Sedimentary pools of phosphorus in the eutrophic Tamar estuary (SW England)†
Received
10th June 2009
, Accepted 14th August 2009
First published on
17th September 2009
Abstract
The speciation of phosphorus in Tamar estuary sediment has been determined at eight stations from Gunnislake (riverine end-member) to Torpoint (marine end-member) using the SEDEX (sedimentary extraction) sequential extraction scheme. The seasonal variability of total phosphorus and each of the five fractions is discussed. Total phosphorus, in the range 26–51 µmol g−1, was stored in the relatively labile fractions, particularly in the iron oxide fraction. The well oxygenated waters of the macrotidal Tamar estuary ensure that the surface sediment layer remains oxic, providing an effective barrier that retains dissolved reactive phosphorus (DRP) within the sedimentary iron oxide fraction and prevents phosphorus migration from the sediment to the water column. The exchangeable and organic phosphorus sedimentary pools, although small, are therefore the main potential sources of phosphorus release to the water column. In particular, this study shows that exchangeable phosphorus and dissolved organic phosphorus (DOP) in the pore water are intimately linked. Finally, the distribution of phosphorus in the estuary is strongly influenced by particle morphology, with most of the sedimentary phosphorus forms exhibiting significant positive correlations with the percent of each grain size.
Environmental impact
Phosphorus (P) plays a critical role in eutrophication of water bodies and high P concentrations stimulate algal blooms. Estuaries are particularly sensitive to eutrophication because they receive the riverine nutrient load, with the major P fraction associated with the sediment. This P pool is subject to biogeochemical transformations and is intimately linked with the water column P concentration. Knowledge of sedimentary P pools is therefore essential for understanding estuarine P dynamics. This study shows P is mainly bound to iron oxides/oxyhydroxides but the oxic surface layer prevents migration to the water column. The exchangeable and organic P pools are therefore more involved in P cycling despite being quantitatively less significant. These findings are broadly applicable to temperate, macrotidal, eutrophic estuaries.
|
1. Introduction
Phosphorus (P) is known to play a critical role in the eutrophication of water bodies1 and high concentrations of P can stimulate algal blooms and sustain excessive nuisance algal growth. Estuaries are particularly sensitive to eutrophication because they receive most of the riverine nutrient load, which has greatly increased in recent years due to increased anthropogenic activity.2 Despite the most bioavailable source of P being in the dissolved form, the major P fraction in rivers and estuaries is generally associated with the sediment phase.3 Sediments contain both inorganic and organic forms of P that, in shallow environments such as estuaries, are subject to biogeochemical transformations, with significant reorganization of P occurring during burial.4 Thus, refractory P compounds are inert and simply buried in their original forms, while more labile P material may decompose and/or dissolve to release bioavailable forms of P in the pore water.5 Depending on the prevailing chemical conditions, the regenerated P may be adsorbed to grain surfaces, co-precipitated with iron oxyhydroxide, eventually transformed in situ to a mineralized form within the sediments, such as authigenic material (e.g. carbonate fluorapatite—CFA), or ultimately released to the overlying water.6 Consequently, the sedimentary forms of P, in association with the environmental conditions, regulate storage, recycling and P release, and are therefore intimately linked with the concentration of dissolved P in the water column.7 Knowledge of the various solid P pools is therefore essential for understanding P dynamics in estuarine environments.
The Tamar estuary is located in south west England and is characterized by substantial nutrient inputs from agricultural runoff and urban wastewater discharges. The distribution and benthic fluxes of inorganic phosphate have already been investigated, indicating short term variability, non-biological removal within the low salinity range8 and transport out of the sediment throughout the year.9 However, the characterization and the quantification of the different sedimentary reservoirs of P have surprisingly received no attention. In this context, the sequential extraction procedure SEDEX (sedimentary extraction), developed by Ruttenberg,10 has been applied to the sediments of the Tamar estuary at four different times of the year (winter, spring, summer and autumn). Due to the importance of sorption processes during fresh and marine water mixing, and the phosphate buffer mechanism,11 special attention has also been given to determining readily exchangeable P. Thus, a series of complementary experiments based on the infinite dilution extrapolation (IDE) approach developed by Aminot and Andrieux12 have been carried out at different salinities and locations in the estuary. The overall objective of this study was to gain an insight into the role of sediments in P cycling in a eutrophic estuarine system. This was specifically addressed by: (1) assessing the distribution of the different reservoirs of P along the salinity gradient at different times of the year, (2) identifying potential releases of exchangeable P under various salinity conditions, (3) examining the factors influencing the distribution of phosphorus forms in coastal and estuarine sediments, with particular attention to particle size, and (4) determining the seasonality of sedimentary P export to the water column.
2. Experimental
2.1. Main characteristics of the study area
The Tamar estuary, located within 50°19′N–50°32′N and 4°06′W–4°17′W, forms the main channel of a large ria system on the south west coast of England (Fig. 1). It is 31 km long from its boundary with Plymouth Sound to its limit of tidal influence at Weir Head, including two tributary rivers (Tavy and Lynher rivers). The whole estuarine catchment area is about 1500 km2, of which the river Tamar catchment is 924 km2. This latter is mainly a region of intensive arable and dairy farming. The concentration of phosphorus (as P2O5) in surface soils ranges from 0.1–1% (m/m) (mean 0.3%). Moreover, around one-third of the catchment soils have ‘excessive’ available P content (∼27 mg P kg−1 determined as Olsen P) for sites under grassland, which accounts for approximately two-thirds of land use across the catchment.13 Tides are semidiurnal and asymmetrical, with mean neap and spring ranges of 2.2 and 4.7 m, respectively. The water depth in the main channel varies between 2 and 8 m below mean spring high-water in the inner estuary and between 8 and 40 m in the outer estuary. A high turbidity maximum (HTM) is consistently associated with the lower salinity reaches of the estuary.14 This turbidity maximum is due to a large, mobile stock of bed source fine sediment and is usually located between 6 and 15 km from the Weir (during normal conditions). It can move up and down the estuary depending on the intensity of the tide and the Tamar river flow. The long-term minimum, mean and maximum freshwater inflows within the estuary are 3, 34 and 290 m3 s−1, respectively. The estuary also exhibits large areas of intertidal mud flats, with the silt and clay content of the bed sediment decreasing from 90–95% in the HTM region to 60–70% downstream. Finally, Jackson et al.15 reported that during summer months, very large populations of freshwater algae (dominated by diatoms) were often observed in the upper reaches of the estuary. Nanoplankton and ultraplankton have also been reported as the most abundant plankton populations in the estuary.16
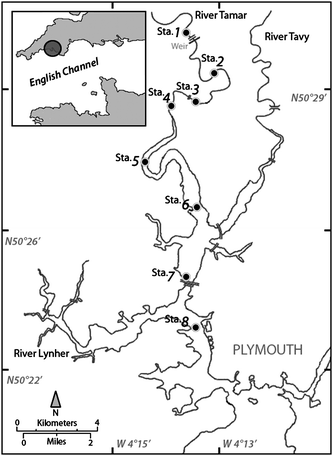 |
| Fig. 1 Map of the Tamar estuary showing the sampling locations for the eight stations. | |
Sample collection and survey programme.
Surface sediment (0–1 cm) grab samples were collected during 4 sampling campaigns in 2007. All samples (one per station) were collected at eight fixed stations along the estuary (Fig. 1), starting upstream immediately after low water, and always at a tidal amplitude <5.2 m. The sediment pore water was removed from the sediment by centrifugation (15 min, 4500 rpm) and the sediment was thereafter frozen at −20 °C, freeze-dried and ground to <125 µm. All the pore water samples were filtered through a 0.2 µm hydrophilic polyethersulfone membrane and then stored frozen at −20 °C until analyzed.
Procedures and analytical methods.
Sediment total phosphorus (TP) and metal concentrations were determined by inductively coupled plasma-optical emission spectroscopy (Perkin Elmer 5300DV) after aqua regia digestion in a sealed microwave digestion system. Detailed P sediment speciation was obtained using the SEDEX sequential extraction procedure reported by Ruttenberg10 with two modifications. Firstly, Fe-bound P was still determined as citrate–dithionite–bicarbonate (CDB), but with a decreased concentration of sodium dithionite to 0.033 M as recommended by Anderson and Delaney.17 Secondly, organic P was determined as the difference between total P and the sum of the first four P pools. All of the original SEDEX wash steps were still included. The five sedimentary P pools separated by the SEDEX scheme are: (1) exchangeable and loosely sorbed P, (2) P bound to ferric oxyhydroxides, (3) authigenic P (including carbonate fluoroapatite—CFA, biogenic Ca–P compounds, CaCO3-bound P and possibly smectite-bound P), (4) detrital apatite of igneous or metamorphic origin and (5) organic P. The precision of individual extractions was generally <7% RSD (n = 3). However, comparisons of organic P determined (a) by difference between TP and the sum of inorganic P determined with the sequential procedure and (b) non-sequentially (organic P determined by the difference in P content of the 1 M hydrochloric acid extract measured before and after ignition of the dry sediments at 550 °C)18 indicated larger uncertainty in the organic P concentration (∼15% RSD, n = 3). Metals such as Al, Fe, Mn and Ca were also determined by inductively coupled plasma-optical emission spectrometry (ICP-OES, Perkin Elmer 5300DV) after aqua regia digestion. Recovery percentages were, respectively, 76, 97, 95 and 103. Analytical precision was 15% RSD (n = 3) for these elements. Finally, particle sizing and specific area on sediment samples were made using a long-bed Malvern Mastersizer X with wet sample unit MS17. The organic matter in the sediment sample was previously removed using a wet oxidation procedure (6% m/v hydrogen peroxide at high temperature).
Dissolved reactive phosphorus (DRP) concentrations in extracts were determined using an automated spectrophotometric flow injection analysis (FIA) system equipped with a multi-reflection flow cell.19 Prior to analysis, all extracts were diluted (1/10) in ultrapure water. DRP was analyzed using the molybdenum-blue method with tin(II) chloride as the reductant and detected at 690 nm. Validation of the method is described in detail by Benson et al.20 All DRP analyses were carried out with the addition of sodium dodecyl sulfate (SDS, 0.3% m/v) to stabilize the phosphomolybdenum blue complex in solution and prevent precipitation in the detection cell. The detection limit (defined as the concentration equivalent to the blank signal + 3σn−1 of the blank) was 0.006 µM (0.2 µg L−1). The relative standard deviation of triplicate injections was always <3% for standard and samples.
Exchangeable phosphate by the infinite dilution extrapolation (IDE) method.
By definition, exchangeable phosphate is the amount of phosphate that could be released from a solid into an oxic water body of infinite volume, totally depleted of phosphate. On this basis, the exchangeable phosphate was determined for surface sediment using the infinite dilution extrapolation (IDE) method developed by Aminot and Andrieux.12 Briefly, different masses of sediment were added to natural water depleted of phosphate (low nutrient seawater, OSIL, Havant, UK). The different salinities (0, 10, and 20) were obtained by dilution of this low nutrient seawater with ultrapure water. The sediment concentrations in solution ranged from 150–2500 mg L−1. After 48 h of contact (on a shaking table), the solutions were centrifuged and analyzed for DRP. Released phosphate (in µmol g−1) was plotted against the inverse of the solid (sediment) concentration (in L g−1). Exchangeable phosphate was then obtained from the asymptote of the plot of 1/Y = m + n/X where Y is the amount of solute sorbed per unit mass of solid at equilibrium minus the initial amount of phosphate sorbed per unit mass of solid in the batch experiment (i.e. exchangeable phosphate) and X = 1/S where S is the concentration of sediment in solution. An example of an IDE method application is shown in Fig. 2.
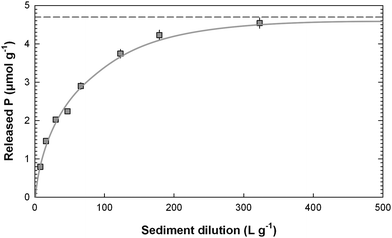 |
| Fig. 2 Example of an IDE (infinite dilution extrapolation) extraction for the determination of exchangeable P (PIDE)—Morwellham (Station 2) in spring, salinity 20. | |
3. Results and discussion
3.1. Tamar estuary surface sediment characteristics
The characteristics of surface sediments along a transect of the Tamar estuary are presented in Table 1. The sediments were composed mainly of <22 µm and 22–63 µm grain size fractions. These fractions represented 66–80% of the sediment over the seasonal cycle, but no marked seasonal variations in grain size were observed. The finest fraction (<22 µm) was mainly located between Stations 2 and 4. Only sediment from Station 1 contained predominantly coarser particles (>125 µm). This resulted from the mixing of natural fine sediment with coarse sand artificially brought into this area to consolidate the riverbanks. The chemical composition of the surface sediment is given in Table 1. The sediments were usually rich in iron and to a lesser extent in aluminium. The concentrations of calcium and manganese were much lower than iron. In general, iron, aluminium and manganese concentrations tended to decrease downstream in the estuary, while calcium was noticeably higher at both estuary end members. Overall, no clear seasonal patterns for these major elements were observed over the sampling period.
Table 1 Grain size characteristics and major element concentrations in surface sediment at eight stations in the Tamar estuary (SW England) in winter, spring, summer, and autumn 2007
Season |
Station |
Grain size (%) |
Major element/mg kg−1 |
<22 µm |
22–63 µm |
63–125 µm |
125–500 µm |
>500 µm |
Fe |
Al |
Ca |
Mn |
Winter |
1 |
9.9 |
5.7 |
6.8 |
61 |
16 |
52 100 |
14 100 |
12 000 |
1630 |
2 |
67 |
25 |
4.0 |
2.9 |
0.5 |
43 000 |
14 100 |
5120 |
903 |
3 |
74 |
20 |
3.5 |
2.1 |
0.6 |
41 700 |
14 000 |
3290 |
749 |
4 |
63 |
27 |
6.8 |
3.0 |
0.5 |
40 800 |
13 900 |
3920 |
837 |
5 |
42 |
32 |
20 |
5.3 |
0.4 |
32 000 |
11 100 |
4700 |
610 |
6 |
48 |
38 |
13 |
1.9 |
0.2 |
34 400 |
11 800 |
7530 |
625 |
7 |
58 |
31 |
8.7 |
2.0 |
0.3 |
34 700 |
11 700 |
7510 |
523 |
8 |
21 |
29 |
29 |
20.9 |
0.7 |
22 600 |
8180 |
19 300 |
304 |
Spring |
1 |
31 |
13 |
3.8 |
45 |
7.5 |
45 800 |
16 000 |
12 300 |
1440 |
2 |
72 |
23 |
3.6 |
1.1 |
0.0 |
40 700 |
16 800 |
4740 |
810 |
3 |
54 |
17 |
3.8 |
9.5 |
16 |
37 600 |
16 000 |
7220 |
845 |
4 |
64 |
26 |
8.5 |
2.1 |
0.0 |
36 200 |
14 800 |
4180 |
670 |
5 |
47 |
34 |
15 |
3.7 |
0.0 |
34 400 |
14 900 |
4850 |
617 |
6 |
59 |
33 |
6.4 |
1.4 |
0.1 |
34 200 |
14 000 |
4790 |
472 |
7 |
58 |
28 |
10 |
3.0 |
0.0 |
38 000 |
15 000 |
4010 |
782 |
8 |
42 |
32 |
19 |
6.7 |
0.1 |
27 800 |
12 100 |
23 800 |
448 |
Summer |
1 |
34 |
13 |
12 |
37 |
3.6 |
39 500 |
17 100 |
9700 |
1440 |
2 |
65 |
27 |
5.3 |
2.1 |
0.1 |
42 000 |
16 400 |
4690 |
1130 |
3 |
60 |
30 |
7.2 |
1.7 |
1.1 |
41 800 |
16 000 |
4240 |
1360 |
4 |
56 |
31 |
9.8 |
2.2 |
0.1 |
39 900 |
14 700 |
3660 |
1200 |
5 |
54 |
33 |
11 |
1.6 |
0.4 |
35 100 |
15 500 |
3640 |
697 |
6 |
58 |
34 |
6.2 |
0.9 |
0.9 |
35 500 |
14 600 |
4720 |
532 |
7 |
31 |
10 |
5.9 |
20 |
32 |
48 700 |
18 500 |
10 900 |
755 |
8 |
26 |
26 |
28 |
19 |
0.4 |
26 900 |
10 000 |
24 600 |
541 |
Autumn |
1 |
4.1 |
2.3 |
2.7 |
0.0 |
91 |
46 600 |
12 200 |
12 200 |
1030 |
2 |
63 |
28 |
6.2 |
0.0 |
2.3 |
40 300 |
12 600 |
5000 |
1120 |
3 |
68 |
26 |
4.4 |
0.0 |
2.4 |
42 500 |
13 900 |
3900 |
831 |
4 |
63 |
26 |
8.0 |
0.0 |
3.4 |
37 100 |
12 100 |
3630 |
696 |
5 |
38 |
30 |
22 |
0.0 |
9.6 |
32 900 |
11 200 |
5000 |
648 |
6 |
43 |
38 |
14.0 |
0.0 |
5.5 |
31 000 |
10 800 |
5230 |
425 |
7 |
32 |
14 |
6.3 |
0.0 |
47 |
40 700 |
13 900 |
6550 |
905 |
8 |
22 |
30 |
28 |
0.0 |
20 |
27 400 |
10 600 |
20 300 |
380 |
3.2. Total phosphorus (TP) in surface Tamar estuary sediment
Surface sediment TP and sequentially extracted sedimentary P fractions are summarized in Table 2 and presented in Fig. 3. The concentrations of TP ranged from 26 to 51 µmol g−1 and on average (40 µmol g−1) remained constant throughout the seasonal cycle. The highest TP concentrations were generally observed in the upper part of the estuary between Stations 2 and 4 while the estuarine end members were always characterized by the lowest TP concentrations. The observed TP concentrations are relatively high compared with other temperate estuaries but are in agreement with previously reported data for the Tamar estuary.21,22
Table 2 Concentrations of total phosphorus and the different forms of phosphorus from a five-step sequential extraction (in µmol g−1) in surface sediments at eight stations in the Tamar estuary (SW England) in winter, spring, summer, and autumn 2007
Season |
Station |
SEDEX extracted P forms |
Total P |
Pex |
PFe |
Paut |
Pdet |
Porg |
TP |
µmol g−1 |
(%) |
µmol g−1 |
(%) |
µmol g−1 |
(%) |
µmol g−1 |
(%) |
µmol g−1 |
(%) |
µmol g−1 |
Winter |
1 |
0.3 |
(0.7) |
24 |
(62) |
1.9 |
(5.0) |
9.4 |
(24) |
3.0 |
(7.7) |
39 |
2 |
1.0 |
(2.0) |
32 |
(65) |
2.4 |
(4.8) |
3.5 |
(7.0) |
11 |
(21) |
50 |
3 |
0.9 |
(1.9) |
35 |
(72) |
2.5 |
(5.2) |
3.2 |
(6.6) |
6.7 |
(14) |
48 |
4 |
1.1 |
(2.2) |
32 |
(68) |
2.6 |
(5.5) |
3.5 |
(7.5) |
7.8 |
(17) |
47 |
5 |
1.0 |
(2.8) |
24 |
(63) |
1.9 |
(5.0) |
5.0 |
(13) |
5.7 |
(15) |
37 |
6 |
1.4 |
(3.6) |
25 |
(62) |
2.3 |
(5.8) |
4.3 |
(11) |
7.4 |
(18) |
40 |
7 |
2.1 |
(5.1) |
24 |
(59) |
2.6 |
(6.5) |
4.2 |
(10) |
7.7 |
(19) |
41 |
8 |
1.6 |
(6.1) |
11 |
(41) |
2.8 |
(11) |
6.6 |
(26) |
4.4 |
(17) |
26 |
Spring |
1 |
0.7 |
(2.4) |
15 |
(55) |
2.0 |
(7.3) |
7.4 |
(27) |
2.4 |
(8.6) |
28 |
2 |
1.2 |
(2.7) |
29 |
(65) |
4.2 |
(9.3) |
3.3 |
(7.2) |
7.3 |
(16) |
45 |
3 |
2.2 |
(5.9) |
19 |
(52) |
4.6 |
(12) |
3.4 |
(9.1) |
7.7 |
(21) |
37 |
4 |
1.7 |
(3.9) |
25 |
(60) |
3.6 |
(8.5) |
3.6 |
(8.5) |
8.0 |
(19) |
42 |
5 |
1.5 |
(3.7) |
25 |
(61) |
3.6 |
(8.9) |
4.2 |
(11) |
6.4 |
(16) |
40 |
6 |
2.1 |
(5.3) |
23 |
(60) |
3.8 |
(10) |
3.4 |
(8.9) |
6.0 |
(16) |
39 |
7 |
1.5 |
(3.1) |
33 |
(69) |
3.5 |
(7.3) |
3.3 |
(6.7) |
7.0 |
(14) |
49 |
8 |
1.5 |
(5.9) |
9.4 |
(36) |
4.3 |
(16) |
5.1 |
(20) |
5.7 |
(22) |
26 |
Summer |
1 |
0.4 |
(1.3) |
18 |
(59) |
2.7 |
(8.5) |
8.8 |
(28) |
1.1 |
(3.4) |
31 |
2 |
1.0 |
(2.0) |
30 |
(61) |
3.5 |
(7.3) |
3.3 |
(6.7) |
11 |
(23) |
49 |
3 |
0.8 |
(1.6) |
32 |
(65) |
3.2 |
(6.4) |
2.7 |
(5.5) |
11 |
(21) |
50 |
4 |
1.0 |
(2.1) |
33 |
(69) |
3.2 |
(6.7) |
3.0 |
(6.2) |
7.8 |
(16) |
48 |
5 |
1.2 |
(2.9) |
28 |
(67) |
3.6 |
(8.7) |
4.3 |
(10) |
4.8 |
(12) |
41 |
6 |
2.0 |
(5.0) |
22 |
(56) |
3.9 |
(9.9) |
3.6 |
(9.0) |
8.1 |
(20) |
40 |
7 |
1.8 |
(5.3) |
17 |
(51) |
6.6 |
(20) |
6.4 |
(19) |
1.6 |
(4.8) |
33 |
8 |
2.0 |
(7.2) |
8.2 |
(29) |
8.4 |
(30) |
6.5 |
(23) |
3.0 |
(11) |
28 |
Autumn |
1 |
0.2 |
(0.5) |
18 |
(56) |
1.2 |
(3.8) |
9.5 |
(30) |
3.1 |
(9.7) |
32 |
2 |
1.1 |
(2.1) |
34 |
(68) |
2.2 |
(4.3) |
3.2 |
(6.4) |
9.9 |
(19) |
51 |
3 |
1.2 |
(2.5) |
33 |
(66) |
2.5 |
(5.0) |
3.4 |
(6.9) |
9.9 |
(20) |
50 |
4 |
1.0 |
(2.4) |
33 |
(75) |
2.3 |
(5.3) |
4.0 |
(9.0) |
3.6 |
(8.2) |
44 |
5 |
1.1 |
(2.4) |
31 |
(71) |
1.9 |
(4.4) |
4.8 |
(11) |
5.1 |
(12) |
44 |
6 |
1.2 |
(3.6) |
21 |
(61) |
2.4 |
(6.9) |
4.9 |
(14) |
4.6 |
(14) |
34 |
7 |
1.5 |
(3.6) |
21 |
(50) |
4.5 |
(10) |
1.3 |
(3.1) |
14 |
(32) |
43 |
8 |
1.2 |
(4.1) |
9.8 |
(34) |
3.1 |
(11) |
7.3 |
(25) |
7.6 |
(26) |
29 |
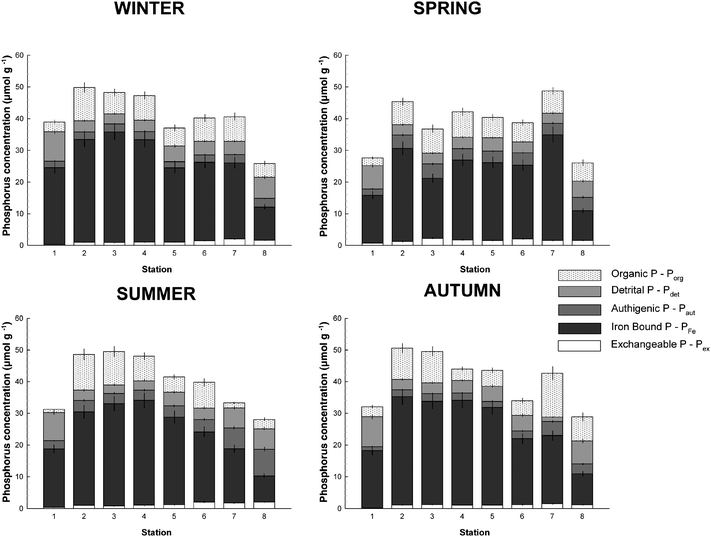 |
| Fig. 3 Seasonal distribution patterns of different phosphorus fractions (i.e. total P—TP; exchangeable P—Pex; iron-bound P—PFe; authigenic P—Paut; detrital P—Pdet; organic P—Porg) in surface sediment along a transect of the Tamar estuary. | |
3.3. Solid-phase P speciation in surface Tamar estuary sediment
Exchangeable or loosely sorbed P (Pex).
The exchangeable and loosely sorbed P determined via the SEDEX procedure represented on average 3% of TP with concentrations generally below ∼2 µmol g−1 throughout the year (Table 2 and Fig. 3). Pex concentrations were always lowest in the freshwater upper stations of the estuary and generally increased slightly towards the marine stations. A low Pex fraction (usually <8 to 10%) is a common characteristic of coastal ecosystem sediments, as observed previously.4,23–27 However, phosphate exchange between sediment particles and the water column has been recognized as a quantitatively important process in rivers and estuaries.28 Because the SEDEX sequential extraction scheme has been developed for marine sediment, the concentration of MgCl2 mimics the chloride concentration in seawater. Thus Pex results from the desorption of exchangeable and loosely sorbed P due to the formation of the Mg–PO4− complex and/or mass action displacement by chloride ions. In the Tamar estuary, the same sediments can be exposed to either fresh or fully marine waters, depending on the state of the tide and/or river flow. Therefore the reported values of Pex are indicative of, but not necessarily directly related to, the potential quantities of P exchanged with estuarine waters.
The IDE approach developed by Aminot and Andrieux12 has therefore been applied to the estuarine sediment for each season at four different salinities that covered the complete estuarine salinity gradient (0, 10, 20 and 35) and the results are presented in Table 3. The concentrations of PIDE ranged from 2.9 to 5.7 µmol g−1 for the upper estuarine sediment (Station 2—Morwellham), from 2.7 to 6.6 µmol g−1 for the middle estuarine sediment (Station 6—Cargreen) and from 1.1 to 4.5 µmol g−1 for the lower estuarine sediment (Station 8—Torpoint). For these three stations, concentrations of exchangeable P extracted by the IDE protocol were on average 4.4, 2.6 and 1.5 times higher than those obtained by SEDEX (Pex). A previous study4 highlighted the difference between PIDE and Pex determinations for surface sediment, which showed PIDE values up to 2.7 times higher than Pex values due to the empirical and system-specific nature of the determination. Therefore comparison between PIDE and Pex concentrations must be treated with caution. Nevertheless, the results suggest that the quantity of exchangeable P might represent up to 12% of the P stored in the surface sediment. The determination of PIDE for each station and season at different salinities exhibited relative standard deviations ranging from 15 to 32%. In general, PIDE tended to decrease with increasing salinity, except during spring when it exhibited the opposite trend. Finally, PIDE concentrations were in general also highest in winter and lowest in summer, clearly highlighting two seasonal extremes. It is therefore likely that during summer conditions, some exchangeable P is released into the water column and contributes to the higher P concentrations usually observed in the estuary at this time of the year.29 Similarly, in winter, surface sediment might be re-loaded with exchangeable P, either directly from the water column and/or via mineralization of fresh, labile organic P transported to the sediment throughout the year (mainly in spring and summer).
Table 3 Seasonal variability of exchangeable phosphorus (in µmol g−1) in Tamar estuary surface sediment at three different stations (Station 2—Morwellham, Station 5—Cargreen and Station 8—Torpoint) and four different salinities (0, 10, 20 and 35)
Station |
Season |
Salinity |
Winter |
Spring |
Summer |
Autumn |
Morwellham |
0 |
5.5 |
2.9 |
5.5 |
5.5 |
10 |
5.6 |
4.7 |
3.6 |
3.8 |
20 |
5.7 |
4.7 |
3.5 |
5.2 |
35 |
4.0 |
5.4 |
3.4 |
4.9 |
Cargreen |
0 |
6.6 |
2.7 |
5.8 |
4.1 |
10 |
3.4 |
4.5 |
4.8 |
2.7 |
20 |
3.7 |
4.8 |
3.5 |
3.8 |
35 |
4.6 |
5.0 |
3.6 |
3.6 |
Torpoint |
0 |
4.5 |
1.2 |
2.3 |
2.9 |
10 |
2.9 |
1.7 |
2.0 |
1.9 |
20 |
2.4 |
1.6 |
1.1 |
2.2 |
35 |
2.8 |
2.5 |
1.5 |
2.1 |
Iron oxide/oxyhydroxide-bound P (PFe).
The PFe concentrations ranged from 8.2 to 34 µmol g−1, representing 29–75% of the TP pool, and is the dominant P fraction in Tamar estuary sediment. The upper and lower reaches of the estuary were always characterized by lower PFe concentrations for all seasons which are probably related to low Fe concentrations in the sediment. Grain size distribution may well affect PFe adsorption capacity in the upper estuary (see discussion in Section 3.4). No seasonal variations were observed but overall a positive correlation between PFe and Fe concentrations was observed (see Table 4). Despite the statistical significance of this relationship, the relatively low correlation coefficient (0.44) probably resulted from some Fe in the sediment being non-reactive.30 Iron oxides such as poorly crystalline ferrihydrite and akaganeite are known to play an important role in binding P to sediment.6 Sedimentary particulate Fe usually occurs not only as Fe oxides but also as siderite (FeCO3), pyrite (FeS2), iron monosulfides (FeS) or vivianite (Fe3(PO4)2·8H2O) and hence the Fe content in the bulk sediment (as measured in this study) does not necessarily reflect the quantity of P-reactive Fe.
Table 4 Correlation coefficient matrix of grain sizes and major element concentrations with the different forms of phosphorus in the Tamar estuary (data for the whole year). Only r values significant at the 95% confidence level are shown. PINOR represents the sum of all the inorganic fractions of P
|
Pex |
PFe |
Paut |
Pdet |
Porg |
TP |
PINOR |
<22 µm (%) |
|
0.72 |
|
–0.82 |
0.54 |
0.75 |
0.67 |
22–63 µm (%) |
0.45 |
|
|
–0.52 |
|
|
|
63–125 µm (%) |
|
–0.55 |
|
|
|
–0.54 |
–0.54 |
125–500 µm (%) |
|
–0.38 |
|
0.69 |
–0.58 |
–0.45 |
|
>500/µm (%) |
|
|
|
0.40 |
|
|
|
Fe/mg kg−1 |
–0.51 |
0.44 |
|
|
|
0.42 |
0.50 |
Mn/mg kg−1 |
–0.68 |
|
|
|
|
|
|
Al/mg kg−1 |
|
|
|
|
|
|
0.37 |
Ca/mg kg−1 |
|
–0.87 |
0.37 |
0.61 |
–0.40 |
–0.81 |
–0.82 |
The fact that sedimentary P speciation is largely dominated by PFe has important implications for P cycling and bioavailability in the overlying waters of the estuary. Indeed, the reduction of Fe oxides in the anoxic sediment zone usually results in substantial release of P (mainly as DRP) into the pore water, which could ultimately reach the overlying water column, and thus possibly sustain further primary production and algal blooms. Provided that the surface sediment layer stays relatively well oxygenated, the redox state of this sediment layer should not be affected and therefore preserve an effective barrier to prevent any DRP migration from the sediment to the water column. Specifically, during eutrophication events, involving nuisance or harmful algal blooms and bottom water hypoxia, release of P into the water column of the Tamar estuary would clearly be expected.
Authigenic P (Paut) and detrital P (Pdet).
In general, the concentrations and percentages of Paut ranged from 1.2 to 8.4 µmol g−1 and 4 to 30%, respectively. As expected from the significant relationship between Paut and Ca (Table 4), the lower part of the estuary was always characterized by the highest content in Paut for all seasons, particularly in summer when Paut can represent up to 30% of TP. Interestingly, the increase in the Paut fraction seems to occur at the expense of the Porg fraction, suggesting a potential adsorption of P released from organic matter mineralization on calcium carbonate during summer.
In general Pdet was quite similar to Paut in terms of concentration and distribution. Pdet concentrations were between 1.3 and 9.5 µmol g−1 (3–30% of TP). However, the upper section of the estuary was characterized by a high Pdet content. Pdet includes P associated with calcium present in apatite from igneous and metamorphic origin and is therefore usually transported by streams and rivers. The positive linear relationship observed between Pdet and Ca (Table 4) explains the high Pdet concentrations in the lower estuary due to high calcium concentrations. Nevertheless, the Pdet/Ca ratio in the lower estuary (Station 8) is substantially lower than in the rest of the estuary, suggesting that Pdet in the marine end-member might have been impoverished in P or diluted with Pdet from a different source. It is worth noting that if the data from Station 8 are excluded, the Pdetversus calcium relationship exhibits a better positive linear trend, as shown in Fig. 4.
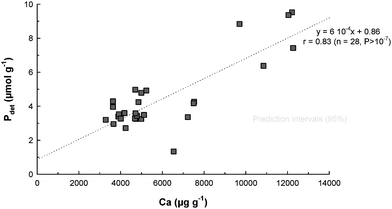 |
| Fig. 4 Relationship between detrital phosphorus (Pdet) and calcium concentrations in the surface sediment of the Tamar estuary (excluding marine stations). | |
Organic P (Porg).
The concentrations of Porg ranged from 1.1 to 13.9 µmol g−1 (3.4–32% of TP), constituting a relatively low average fraction of TP over the year (16%). During most of the year, the upper part of the estuary (Stations 2 and 3) received the highest quantity of Porg, suggesting that organic matter was mainly derived from the catchment. Nevertheless, in autumn the lower estuary (Stations 7 and 8) exhibited relatively high Porg concentrations, which might result from the input of organic matter produced in this area during summer.15 Overall, the Porg concentrations found in the Tamar estuary are similar to those observed in other temperate macrotidal estuaries.31–33
3.4. Relationship between solid-phase P speciation and grain size
Some significant correlations were observed between grain size and almost all forms of P as shown in Table 4. Pex, PFe and Porg were significantly positively correlated with the silt fraction (<63 µm) and more specifically with the finest size fraction (<22 µm) for PFe and Porg. The close relationships observed in this study between PFe, Porg and grain size are in good agreement with those reported in other coastal areas.31,34 For PFe in particular, it is usually hypothesized that this correlation results from the concentration of colloidal ferric oxyhydroxides plus associated Fe-bound P in the finer sediment particles because of their greater surface area for adsorption of ferric colloids.35 This hypothesis is confirmed for the Tamar estuary by the relationship between PFe and the sediment specific surface area shown in Fig. 5. The stations that were clearly dominated by particles >125 µm (e.g. Station 1) had specific surface areas up to 10 times lower than in the rest of the estuary (data not presented here), which explains the low PFe observed despite the presence of high iron concentrations. This confirms that differences in the P sorption properties of sediments can be partly explained on the basis of their physical characteristics.36
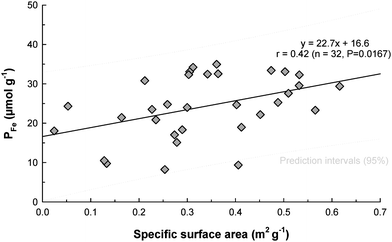 |
| Fig. 5 Relationship between PFe and sediment specific surface area in Tamar estuary sediments. | |
Detrital P (Pdet) showed the opposite trend to other P forms. Indeed Pdet was positively correlated with the coarser sediment material and also inversely correlated with the finest particle size fraction. As expected from the intrinsic properties of Pdet, i.e. apatite from rocks of igneous and metamorphic origin, it is “non-sensitive” to adsorption processes and usually concentrated in the coarser size fractions.34 This fraction is likely to be the most easily transported (mobile) fraction during high flow events and diluted with marine sediment further downstream.
Finally it is important to note that the only P fraction which did not show any significant correlation with grain size was authigenic P (Paut), which has also been observed in other temperate macrotidal estuaries31 and suggests that, like Pdet, Paut is not particularly subject to surface effects, probably due to the inclusion of calcium-bound phosphate within the sedimentary mineral matrix.
3.5. Importance of surface sediment in P cycling within the Tamar estuary
The Tamar is a partially mixed estuary with strong tidal mixing and moderate river flow that allows relatively good mixing of marine and fresh waters throughout the estuary. This relatively dynamic system minimizes extended periods of stratification and potential large hypoxia events at the bottom of the estuary, and the surface sediment stays mainly well oxygenated throughout the year. Therefore, the dominant sedimentary P fraction, PFe, should not play a major role in the potential release of P into the water column within the estuary. In fact, the sediment surface should act as a preventive barrier limiting P release via upward diffusion through the sediment–water interface. In this surface layer, more labile sedimentary P phases (i.e. Porg and Pex) are more likely to drive the mobility of P. In particular, the non-refractory fraction of the organic material is usually decomposed in the surface layer of the sediment. During summer, when favourable conditions for enhanced primary production exist, low river flow and rising temperature (up to 20.8 °C) are generally conducive to organic matter deposition and mineralization in the surface layers of the sediment. It is then hypothesized that this microbial degradation releases phosphate to the sediment pore water. During this study, the observed variations of Porg showed no obvious seasonal or spatial trends. The concomitant variations of DRP in the surface sediment pore water of the estuary were also quite variable37 and no significant correlations were found between those two variables, either annually or seasonally. Only Pex concentrations showed a significant relationship with P in Tamar sediment pore water. Interestingly, Pex was only weakly correlated with DRP in the autumn (r = 0.35, n = 8, P > 0.4) and winter (r = 0.32, n = 8, P > 0.4). Dissolved organic P concentrations were recently measured within the pore water samples used for this study.37 It appears that Pex and DOP concentrations were conversely characterized by significant positive correlations throughout the year, particularly in summer (Fig. 6). This was confirmed by exchangeable P, determined as PIDE, which gave positive correlations with DOP, especially for the dilutions carried out at salinities 20 and 35. Furthermore, Pex also appeared to be seasonally linked to the other sedimentary phases. Indeed, Pex concentrations were negatively correlated with PFe in winter and summer (r = 0.55 and 0.39, respectively, n = 8 and P > 0.4) and positively correlated with Porg in spring and autumn (r = 0.68, n = 8, P > 0.06). These results suggest that despite being the smallest fraction of sedimentary P within the estuary, Pex is the fraction that is most involved in P cycling. Surprisingly, the variations of DRP concentrations did not provide clear evidence of possible conversion of PFe or Porg to Pex. A possible explanation for this is that the kinetics of adsorption/desorption were very rapid,11 thus masking any potential net accumulation of DRP in the pore water.
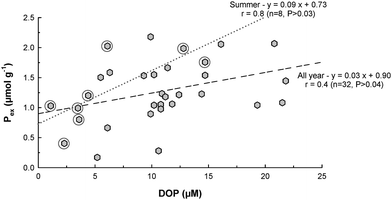 |
| Fig. 6 Relationship between exchangeable P (Pex) and dissolved organic phosphorus (DOP) in the surface sediment of the Tamar estuary. The circles highlight concentrations observed in summer. | |
The results of this study suggest, however, that DOP compounds may well be included in the exchangeable P pool. Sedimentary environments such as estuaries are generally relatively rich in organic matter. Indeed, besides containing natural marine organic matter, terrestrial organic matter is also important due to the proximity of the estuary to land and river outflow. Organic P compounds are primarily exported from rivers in association with the particulate phase due to their affinity for sediment particles38 and can be released into the water column during estuarine mixing. For example, Gardolinski et al.39 showed that substantial amounts of DOP were rapidly released from an aerobic freshwater sediment under conditions of increasing salinity (10–20) similar to the regime experienced when sediment and suspended particulate materials are transported into estuaries. A large proportion of the DOP released underwent rapid degradation and became bioavailable (approximately 37% of the total DRP release). Furthermore, Monbet et al.37 recently assessed the speciation of DOP in the water column and pore waters of the Tamar estuary. They showed that DOP was quantitatively involved in P cycling within the estuary and that a substantial fraction of this DOP was bioavailable (70%, as determined via enzymatic hydrolysis), both spatially and seasonally, throughout the estuary. More importantly, they highlighted the fact that the relative composition of DOP in these waters was very different to that generally found in soils,40 indicating transformation and/or degradation of the organic P pool when it reached the aquatic environment. The results of the present study confirm the important role of the sedimentary phase, via the exchangeable phosphorus pool (Pex), in the biogeochemical cycling of P throughout the Tamar estuary.
4. Conclusions
This study shows that P present in surface sediments of the Tamar estuary occurred mainly as PFe (up to 75%). The other fractions, Porg, Paut and Pdet, barely exceeded 16, 13 and 9% on average, respectively, and therefore constituted a much lower fraction of the TP pool. The lowest P fraction stored in the sediment (up to 12%) was exchangeable P (Pex/PIDE). The distribution of P in the estuary was also strongly influenced by particle morphology. The concentrations of most of the sedimentary P forms exhibited significant correlations with particulate grain size. Most P forms (Pex, PFe and Porg) were preferentially associated with the fine particles (<63 µm), and PFe and Porg were associated particularly with the finest size fraction (<22 µm). This confirms that differences in the P sorption properties of sediments can be partly explained by their physical characteristics and it should be possible to predict the concentrations of the different P forms for estuarine locations where physical data on sediment properties are available.
Overall, this study shows that P is stored in the sediment in relatively labile fractions, which suggests that some release into the water column is ultimately possible, depending on the local environmental conditions encountered. For the present dataset, a hypoxic event could cause a reduction of Fe oxides and lead to substantial release of P within the water column. However, as long as the surface sediment layer stays relatively well oxygenated (and this is favoured by the macrotidal character of the estuary), the redox state of this sediment layer should not be affected and will therefore preserve an effective barrier to prevent DRP migration from the sediment to the water column. Other P forms, such as Pex and Porg, are therefore more likely to be involved in P cycling in surface sediments of the Tamar estuary despite being quantitatively less important. In particular this study shows that exchangeable P and DOP in the pore water are intimately linked. The degradation of the organic P sedimentary fraction might therefore release some DOP compounds that interact with the exchangeable P fraction, especially during summer. The degree of lability of the organic matter, as well as the affinity of these organic compounds for the sediment particles, will therefore play a key role in the extent of adsorption/release via sedimentary exchangeable P. Assuming that the Tamar is representative of temperate, macrotidal, eutrophic estuaries, the findings of this study are broadly applicable to estuarine ecosystems where sediment readily interacts with the overlying water and this process will ultimately affect its trophic status.
References
-
R. A. Vollenweider, Organization for Economic Co-operation and Development, Directorate for Scientific Affairs, Paris, 1968, p. 159 Search PubMed.
- S. R. Carpenter, N. F. Caraco, D. L. Correll, R. W. Howarth, A. N. Sharpley and V. H. Smith, Issues Ecol., 1998, 3, 1 Search PubMed.
-
K. C. Ruttenberg, in Treatise on Geochemistry, ed. D. H. Heinrich and K. T. Karl, Pergamon, Oxford, 2003, p. 585 Search PubMed.
- P. Monbet, I. D. McKelvie and P. J. Worsfold, Environ. Chem., 2007, 4, 334 CAS.
- S. J. Schenau and G. J. De Lange, Mar. Chem., 2001, 75, 201 CrossRef CAS.
- C. P. Slomp, E. H. G. Epping, W. Helder and W. Van Rassphorst, J. Mar. Res., 1996, 54, 1179 CrossRef CAS.
- H. P. Jarvie, M. D. Jurgens, R. J. Williams, C. Neal, J. J. L. Davies, C. Barrett and J. White, J. Hydrol., 2005, 304, 51 CrossRef CAS.
- A. W. Morris, A. J. Bale and R. J. M. Howland, Estuarine, Coastal Shelf Sci., 1981, 12, 205 CrossRef CAS.
- P. G. Watson, P. E. Frickers and R. J. M. Howland, Neth. J. Aquat. Ecol., 1993, 27, 135 Search PubMed.
- K. C. Ruttenberg, Limnol. Oceanogr., 1992, 37, 1460 CAS.
- P. Froelich, M. L. Bender, N. A. Luedtke, G. R. Heath and T. DeVries, Am. J. Sci., 1982, 282, 474 Search PubMed.
- A. Aminot and F. Andrieux, Water Res., 1996, 30, 2805 CrossRef CAS.
- B. G. Rawlins, K. O’Donnell and M. Ingham, Geochemical survey of the Tamar catchment (south-west England), British Geological Survey Report CR/03/027, 2003, 232 pp.
- R. J. Uncles and J. A. Stephens, Estuaries, 1993, 16, 126 CrossRef.
- R. H. Jackson, P. J. Williams and I. R. Joint, Estuarine, Coastal Shelf Sci., 1987, 25, 299 CrossRef CAS.
- J. P. Mommaerts, J. Mar. Biol. Ass. U. K., 1969, 49, 749 Search PubMed.
- L. D. Anderson and M. L. Delaney, Limnol. Oceanogr., 2000, 45, 509 CAS.
- K. I. Aspila, H. Agemian and A. S. Y. Chau, Analyst, 1976, 101, 187 RSC.
- P. S. Ellis, A. J. Lyddy-Meaney, P. J. Worsfold and I. D. McKelvie, Anal. Chim. Acta, 2003, 499, 81 CrossRef CAS.
- R. L. Benson, I. D. McKelvie, B. T. Hart, Y. B. Truong and I. C. Hamilton, Anal. Chim. Acta, 1996, 326, 29 CrossRef CAS.
- R. N. Strom and R. B. Biggs, Estuaries, 1982, 5, 95 CrossRef CAS.
- E. Gomez, M. Fillit, M. C. Ximenès and B. Picot, Hydrobiologia, 1998, 373/374, 203 CrossRef.
- P. Monbet, G. J. Brunskill, I. Zagorskis and J. Pfitzner, Geochim. Cosmochim. Acta, 2007, 71, 2762 CrossRef CAS.
- K. C. Ruttenberg and R. A. Berner, Geochim. Cosmochim. Acta, 1993, 57, 991 CrossRef CAS.
- M. S. Koch, R. E. Benz and D. T. Rudnick, Estuarine, Coastal Shelf Sci., 2001, 52, 279 CrossRef CAS.
- P. Lopez, Mar. Geol., 2004, 203, 161 CrossRef CAS.
- S. M. Liu, J. Zhang and J. L. Li, Estuarine, Coastal Shelf Sci., 2004, 59, 209 CrossRef CAS.
- P. N. Froelich, Limnol. Oceanogr., 1988, 33, 649 CAS.
- P. C. F. C. Gardolinski, PhD Thesis, “In Situ Monitoring and Biogeochemical
Cycling of Nutrients in Estuarine Waters”, University of Plymouth, Plymouth, 2002..
- R. Raiswell and D. E. Canfield, Am. J. Sci., 1998, 298, 219 Search PubMed.
- F. Andrieux-Loyer and A. Aminot, Estuarine, Coastal Shelf Sci., 2001, 52, 617 CrossRef CAS.
- J. P. Coelho, M. R. Flindt, H. S. Jensen, A. I. Lillebø and M. A. Pardala, Estuarine, Coastal Shelf Sci., 2004, 61, 583 CrossRef CAS.
- F. Andrieux-Loyer, X. Philippon, G. Bally, R. Kérouel, A. Youenou and J. Le Grand, Biogeochemistry, 2008, 88, 213 CrossRef CAS.
- R. A. Berner and J. L. Rao, Geochim. Cosmochim. Acta, 1994, 58, 2333 CrossRef CAS.
- J. L. Rao and R. A. Berner, Mar. Geol., 1997, 139, 95 CrossRef CAS.
- P. V. Sundareshwar and J. T. Morris, Limnol. Oceanogr., 1999, 44, 1693 CAS.
- P. Monbet, I. D. McKelvie and P. Worsfold, Geochim. Cosmochim. Acta, 2009, 73, 1027 CrossRef CAS.
-
L. Celi and E. Barberis, in Organic Phosphorus in the Environment, ed. B. L. Turner, E. Frossard and D. S. Baldwin, CAB International, Wallingford, 2005, p. 113 Search PubMed.
- P. C. F. C. Gardolinski, P. J. Worsfold and I. D. McKelvie, Water Res., 2004, 38, 688 CrossRef CAS.
-
L. M. Condron, B. L. Turner and B. J. Cade-Menun, Phosphorus: Agriculture and the Environment, American Society of Agronomy, Madison, 2005 Search PubMed.
Footnote |
† Part of a themed issue dealing with water and water related issues. |
|
This journal is © The Royal Society of Chemistry 2010 |
Click here to see how this site uses Cookies. View our privacy policy here.