DOI:
10.1039/B813083N
(Tutorial Review)
Chem. Soc. Rev., 2010,
39, 150-155
Functional evolution on the assembled DNA template
Received 1st May 2009
First published on 16th September 2009
Abstract
After more than twenty years of effort, using DNA to fabricate addressable nanostructures is now a well-established technology. In this tutorial review, we attempt to present an overview of the applications of DNA templates, including its biological significance, the directing of chemical reactions at the molecular level, as well as the placing of nanoparticles and proteins in position.
 Lijin Xu | Lijin Xu was born in Qingdao, China. He studied chemistry at Qufu Normal University, and received in 1998, a PhD from the Institute of Chemistry, the Chinese Academy of Sciences. During 1998–2000 he held a two-year postdoctoral fellowship at the Leverhulme Centre for Innovative Catalysis, the University of Liverpool under the direction of Prof. Jianliang Xiao. After a one-year postdoctoral appointment at Loughborough University, he joined Prof. Albert S. C. Chan’s group as a research associate at the Hong Kong Polytechnic University. In 2005, he took up an associate professor position in the Department of Chemistry, Renmin University of China. His current interests mainly focus on transition-metal catalyzed organic synthesis, DNA-templated organic synthesis and green chemistry. |
 Dongsheng Liu | Professor Dongsheng Liu graduated from the University of Science and Technology of China with a BS degree in 1993. After working in the Institute of Chemistry, Chinese Academy of Sciences for six years, he went to the Hong Kong Polytechnic University and finished his PhD there under the supervision of Professor A. S. C. Chan in 2002. He worked as a postdoc research associate at Cambridge University, UK. In 2005, he became a principle investigator in the National Centre for NanoScience and Technology, China. In June 2009, he became a full professor in the Department of Chemistry, Tsinghua University. Now his research is mainly focused on using biomolecules to fabricate nanostructures and nanodevices. |
1. Introduction
Other than its biological significance, DNA has also been demonstrated to serve as a building block in the fabrication of nanostructures because of its precise base-pairing recognition and designable sequences.1 After more than twenty years of effort, researchers can now easily enable the fabrication of 2D and 3D structures. At this stage, finding applications for this technology emerges as one of the most important challenges in this field. In this tutorial review, we will use examples to illustrate the application of DNA assemblies, such as: directing chemical reactions, making cyclic DNAs, placing nanoparticles in position etc. These results demonstrate that DNA templates could serve as platforms to manipulate targets at the molecular level.2. DNA-templated synthesis of DNA analogues
2.1 DNA-templated enzymatic ligation reactions
To enable the biological functions, the replication of double helical DNA is an essential in vivo process.2 In this process, a number of short daughter complementary sequences are synthesized on the unwound parent strands first, which finally leads to the formation of a fully matched duplex with many non-chemically linked ‘nicks’ between daughter sequences. As the formed duplex can hold the 5′-phosphate and the 3′-hydroxy groups of two neighboring daughter DNA fragments in the ideal positions for the formation of a phosphodiester bond, these two daughter sequences then can be easily chemically linked via the formation of a phosphodiester bond catalyzed by DNA ligase. This is nature’s inspiration for the application of DNA assemblies.2.2 DNA-templated non-enzymatic ligation reactions
DNA carries the code of life and decides the structures of enzymes which are a kind of protein; meanwhile, the replication of DNA in vivo is catalyzed by enzymes. ‘Which one appears first?’ is a generally interesting topic in the past decades and a lot of chemists have devoted themselves to this question. In 1966, Naylor and co-workers first attempted the non-enzymatic DNA-templated phosphodiester formation reaction of two short thymidine oligonucleotides, activated by a carbodiimide with polyadenosine as a template in aqueous solution at −3 °C (Fig. 1).3 Although only 5% and 3% yields were observed, these results experimentally proved that DNA elongation without enzymes is feasible. It is notable that no reaction was observed in the absence of the template, demonstrating the power of DNA assembly. Afterwards, it was found that several types of chemical reactions could also enable the templated formation of phosphodiester bonds without the presence of any enzyme if the 5′-phosphate and the 3′-hydroxy groups are placed in the proper positions.4 For example, Shabarova and co-workers found BrCN could work more efficiently for the chemical formation of phosphodiesters than EDC (1-(3-(dimethylamino)propyl)-3-ethyl-carbodiimide hydrochloride) because of its high reaction rate and the lack of by-products.5 The activating capacity of BrCN was exemplified in the efficient synthesis of an artificial gene with 183 base pairs through DNA-templated ligation of complementary synthetic oligonucleotides through the formation of phosphodiester bonds in several minutes. Impressively, Orgel and his group have even demonstrated that 2-methylimidazole activated nucleic acid monomers could be successfully oligomerized into a fully complementary strand on a DNA template.6 |
| Fig. 1 The first DNA-templated chemical ligation. | |
Based on these findings, the chemical ligation reactions could also lead to some other interesting results. For example, von Kiedrowski and co-workers reported the autocatalytic behavior of the DNA-templated phosphodiester formation reaction on the basis of duplex formation.7 In related work, Li and Nicolaou described the chemical self-replication of a duplex via the formation of a triplex template.8
3. Functional evolution of DNA-templated chemical reactions
With well-developed DNA modification chemistry and the ability of DNA assemblies to place the 5′-phosphate and the 3′-hydroxy groups of DNA pieces in close proximity, more functions could be attained. By replacing the phosphate and hydroxy groups with different functional systems, the DNA supramolecular assembly could provide different disciplines, such as organic chemistry, materials science and nanotechnology, with a novel strategy to control the interactions between subjects at a molecular level and increase the effective molarity by DNA base pairing, which subsequently promote the development of new directions in these fields. Besides the use of simple secondary structures, the recent development of DNA nanotechnology, which now enables the fabrication of more complicated DNA assemblies, has also provided more feasibility for exploring new applications in new areas.3.1 The use of DNA as a template to control organic reactions
DNA-templated reactions are not just for the synthesis of structural analogs of nucleic acid, and recent studies have indicated that in combination with the flexibility and power of modern synthetic organic chemistry it could be widely used to incorporate a number of functionalized groups unrelated to nucleic acids at a particular site of the nucleic acid backbone. In general, these templated reactions proceed in aqueous solution with single-stranded DNA as the templates, and the temperature is around room temperature or lower because higher temperature would destabilize the DNA hybridization. A DNA-templated organic reaction involving the formation of a non-nucleotide functional group was first reported in 1992 by Lynn and co-workers, who found that the DNA template could facilitate the reagent oligonucleotides bearing a 5′-amino and 3′-formyl to form imines in the absence of any added condensation agent (Fig. 2A).9 Lestinger and Kool described the DNA-templated substitution reactions of DNA oligonucleotides bearing a 5′-electrophilic group with the neighboring DNA oligonucleotide bearing a 3′-nucleophilic group without employing any activating reagent, and moderate to high sequence fidelities were observed (Fig. 2B).10 Fujimoto et al. developed an efficient DNA-templated photoligation of an oligonucleotide containing 5-vinyldeoxyuridine at the 5′-end with a 3′-thymine substituted oligonucleotide, giving rise to up to a 96% yield (Fig. 2C).11 The irradiation by long-wavelength UV light of 366 nm led to a smooth photoligation between the substrates without damaging the DNA components, and reliable photoreversibility was confirmed upon irradiating the photoligated product at 302 nm.More impressive developments have been made by Liu and co-workers.12 They disclosed that a number of useful organic reactions, such as amide-bond formation, reductive amination, aldol reaction, nitro-aldol, nitro-Michael, Wittig olefination, 1,3-dipolar cycloaddition, alkene-alkyne coupling and Heck coupling reactions could run well even at nanomolar absolute concentrations regardless of different requirements in the transition-state geometry, steric hindrance and conformational flexibility (Fig. 3). These reactions proceeded in a high sequence-specific manner, and no product was detected when mismatches were introduced into the sequence of the template or the reagent DNA fragment. In combination with appropriate linkers and purification strategies, multistep DNA-templated organic synthesis has been successfully implemented, further demonstrating the general applicability of this templated process. It is notable that DNA templates can simultaneously direct several different types of synthetic reaction within the same solution, even though the reactants involved would be cross-reactive and therefore incompatible under traditional synthesis conditions. More recently, Brown and co-workers disclosed that the DNA-templated click reaction between DNA fragments bearing an azide and alkyne, respectively, could proceed smoothly, providing DNA strands with an unnatural triazole linkage.13
 |
| Fig. 3 Different types of DNA-templated organic reactions. | |
Considering the stability of DNA conjugates, DNA-templated organic reactions are limited to only the structures that can be produced in aqueous solvents. However, a recent study by Liu and co-workers has indicated that these reactions could also proceed smoothly in a variety of organic solvents (such as CH3CN, DMF, THF and CH2Cl2) with low or minimal water content.14 This result will enable water insoluble reagents to be used in DNA-templated reactions, thus greatly improving their applicability.
All the DNA-templated organic reactions described above are limited to only reactions between two DNA-linked synthetic molecules, but reactions involving non-tethered reagents have been less explored. In 2002, von Kiedrowski et al. described the efficient coupling of three hydrazide-linked oligonucleotides with free trimesaldehyde in the presence of a branched DNA Y-shaped template (Fig. 4A).15 More recently Marx and Tang have demonstrated that a DNA-linked proline could efficiently catalyze the cross-aldol reaction between an aldehyde tethered to a complementary DNA strand and a free ketone (Fig. 4B).16 A series of ketones were examined and up to 99% yields were observed. It is notable that the reaction ran smoothly even using DMSO as the co-solvent when water-insoluble ketones were employed.
 |
| Fig. 4 DNA-templated reactions of non-tethered reagents: (A) tris-hydrazone formation; (B) aldol reaction. | |
Recently, a useful strategy to efficiently tune DNA-templated chemical reactions based on DNA conformational change has been developed by Mao and Chen (Fig. 5).17 They found that varying the effective concentration of reagents in response to a change in pH could induce the conformational transition between the triplex and duplex DNA structures of a 5′-carboxylate linked DNA template, hybridized by two 5′-amino linked DNA strands, therefore enabling the selective amidation of the carboxylate with the adjacent amine group. Alternatively, Yan et al. reported that the addition of a control DNA strand could trigger the chemical reaction of the two reactants linked by two spatially separated DNA oligonucleotides.18
 |
| Fig. 5 DNA-templated organic reaction with tunable reactivity (reprinted with permission from ref. 17). | |
In addition to many useful organic reactions, DNA-templated chemistry has been demonstrated to be able to direct the preparation of metal complex–DNA conjugates. Sheppard and co-workers reported that two DNA fragments containing salicylaldehyde moieties at the 3′- or 5′-end aligned on a single-stranded DNA template with a spacer region were brought into close proximity to produce a metallosalen-DNA conjugate upon addition of ethylenediamine and a divalent metal (Fig. 6).19 The effect of the spacer was examined, and the spacer consisting of two nucleotide residues was the optimal choice for the formation of metallosalen-DNA complexes. Just like many other DNA-templated reactions, the presence of mismatches compromised the reaction yields. It merits mention that this strategy was soon followed by Gothelf et al. to synthesize metallosalen-DNA conjugates with various nanostructures.20
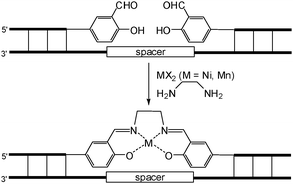 |
| Fig. 6 DNA-templated synthesis of metallosalen-DNA complexes. | |
3.2 DNA-templated preparation of cyclic DNAs
Double-stranded cyclic DNA structures, such as plasmid DNA, play an important role in vivo. Meanwhile, single-stranded cyclic DNA has attracted much attention as an excellent antisense candidate because its lack of ends makes it less sensitive to digestion by exonucleases compared with its linear format. Even though the circularization process of double-stranded DNA is a proven biological process catalyzed by enzymes, the developments of DNA assembly design and non-enzymatic ligation chemistry have motivated the quest for novel strategies in preparing abnormal cyclic DNAs. For example, Shabarova and co-workers have demonstrated that linear single-stranded DNA sequences could be circularized efficiently by chemical means templated by duplex DNA assemblies.21 Circular dumbbell DNAs have been prepared by Ashley and Kushlan with DNA self-templated chemical ligations.22 In 1991, Dervan et al. circularized a special double-stranded DNA by the triplex DNA templated chemical ligation process.23 As shown in Fig. 7A, the DNA precursor is a 3.7 kilobase pair blunt-ended linear DNA duplex, which cannot be circularized by the normal biological process, but the 15 base pair purine tract at each end could form a triplex on a single-stranded Hoogsteen template, and then undergo chemical ligation in the presence of N-cyanoimidazole to produce the circular DNA. Also based on triplex structures, Kool and co-workers developed a convergent approach, in which two half-length DNA strands are ligated twice in one step to produce a series of single-stranded cyclic DNAs ca. 30–70 bp in length from one or two linear single-stranded DNA precursors with good yields (Fig. 7B).24 It is noteworthy that cyclic DNAs prepared by this method have no internal base pair formation under physiological conditions.To maintain stability of the DNA assembly in the ligation process, the substrate DNA strand participating in the formation of the assembly must possess a minimum length, thus the cyclic DNA prepared by the above mentioned methods normally cannot be smaller than 30 nucleotide residues even without carrying any extra functional analogues. In 2001, Chan and co-workers reported a new strategy which can prepare smaller single-stranded cyclic DNAs efficiently.25 As shown in Fig. 8A, with the formation of quadruplex DNA, an i-motif, the 5′-phosphate and 3′-hydroxy termini of a designed linear cytosine-rich single-stranded DNA were aligned in the proper position to be covalently linked in the presence of N-cyanoimidazole. Because the i-motif structure could only be stable under slight acidic conditions, the cyclic DNAs prepared via this method do not have internal base pair formation under physiological conditions. In a similar way, a G-quadruplex could also be used as the template for the preparation of cyclic DNA (Fig. 8B).26 By changing the DNA analogue, the DNA assembly can be easily tuned, thereby influencing the products after ligation. For example, the 27 mer single-stranded DNA 5′-pCC(TAACCCC)3TAACC-3′ could fold on itself to form an intramolecular i-motif structure and subsequently result in one cyclic DNA; meanwhile, a much shorter sequence 5′-pCCTCCCTTC-3′ can only form an intermolecular i-motif structure where the two molecules use each other as templates and lead to the preparation of much smaller cyclic DNA.27 This result not only fulfils the size gap between direct circularization and previous assembly-directed circularization, but also illustrates an unusual phenomenon that this prepared small cyclic DNA migrates faster than its linear format in denatured polyacrylamide gel electrophoresis. This finding and the migration properties of previously reported single-stranded cyclic DNA could be excellent examples for studying physical properties of cyclic DNAs.
In 1996, Alivisatos and Schultz et al. developed a method to prepare a gold nanoparticle with only one single-stranded DNA modification.28 Based on this approach, they also demonstrated that, via DNA assembling, two or more gold nanoparticles could be annealed onto a single-stranded DNA template to form homodimeric and homotrimeric nanoparticle assemblies (Fig. 9). Interestingly, the above mentioned single-stranded cyclic DNA has enabled a rolling circle amplification reaction that can easily generate very long single-stranded DNA containing numerous repeated units. On this template, Mao and co-workers have reported the preparation of a micrometres-long linear gold nanoparticle array.29 Considering that a long enough single-stranded DNA could bear numerous binding sites which are different to each other and have sufficient stability, the hetero-nanoparticles assembly could be prepared theoretically with practical nanoparticle modification technology. Obviously, this is a useful technology for materials science and physics. More recently, Sleiman and co-workers have developed some new DNA templates containing rigid vertices, which are capable of organizing gold nanoparticles into various cyclic structures through DNA assembling.30Assembling nanoparticles on a linear template is an important progress but is not the destination of this technology as it can only provide one dimensional controllability.30–32 Along with the maturation of two and three dimension DNA nanostructure fabrication, now DNA supramolecular assembly can enable more controllability with more objects. For example, in 2004, Yan and co-workers demonstrated that periodic protein arrays could be achieved by templated self-assembly of streptavidin onto the DNA nanogrids containing biotinylated oligonucleotides.33 Further with a two-step metallization procedure, the DNA template could act as an excellent scaffold for the production of highly conductive, uniform-width silver nanowires. Very recently Yan and his team have pushed this technology to another peak, as shown in Fig. 10; single-stranded DNA modified gold nanoparticles are attached to designed positions of two dimensional DNA tile lattices, and the assembling process could lead to the closing up of lattices and the formation of tubular three-dimensional architectures, ranging in shape from stacked rings to single spirals, double spirals, and nested spirals.34 The nanoparticles are active elements that control the preference for specific tube conformations through size-dependent steric repulsion effects. The assembly of nanoparticles could allow for greater control of the interactions between these particles or with molecules.
 |
| Fig. 10 The design of a DNA tile system for the formation of a variety of tubular structures carrying 5-nm AuNPs. (A and B) Top and side views of the four DX tiles (A tile, blue; B tile, red; C tile, green; and D tile, brown). The A tile carries a 5-nm AuNP on the top of the tile. The C tile carries a DNA stem loop pointing downward. (C) The four different tiles are designed to self-assemble into a 2D array displaying parallel lines of AuNPs. (D) Possible ways for the corresponding edge tiles on opposite sides of the 2D array to associate and lead to the formation of tubes displaying patterns of AuNPs in stacked rings, single spirals, double spirals, and nested spiral tubes. (E) The different tube conformations were observed in a single TEM image (reprinted with permission from ref. 34). | |
4. Outlook
In this review, we have briefly summarized the application of DNA assemblies, from simple duplex formation to elegant two dimension origami, as templates for chemical reactions and protein or nanoparticle organization. However, the function of DNA assembly in all these applications is the same, namely placing an object in the right position. At the present time, the type of object is very limited, thus restricting the wider applications. But with this concept and the development of DNA modification technology, DNA assembly will become a useful platform for studying the interactions between different subjects at the single molecule level. Yan and his co-workers have pioneered this direction recently. As shown in Fig. 11, by using DNA assembly to control the distance between two ligands, the cooperative binding affinity of these two ligands to the target could be well controlled.35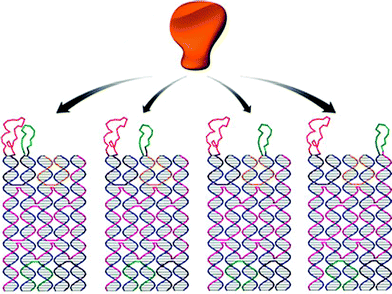 |
| Fig. 11 Schematic illustration on how binding affinity is influenced by the distance between ligands (reprinted with permission from ref. 35). | |
Acknowledgements
The authors would like to thank Prof. Qinghua Fan in the Institute of Chemistry, Chinese Academy of Sciences for helpful discussion and NSFC under grant No. 20725309 (for DL) and 20873179 (for LX), NSFC-DFG joint project TRR61 and MOST under grant No. 2007CB935902 for financial supports.References
- N. C. Seeman, Nature, 2003, 421, 427–431 CrossRef.
- R. H. Garrett and C. M. Grisham, Biochemistry, Brooks/Cole Publishing Company, 3rd edn, 2005 Search PubMed.
- R. Naylor and P. T. Gilham, Biochemistry, 1966, 5, 2722–2728 CrossRef CAS.
- Y. Gat and D. G. Lynn, in Templated Organic Synthesis, ed. P. J. Stang and F. Diederich, Wiley-VCH, Weinheim, 1999, pp. 133–153 Search PubMed.
- N. G. Dolinnaya and Z. A. Shabarova, Russ. Chem. Bull., 1996, 45, 1787–1809 Search PubMed.
- L. E. Orgel, Acc. Chem. Res., 1995, 28, 109–118 CrossRef CAS.
- G. von Kiedrowski, Angew. Chem., Int. Ed. Engl., 1986, 25, 932–935 CrossRef.
- T. Li and K. C. Nicolaou, Nature, 1994, 369, 218–221 CrossRef CAS.
- J. T. Goodwin and D. G. Lynn, J. Am. Chem. Soc., 1992, 114, 9197–9198 CrossRef CAS.
- A. P. Silverman and E. T. Kool, Chem. Rev., 2006, 106, 3775–3789 CrossRef CAS.
- K. Fujimoto, S. Matsuda, N. Takahashi and I. Saito, J. Am. Chem. Soc., 2000, 122, 5646–5647 CrossRef CAS.
- X. Li and D. R. Liu, Angew. Chem., Int. Ed., 2004, 43, 4848–4870 CrossRef CAS.
- A. H. El-Sagheer and T. Brown, J. Am. Chem. Soc., 2009, 131, 3958–3964 CrossRef CAS.
- M. M. Rozenman and D. R. Liu, ChemBioChem, 2006, 7, 253–256 CrossRef CAS.
- L. H. Eckardt, K. Naumann, W. M. Pankau, M. Rein, M. Schweitzer, N. Windhab and G. von Kiedrowski, Nature, 2002, 420, 286 CrossRef CAS.
- Z. Tang and A. Marx, Angew. Chem., Int. Ed., 2007, 46, 7297–7300 CrossRef CAS.
- Y. Chen and C. Mao, J. Am. Chem. Soc., 2004, 126, 13240–13241 CrossRef CAS.
- R. Chhabra, J. Sharma, Y. Liu and H. Yan, Nano Lett., 2006, 6, 978–983 CrossRef CAS.
- J. L. Czlapinski and T. L. Sheppard, J. Am. Chem. Soc., 2001, 123, 8618–8619 CrossRef CAS.
- K. V. Gothelf, A. Thomsen, M. Nielsen, E. Clo and R. S. Brown, J. Am. Chem. Soc., 2004, 126, 1044–1046 CrossRef CAS.
- N. G. Dolinnaya, M. Blumenfeld, I. N. Merenkova, T. S. Oretskaya, N. F. Krynetskaya, M. G. Ivanovskaya1, M. Vasseur and Z. A. Shabarova, Nucleic Acids Res., 1993, 21, 5403–5407 CAS.
- G. W. Ashley and D. M. Kushlan, Biochemistry, 1991, 30, 2927–2933 CrossRef CAS.
- K. J. Luebke and P. B. Dervan, J. Am. Chem. Soc., 1991, 113, 7447–7448 CrossRef CAS.
- E. Rubin, S. Rumney, S. Wang and E. T. Kool, Nucleic Acids Res., 1995, 23, 3547–3553 CrossRef CAS.
- T. Li, D. Liu, J. Chen, A. H. F. Lee, J. Qi and A. S. C. Chan, J. Am. Chem. Soc., 2001, 123, 12901–12902 CrossRef CAS.
- J. Chen, D. Liu, A. H. F. Lee, J. Qi, A. S. C. Chan and T. Li, Chem. Commun., 2002, 2686–2687 RSC.
- D. Liu, J. Chen, A. H. F. Lee, L. M. C. Chow, A. S. C. Chan and T. Li, Angew. Chem., Int. Ed., 2003, 42, 797–799 CrossRef CAS.
- A. P. Alivisatos, K. P. Johnsson, X. Peng, T. E. Wilson, C. J. Loweth, M. P. Bruchez, Jr and P. G. Schultz, Nature, 1996, 382, 609–611 CrossRef CAS.
- Z. Deng, Y. Tian, S. Lee, A. E. Ribbe and C. Mao, Angew. Chem., Int. Ed., 2005, 44, 3582–3585 CrossRef CAS.
- F. A. Aldaye, A. L. Palmer and H. F. Sleiman, Science, 2008, 321, 1795–1799 CrossRef CAS.
- K. V. Gothelf and T. H. LaBean, Org. Biomol. Chem., 2005, 3, 4023–4037 RSC.
- C. Lin, Y. Liu, S. Rinker and H. Yan, ChemPhysChem, 2006, 7, 1641–1647 CrossRef CAS.
- H. Li, S. H. Park, J. H. Reif, T. H. LaBean and H. Yan, J. Am. Chem. Soc., 2004, 126, 418–419 CrossRef CAS.
- J. Sharma, R. Chhabra, A. Cheng, J. Brownell, Y. Liu and H. Yan, Science, 2009, 323, 112–116 CrossRef CAS.
- S. Rinker, Y. Ke, Y. Liu, R. Chhabra and H. Yan, Nat. Nanotechnol., 2008, 3, 418–422 Search PubMed.
|
This journal is © The Royal Society of Chemistry 2010 |