accumulation of the 85 kDa fragment. Garcinol also induced cleavage of DFF-45 in a dose-dependent manner. However, cleaved DFF-45 was only detected after 3 h of 50 μM garcinol treatment. (Fig. 3B).
3.5. Induction of GADD153 mRNA and protein expression by garcinol in Hep3B cells
Recently, several studies have suggested that GADD153 triggers critical early events in the initiation of apoptosis.20 Thus, we investigated the effect of garcinol on GADD153 mRNA and protein expression in Hep3B cells. As shown in Fig. 6A, garcinol up-regulated GADD153 mRNA in concentration- (upper panels) and time-dependent manners (lower panels), with peak induction at 1 h following treatment. Similarly, garcinol treatment increased GADD153 protein expression, with the maximal expression also reached after 1 h (Fig. 6B, upper panels). Furthermore, the level of GADD153 protein expression was increased in a dose-dependent manner (Fig. 6B, lower panels). These results indicate that garcinol induced the expression of both GADD153 mRNA and protein, which may play a role in apoptosis.
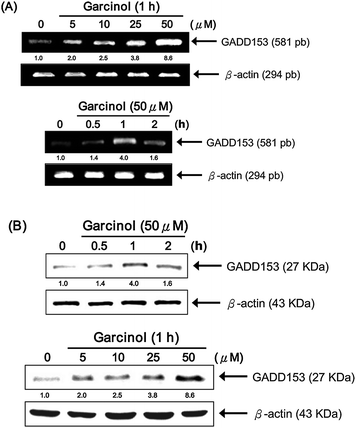 |
| Fig. 6 Garcinol induces up-regulation of GADD153 mRNA and protein expression in a dose-dependent manner. Hep3B cells were incubated with either 5 to 50 μM garcinol for 1 h or 50 μM garcinol for 0 to 2 h. (A) Total RNA was then isolated for multiplex relative RT-PCR analysis using gene-specific primers for the target gene, GADD153, and the internal control gene, β-actin. (B) Whole cell lysates were prepared for Western blot analysis of GADD153 and β-actin. This experiment was repeated three times with similar results. | |
4. Discussion
In this study, we have shown that garcinol treatment decreases cell viability and strongly induces apoptosis via the ROS-dependent pathway in Hep3B cells. Recent studies have suggested numerous apoptosis-inducing chemotherapeutic agents for the treatment of cancer, mutations in p53 block the anti-tumor effects of chemicals in many cases. Interestingly, garcinol, which has been shown to be pharmacologically quite safe and is used in traditional medicine, was found to induce apoptosis in p53-deficient Hep3B cells. Almost half of hepatocellular cancer cases lack functional p53,15b the absence of which blocks the apoptosis-inducing effects of chemotherapeutic agents.21 However, our initial findings indicate a potential clinical application of garcinol for the treatment of hepatocellular cancers.
Garcinol, which contains a phenolic, has a β-diketone and an α,β-unsaturated ketone moiety. Previous studies suggest that α,β-unsaturated carbonyls are susceptible to nucleophilic addition reactions with thiols such as glutathione, the most abundant nonprotein thiol in vivo.22 It is clear that the α,β-unsaturated ketone moiety is essential for exerting cytotoxic activity via depletion of intracellular glutathione.23 Recent studies indicate that intracellular glutathione depletion could result in mitochondrial dysfunction.24 Moreover, oxidative damage to the mitochondrial membrane due to increased generation of ROS has been shown to play an important role in apoptosis. Herein, we demonstrated that garcinol could disrupt mitochondrial function in the early stages of apoptosis and subsequently induce caspase-9, but not caspase-1, activation through the release of cytochrome c. Therefore, we speculate that intracellular generation of ROS could be an important factor in garcinol-induced apoptosis. Recent studies have demonstrated that garcinol can potentiate TRAIL-induced apoptosis through the up-regulation of death receptors and down-regulation of antiapoptotic proteins.25 We also found that garcinol treatment increased caspase-8 activity (approximately 8.3-fold) and levels of tBid in Hep3B cells. These results indicate that garcinol may also induce apoptosis via the death receptor-mediated pathway in Hep3B cells.
Taken together, these data show that garcinol induced the generation of ROS (O2− and H2O2) and the expression of GADD153 in Hep3B cells. The increased ROS and GADD153 further resulted in downstream apoptotic events, including an increase in the Bax/Bcl-2 ratio, a reduction in the mitochondrial membrane potential, the release of cytochrome c into the cytosol, the processing of procaspase-9 and procaspase-3, and the cleavage of PARP and DFF-45. Our findings suggest that the apoptosis-inducing effect of garcinol may provide a means for chemotherapy in hepatocellular cancers.
Acknowledgements
This study was supported by the National Science Council NSC 98-2313-B-022-002-MY3 and NSC 98-2321-B-022-001-
References
-
(a) C. Ito, M. Itoigawa, Y. Miyamoto, S. Onoda, K. S. Rao, T. Mukainaka, H. Tokuda, H. Nishino and H. Furukawa, J. Nat. Prod., 2003, 66, 206 CrossRef CAS;
(b) M. Iinuma, H. Tosa, T. Tanaka, S. Kanamaru, F. Asai, Y. Kobayashi, K. Miyauchi and R. Shimano, Biol Pharm Bull, 1996, 19, 311 CAS.
- S. Padhye, A. Ahmad, N. Oswal and F. H. Sarkar, J. Hematol. Oncol., 2009, 2, 38 Search PubMed.
-
(a) C. H. Liao, C. T. Ho and J. K. Lin, Biochem. Biophys. Res. Commun., 2005, 329, 1306 CrossRef CAS;
(b) J. Kolodziejczyk, M. Masullo, B. Olas, S. Piacente and B. Wachowicz, Platelets, 2009, 20, 487 CrossRef CAS.
- F. Yamaguchi, M. Saito, T. Ariga, Y. Yoshimura and H. Nakazawa, J. Agric. Food Chem., 2000, 48, 2320 CrossRef CAS.
- A. Chatterjee, T. Yasmin, D. Bagchi and S. J. Stohs, Mol. Cell. Biochem., 2003, 243, 29 CrossRef CAS.
- C. H. Liao, S. Sang, Y. C. Liang, C. T. Ho and J. K. Lin, Mol. Carcinog., 2004, 41, 140 CrossRef CAS.
- T. Tanaka, H. Kohno, R. Shimada, S. Kagami, F. Yamaguchi, S. Kataoka, T. Ariga, A. Murakami, K. Koshimizu and H. Ohigashi, Carcinogenesis, 2000, 21, 1183 CrossRef CAS.
- K. Yoshida, T. Tanaka, Y. Hirose, F. Yamaguchi, H. Kohno, M. Toida, A. Hara, S. Sugie, T. Shibata and H. Mori, Cancer Lett., 2005, 221, 29 CrossRef CAS.
- M. H. Pan, W. L. Chang, S. Y. Lin-Shiau, C. T. Ho and J. K. Lin, J. Agric. Food Chem., 2001, 49, 1464 CrossRef CAS.
- C. S. Chen, C. H. Lee, C. D. Hsieh, C. T. Ho, M. H. Pan, C. S. Huang, S. H. Tu, Y. J. Wang, L. C. Chen, Y. J. Chang, P. L. Wei, Y. Y. Yang, C. H. Wu and Y. S. Ho, Breast Cancer Res. Treat. DOI:10.1007/s10549–010–0821–3.
- A. Ahmad, Z. Wang, R. Ali, M. Y. Maitah, D. Kong, S. Banerjee, S. Padhye and F. H. Sarkar, J. Cell Biochem., 2010, 109, 1134 CAS.
- S. Prasad, J. Ravindran, B. Sung, M. K. Pandey and B. B. Aggarwal, Mol. Cancer Ther., 2010, 9, 856 CrossRef CAS.
- C. H. Liao, S. Sang, C. T. Ho and J. K. Lin, J. Cell. Biochem., 2005, 96, 155 CrossRef CAS.
- K. Balasubramanyam, M. Altaf, R. A. Varier, V. Swaminathan, A. Ravindran, P. P. Sadhale and T. K. Kundu, J. Biol. Chem., 2004, 279, 33716 CrossRef CAS.
-
(a) P. A. Wingo, T. Tong and S. Bolden, Ca–Cancer J. Clin., 1995, 45, 8 Search PubMed;
(b) E. Tabor, J. Med. Virol., 1994, 42, 357 CrossRef CAS.
- B. Bressac, K. M. Galvin, T. J. Liang, K. J. Isselbacher, J. R. Wands and M. Ozturk, Proc. Natl. Acad. Sci. U. S. A., 1990, 87, 1973 CrossRef CAS.
- B. Ringe, R. Pichlmayr, C. Wittekind and G. Tusch, World J. Surg., 1991, 15, 270 CrossRef CAS.
- M. H. Pan, Y. S. Lai, C. S. Lai, Y. J. Wang, S. Li, C. Y. Lo, S. Dushenkov and C. T. Ho, J. Agric. Food Chem., 2007, 55, 5081 CrossRef CAS.
- S. G. Cho and E. J. Choi, J. Biochem. Mol. Biol., 2002, 35, 24 Search PubMed.
-
(a) S. Ikeyama, X. T. Wang, J. Li, A. Podlutsky, J. L. Martindale, G. Kokkonen, R. van Huizen, M. Gorospe and N. J. Holbrook, J. Biol. Chem., 2003, 278, 16726 CrossRef CAS;
(b) D. G. Kim, K. R. You, M. J. Liu, Y. K. Choi and Y. S. Won, J. Biol. Chem., 2002, 277, 38930 CrossRef CAS.
- S. W. Lowe, Curr. Opin. Oncol., 1995, 7, 547 CrossRef CAS.
- E. Boyland and L. F. Chasseaud, Biochem J, 1968, 109, 651 CAS.
- J. Atsmon, M. L. Freeman, M. J. Meredith, B. J. Sweetman and L. J. Roberts, 2nd, Cancer Res, 1990, 50, 1879 CAS.
- C. Friesen, Y. Kiess and K. M. Debatin, Cell Death Differ., 2004, 11(Suppl 1), S73 CrossRef CAS.
- S. Prasad, J. Ravindran, B. Sung, M. K. Pandey and B. B. Aggarwal, Mol. Cancer Ther., 2010, 9, 856 CrossRef CAS.
|
This journal is © The Royal Society of Chemistry 2010 |