Received 20th August 2009, Accepted 2nd October 2009
First published on 6th November 2009
Abstract
Photo-ionization mass spectrometry and electric beam deflection experiments were used to study isolated SnMPbN clusters (7 ≤N + M≤ 13 for tin-rich clusters, 7 ≤N + M≤ 15 for lead-rich clusters) in a molecular beam apparatus. The observed mass spectra reveal a broad abundance distribution of the bimetallic clusters in which all possible cluster compositions can be identified within the investigated size ranges. Comparison of the relative cluster intensities between pure tin or lead clusters (SnN+M and PbN+M) and mixed SnMPbN clusters indicate quite similar relative abundance distributions which can be smoothly shifted from one to the other extreme by changing the composition. The mass spectroscopic findings could be explained by assuming a substitution “alloy” formation in the SnMPbN cluster system. In combination, the dielectric properties were determined by passing the bimetallic clusters through an inhomogeneous electric field. The observed polarizabilities are significantly increased for most of the bimetallic clusters. This can be explained in an adiabatic polarization model by the presence of permanent electric dipole moments. These observations demonstrate how the electronic properties are not only crucially influenced by the cluster size but also by the composition of this nanoalloy model system. In addition to the enhanced polarizability, most of the measured beam profiles for tin-rich clusters show detectable beam broadenings due to the permanent dipole moments, in contrast to lead-rich clusters which possess considerable smaller dipole moments. Molecular dynamic simulations of the measured beam profile for Sn6Pb1 taking theoretically calculated isomeric structures and dipole moments into account yields no completely satisfying outcome. Therefore we discuss possible reasons for the discrepancy between experimental and theoretical results.
I. Introduction
Nanoalloy clusters have received much attention recently, because the physical properties of these nanosized systems can be varied by size and composition. This is not only due to the potential applications of small bimetallic clusters in electronics, optics, catalysis and as storage media1 but the bimetallic clusters are also of general interest, since the understanding of the electronic and geometric structure of these systems is crucial in nanoscience. However, up to now there are only a few experimental examples which demonstrate how the optical,2 magnetic3 and catalytic4 behaviour changes with size and composition. Besides these promising results a clear correlation between size and composition of the bimetallic clusters and the observed behaviour is still lacking. This is due to the high complexity of the studied systems, because not only the chemical composition but also the spatial distribution of the different atoms can have great impact on the observed properties.5 Furthermore the electronic and geometric structure of clusters are not only influenced by intrinsic effects but also depend on solvent/ligand6 molecules or the support material.7 It is therefore reasonable to minimize the complexity by studying nanoalloys in the gas phase to separate the intrinsic properties of the bimetallic clusters from environmental effects. This additionally enables a simplified theoretical approach to discuss the experimental findings. Following this procedure the stability of gas phase alloy clusters has been investigated by Lievens et al.8–10 using mass spectrometry in combination with quantum chemistry identifying bimetallic clusters with enhanced stability, i.e.“magic numbers” of mixed clusters. In addition photoelectron spectroscopy (PES) was used to study the electronic structure of bimetallic clusters11 and when combined with electron diffraction can be used as a probe for the geometric structure.12 While Koretsky et al. measured the ionization potentials of CoNMnM clusters13 to correlate these to the observed reactivities, Neumaier et al. studied CO adsorption and dissociation kinetics of the system AgMAuN+ to extract structural information by combining the experimental results with density functional theory (DFT) calculations.14 Further studies with drift mobility measurements15 were used to obtain structural information by comparing calculated and experimental cross sections of AgMAuN+ clusters. By using a free electron laser Fielicke et al. were able to obtain geometric structures of bimetallic clusters with the help of infrared multiple photon dissociation (IR-MPD) experiments in combination with DFT calculations.16,17 The magnetic properties of nanoalloy model systems can be studied in Stern–Gerlach experiments. For CoNMnN18 the magnetic moment of cobalt can be enhanced when the pure cluster is doped with Mn atoms which is in contrast to the bulk alloy while for MnSnN19 clusters the non-magnetic component strongly influences the magnetization dynamics. In analogy to magnetic deflection experiments it is possible to measure the dielectric response of a cluster beam in an inhomogeneous electric field. For pure clusters the observed polarizabilities and electric dipole moments can be used as a probe for the electronic20,21 and geometric22 structure, but also to reveal temperature induced dynamics.23–26 Another approach to probe the structure of bimetallic clusters was used by Waldschmidt et al., who were able to obtain informations concerning the energetics by comparing surface induced dissociation (SID) spectra of SnMPb+ and SnPbN+ clusters with theoretical predictions.27In the present paper we studied SnMPbN clusters using time-of-flight mass spectrometry (TOF-MS) and electric beam deflection experiments. Since a mixture of these components shows a miscibility gap in the bulk material, it is instructive to study the composition of the tin–lead alloy clusters with the help of TOF-MS. Using electric beam deflection experiments a clear discrimination between tin-rich and lead-rich alloy clusters will be possible. The observed polarizabilities of the bimetallic species will be interpreted within an adiabatic polarization model and the measured beam profiles will be discussed by numerically solving the Euler equations taking DFT calculations into account.
The molecular beam apparatus used in the experiments is described elsewhere28 and therefore only reported briefly. In order to generate bimetallic clusters, it was necessary to produce a mixed vaporization target to study SnMPbN clusters in the gas phase. Therefore two mixed tin–lead rods were synthesized, the first containing 24 atom% Sn (rod 1: 22.5 g Pb and 4.0 g Sn) and the second 81 atom% Sn (rod 2: 5.2 g Pb and 12.4 g Sn). In an evacuated quartz tube the two metals (Sn 99.99%, Alfa-Aesar; Pb 99.95%, GoodFellow) were molten, carefully mixed and then rapidly cooled down (faster than 5 min) to produce a polycrystalline rod. After trimming the two rods to the required diameter they could be used as vaporization targets. The bimetallic clusters are then produced by a laser vaporization source. A plasma is formed by irradiating pulsed light (1064 nm) of a Nd:YAG (yttrium aluminium garnet) laser on a composite tin–lead rod. The plasma is cooled down by injection of a helium pulse whereby it condenses to form mixed tin–lead clusters. The helium–cluster mixture is then expanded through a nozzle into a high vacuum apparatus, thereby forming a supersonic beam of SnMPbN clusters. In order to control the supersonic beam expansion the leading 25 mm of the 61 mm long nozzle (2 mm diameter) can be cooled with the help of a helium refrigerator down to 40 K. By reducing the nozzle temperature the kinetic energy of the clusters in the molecular beam is lowered, in addition to the fact that a cooling of the internal degrees of freedom of the clusters occurs. In the present work, experiments were performed with a nozzle temperature of 50 K. After the supersonic expansion a double skimmer narrows the beam diameter before passing a homemade chopper for measuring the cluster velocities.29 Behind two collimators, the molecular beam reaches the inhomogeneous electric “two-wire” field, which is oriented perpendicular to the molecular beam axis.30 The distance between the two electrodes is 1.5 mm and the maximum value of the electric field, which could be generated, is 2 × 107 V m−1 at an applied deflection voltage U of 30 kV. After passing a field free drift region (about 1200 mm) the alloy clusters pass a moveable slit having a width of 400 μm and are subsequently ionized by a low fluence excimer laser (7.89 eV). The ionized clusters enter a TOF-MS, are deflected perpendicular to the molecular beam axis and are detected with the help of an Even-cup. By repeating this procedure for different slit positions p the molecular beam profiles can be obtained. The mass spectra can be extracted from the deflection experiment raw data.In order to quantify the measured electric dipole moments and polarizabilities, the known polarizability of the Ba atom was used as calibration of the apparatus.28,31 Therefore the reported dielectric properties have an absolute uncertainty of about 8%. The uncertainties arising from the velocity and deflection measurements are indicated by error bars.
III. Results and discussion
Fig. 1 shows a typical mass spectrum obtained for the bimetallic SnMPbN clusters using a lead-rich rod for laser vaporization. The assignment of the different peaks was done by creating a calibration function (Fig. 1, inset). The calibration function has been adapted by the well known flight times of the atoms and the pure tin and lead clusters. Since the TOF is proportional to the square root of the cluster mass (only single charged clusters are assumed throughout this paper) it is possible to assign the mass peaks of the mixed clusters. However, due to the broad isotope distribution of tin and the fact that seven tin atoms differ by only 2 amu in mass from 4 lead atoms a few bimetallic clusters coincide with pure SnN+M, PbN+M or other SnMPbN cluster sizes. For a further mass peak assignment the beam deflection profiles were additionally used. Since the dielectric response of the pure SnN+M32 and PbN+M33 clusters is well known it is possible to use the deflection information as a probe for different cluster species. Due to the remarkable difference between the obtained beam profiles of the bimetallic (following section) and pure clusters for many cluster sizes, mainly in the high voltage regime, at least a discrimination between pure and mixed clusters is possible. This approach can not completely rule out the presence of more than one cluster composition but shows that the fraction of other species is to small to significantly contribute to the measured molecular beam profiles. Therefore it is legitimate to assume a minor contribution to the observed peak intensity. A discrimination between two mixed cluster species (no peaks with more than two coinciding masses were analysed) was done by comparing the beam deflection profiles obtained from rod 1 and rod 2. First the beam profiles of the peaks corresponding to the same mass, obtained from the two different rods, were computed. If the profiles can be discriminated two different mixed cluster species have to be responsible. Therefore the profile obtained from rod 1 was assigned to the possible lead-rich and from rod 2 to the possible tin-rich cluster composition (for an example see Fig. S1 in ESI†). The described procedures enabled a mass peak assignment for SnMPbN clusters with 7 ≤N + M≤ 13 (tin-rich) and 7 ≤N + M≤ 15 (lead-rich) atoms. The most obvious finding for SnMPbN clusters is that every possible composition of the bimetallic clusters can be identified within the mentioned size range. However, this mass spectroscopic result gives no information about the spatial distribution of the atoms in the clusters, i.e. the “miscibility” of the two components is still unclear. In order to be able to extract more information from the recorded mass spectra we analysed the relative intensities Ii/∑Ij of SnMPbN clusters possessing 7 ≤N + M≤ 13 atoms, whereby ∑Ij is the sum over all cluster intensities with the same number of dopant atoms, also including the specific cluster intensity Ii. The results are given in Fig. 2. One has to keep in mind that for charged clusters the intensities of the mass spectra combined with quantum chemical calculation can often be correlated to the overall stability of the gas phase species.10 However in the case of neutral clusters the abundance distribution is influenced by the ionization process,34 as well, and does not necessarily reflect the stability and thereby the occurrence of the neutral clusters in the molecular beam. However, a comparison of pure and mixed clusters under the same experimental conditions can reveal possible similarities or differences between mixed SnMPbN and pure SnN+M or PbN+M clusters. The relative intensities of the pure clusters are given in the inset of Fig. 2. Comparing these intensity profiles with those of the mixed clusters (Fig. 2) shows striking similarities indeed. The intensity distribution for SnMPb1 (Sn1PbN) is comparable to that of SnM+1 (PbN+1). Increasing the number of dopant atoms for a cluster with a fixed value of N + M slightly shifts the relative intensity for these species. This can clearly be seen for the series SnM−1Pb2 and SnM−2Pb3 (Sn2PbN−1 and Sn3PbN−2) for which the differences between SnM+N (PbM+N) and mixed clusters become more obvious. On the other hand the relative intensity of tin (lead) rich clusters changes continuously for the transition to pure lead (tin) clusters. This effect is more pronounced for clusters for which the tin to lead ratio is changed significantly by replacement of one tin to one lead atom and vice versa. This smooth shift from one to the other extreme points to the suggestion of a substitution “alloy” formation in the SnMPbN cluster system. This idea is supported by theoretical and experimental results. Theoretical calculations predict an enhanced stability on Sn10.35 In agreement with this the mass spectra show an increased relative intensity of Sn10 but also of all other clusters SnMPbN with N + M = 10. A similar substitution of tin and lead in stable clusters was also found in solution by the identification of mixed tin–lead Zintl-ions via NMR spectroscopy.36 These mass spectroscopic findings combined with the mentioned theoretical and experimental evidences make the idea of a substitutional “alloy” reasonable.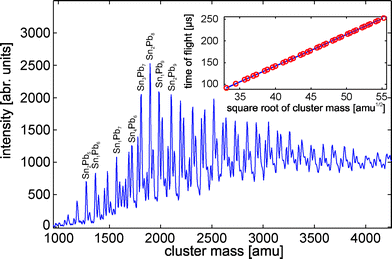 |
| Fig. 1 TOF mass spectrum of SnMPbN clusters. The bimetallic clusters were obtained by vaporizing a mixed Sn/Pb target containing 24 atom% Sn (rod 1). The conversion of the detected TOF into the cluster mass was done by the calibration function shown in the inset. A few peaks are labeled with the corresponding cluster composition. | |
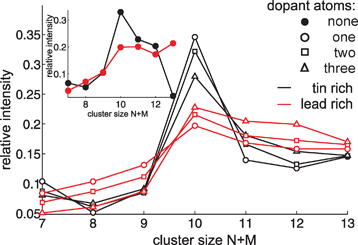 |
| Fig. 2 Relative abundance distribution for SnMPbN clusters with 7 ≤N + M≤ 13 atoms. In the inset the relative abundance distribution of pure SnN+M and PbN+M clusters is shown. Connecting lines are used as a guide to the eye. | |
B Deflection experiments
By passing a cluster beam through an inhomogeneous electric field it is deflected due to the Stark effect. The deflection for a specific cluster SnMPbN in the quantum state |n〉 is given by: | 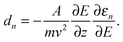 | (1) |
Here m represents the cluster mass, E and ∂E/∂z the deflection field and deflection field gradient, v the cluster velocity, A an apparatus function and ∂εn/∂E the Stark effect of the cluster in the specific quantum state |n〉 with energy εn. The apparatus function A depends on the geometry of the electrodes generating the inhomogeneous field and the distance between the electric field and the detection region of the clusters.37 As mentioned before A was calibrated by taking the polarizability value of (39.7 ± 3.2) Å3 for the Ba atom into account.31 Due to a distribution of quantum states of one cluster size in the molecular beam, the experimentally observed beam profile represents an ensemble average 〈·〉 of the deflections dn.The simplest approach, which is valid for a spherical, rigid cluster with a rotational energy far exceeding the interaction energy, is a first-order perturbation treatment.32 In this approximation one can correlate the mean value of the deflection distribution d with the polarizability α:
| 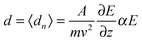 | (2) |
and the variance
b2 with the electric dipole moment
μ:
| 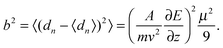 | (3) |
The dielectric properties of the mixed
clusters are computed within first-order perturbation theory (FOPT) approximation by fitting Gaussians to the
experimental data points for the field on and field off case, in order to determine
d and
b2 from the shift of the maxima of the Gaussians and from the square root of the difference of the variances (see Fig. S3–S8 in ESI
†).
The molecular deflection profiles for Sn8Pb2 (25 kV) and Sn2Pb8 (28 kV) are given as examples in Fig. 3. For tin-rich clusters measurements at 10, 15, 25 and 28 kV are available. However, many tin-rich clusters show a strong beam broadening at high deflection voltages, though a reasonable description of the profiles with Gaussians is no longer possible. Therefore the experimental data for tin-rich clusters at 28 kV were not used for the first-order perturbation analysis, however it can be used to highlight the differences between tin- and lead-rich clusters (see Fig. S2 in ESI†). Hence the perturbation analysis was done using 10, 15 and 25 kV measurements for tin-rich clusters and 20 and 28 kV for lead-rich clusters, respectively (see Fig. 3 as example). It is obvious from Fig. 3 that the dielectric response for SnMPbN, containing the same overall number of atoms and dopants, depends strongly on the composition. The tin-rich cluster shows an observable beam broadening, while the lead-rich cluster is almost only deflected towards high field strength. This has nothing to do with the increased mass of the lead-rich cluster, since the higher deflection voltage nearly compensates the anticipated mass dependence in this particular case. This difference in the dielectric response of tin- and lead-rich clusters can be generalized. It is obvious from Fig. 4 that tin-rich clusters possess larger dipole moments than lead-rich clusters. The squared electric dipole moments per atom for SnMPb1–3 and Sn1–3PbN are comparable in the case of small cluster sizes. Though increasing the cluster size slightly lowers the dipole moments for lead-rich clusters, while the dipole moments of tin-rich clusters show considerable variation. A comparison with the pure cluster species shows the influence of the dopant atoms on the dipole moments. Tin-rich clusters possess an enhanced electric dipole moment for nearly all clusters sizes. Otherwise the dopant atoms do not change the dipole moment significantly for lead-rich cluster with a few exceptions. So a minor additional contribution due to the dopant atoms to the dipole moment can be identified for small lead-rich cluster (N + M about 7–9). On the other hand a clear decrease of the dipole moment for N + M = 12 and 14 and a minor one for N + M = 10 is obvious. The second experimental observable, the polarizability per atom, also shows a difference between tin- and lead-rich clusters which can be rationalized by an adiabatic polarization model. The experimental data points are given in Fig. 5 by blue circles. Nearly all cluster polarizabilities significantly exceed the value of a metal sphere with bulk properties (computed by weighting the Wigner–Seitz radii of the elements with the composition of the cluster) including a spill-out within the jellium model.38 However, the experimental data points can be reproduced by taking the determined dipole moments per atom (Fig. 4) into account. A contribution of the dipole moment to the observed polarizability is given by:
| 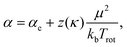 | (4) |
as derived for an adiabatic entrance of rigid
clusters into an electric field by Becker
et al., using second-order perturbation theory.
39,40 Here
α and
αe represent the overall and the pure electronic polarizability,
μ stands for the electric dipole moment,
Trot represents the rotational temperature of the
cluster ensemble,
kb the Boltzmann constant and
z(
κ) is a factor that depends on the moments of inertia of the
cluster. In this paper
z(
κ) is set to 2/9, assuming nearly spherical tops. To get an idea of the order of magnitude of the dipole contribution Sn
10Pb
2 is considered. From FOPT one obtains the dielectric parameters (43.6 ± 2.0) Å
3 and (0.74 ± 0.1) D. Taking the pure electronic jellium polarizability of 10.9 Å
3 and a rotational temperature of 2.5 K into account, the permanent dipole moment contributes (29.3 ± 4.1) Å
3 to the observed polarizability, which agrees reasonably well with the experimental value within the experimental uncertainty. For a rotational temperature of (3.0 ± 0.5) K the adiabatic model fits the observed experimental trend for the polarizabilities qualitatively and often quantitatively (
Fig. 5). A rotational temperature of about 3 K seems reasonable compared to previous electric deflection studies
33 and Stern–Gerlach experiments
41 under same or similar experimental conditions. The correspondence between experimental values and the polarization model is surprisingly good, keeping in mind that the
clusters might not be spherical in the studied size range, nor rigid. A deviation between experiment and the second-order perturbation model can therefore probably be attributed to the mentioned assumptions of a rigid, nearly spherical rotor, which might not be valid for all
cluster sizes. Nevertheless the observed dipole moments and polarizabilities demonstrate a remarkable difference between
tin- and
lead-rich alloy
clusters, which should be addressable to a different electronic or geometric structure.
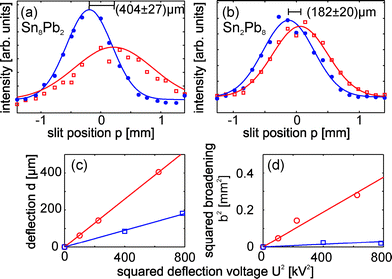 |
| Fig. 3 Beam profiles for (a) Sn8Pb2 and (b) Sn2Pb8 at 50 K nozzle temperature and a electric field strength of 1.7 × 107 V m−1 (a) and 1.9 × 107 V m−1 (b). Blue circles (red squares) show the experimental data points without (with) applied deflection voltage. As a guide to the eye Gaussians are fitted to the data points. In (c) and (d) the predicted linear dependence of deflection (variance) and the squared deflection voltage, given in eqn (2) (eqn (3)), is valid within the experimental uncertainty. Sn8Pb2 (Sn2Pb8) is represented by red circles (blue squares). | |
![Squared dipole moments per atom [μ/(N + M)]2 obtained from the deflection measurements for (a) SnMPb1 (blue squares) and Sn1PbN (red squares), (b) SnMPb2 (blue squares) and Sn2PbN (red squares) and (c) SnMPb3 (blue squares) and Sn3PbN (red squares). The squared dipole moments for pure tin (broken, blue line) and lead (broken, red line) at 50 K nozzle temperature are plotted for comparison.32,33 Connecting lines are used as a guide to the eye.](/image/article/2010/CP/b917206h/b917206h-f4.gif) |
| Fig. 4 Squared dipole moments per atom [μ/(N + M)]2 obtained from the deflection measurements for (a) SnMPb1 (blue squares) and Sn1PbN (red squares), (b) SnMPb2 (blue squares) and Sn2PbN (red squares) and (c) SnMPb3 (blue squares) and Sn3PbN (red squares). The squared dipole moments for pure tin (broken, blue line) and lead (broken, red line) at 50 K nozzle temperature are plotted for comparison.32,33 Connecting lines are used as a guide to the eye. | |
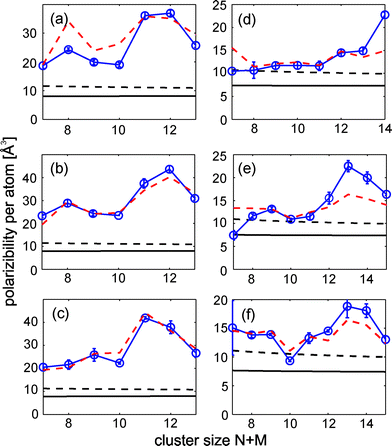 |
| Fig. 5 Obtained polarizability per atom α/(N + M) for SnMPb1 (a), SnMPb2 (b), SnMPb3 (c) , Sn1PbN (d), Sn2PbN (e) and Sn3PbN (f) taking eqn (2) and the measurements at an electric field strength of 6.7 × 106, 1.0 × 107 and 1.7 × 107 V m−1 (1.3 × 107 and 1.9 × 107 V m−1) for tin rich (lead-rich) clusters into account (blue circles, connecting lines are used as a guide to the eye). The polarizability of a metal sphere with bulk properties (solid, black line) is slightly increased, when a spill-out of 0.5 Å is included (broken, black line). A semi-quantitative description for most cluster sizes is possible by further considering a dipole contribution to the polarizability for a nearly spherical top (eqn (4)) and a rotational temperature of (3.0 ± 0.5) K (broken, red line). | |
While some quantum chemical calculations have predicted the structure of pure SnN+M32,35,42 and PbN+M43,44 clusters, theoretical investigations of mixed clusters are rare. First calculations by Waldschmidt et al. for SnMPb and SnPbN (N + M = 7–12) substitution alloy clusters indicate the preference of tin atoms to occupy higher coordinated sites in lead-rich clusters, while the situation is reversed for the tin-rich clusters.27 From this follows that tin-rich clusters should possess a peripheral lead atom and lead-rich clusters should exhibit tin atoms located as near as possible to the center. This finding would give rise to an increased dipole moment in tin-rich clusters due to the more outlying polarizable lead atoms. Therefore the observed dipole moments can be qualitatively explained by a geometric effect caused by the preferred bonding of the group 14 atoms and not necessarily reflect a dopant induced charge density deformation. Our experimental data confirms this theoretically predicted trend for singly doped clusters as well as for clusters with a higher number of dopants. Additionally the theoretically predicted structural motifs27 for singly doped clusters change only slightly in comparison to the pure clusters, which agrees with the mass spectroscopic interpretation mentioned before.
To correlate the observed dielectric behaviour to the geometric structure of the cluster, we tried to model the deflection behaviour by molecular dynamic (MD) simulations first introduced by Dugourd et al.45 in order to analyse the beam profiles of asymmetric rotors.46,47 For that purpose theoretically predicted isomer structures and the dielectric properties are taken into account. The moments of inertia and the electric dipole moment of one isomer structure are used to numerically solve the classical equations of motion for a rigid body, i.e. the Euler equations, in an electric field. This is done for several initial conditions, sampling a thermal ensemble adiabatically entering the electric field. This results in a distribution of averaged effective dipole moments and by using eqn (1) in a distribution of deflections. By convoluting this deflection distribution with the profile without an electric field the beam profile with applied deflection voltage is obtained. It was shown that this approach can be used to distinguish different cluster isomers which are present in the molecular beam experiments.22,32
As an example we analysed the beam profiles of Sn6Pb using the described procedure, since only two isomers are possible according to the substitution “alloy” idea. The two isomers are generated using the ground-state structure of Sn732 and subsequent substitution of either an axial or equatorial tin atom with a lead atom. The properties of these cluster isomers were calculated within density functional theory using the B3LYP functional as implemented in the Gaussian 03 software package.48 At first the structures were geometry optimized using the Stuttgart–Dresden effective core potentials (ECP) and the corresponding basis sets for the four valence electrons (abbreviated as LC) in Gaussian 03. An additional single point calculation was performed for both geometrically relaxed isomers treating the outer 22 electrons for every atom explicitly with a corresponding (12s12p9d3f2g)/[6s6p3f2g] basis while a small core ECP (abbreviated as SC) was used to replace the remaining core electrons, both provided by the Stuttgart group.49 The theoretically predicted simulation parameters are given in Table 1.
Table 1 Results obtained for Sn6Pb by quantum chemical calculations. The nomenclature for the isomers and quantum chemical methods are given in the text. The dipole moments μ in D and the moments of inertia I in 10−44 kg m2 are given in the principal axis system. The relative energy difference ΔE is stated in eV. The predicted energy difference between {1} and {2} at the SC level of the theory has to be treated with some care, since the energies are calculated at the equilibrium LC geometry
| Ia | Ib | Ic | μa | μb | μc | |μ| | ΔE |
---|
{1} Sn6Pb (LC) | 4.70 | 5.75 | 8.18 | 0.95 | 0.00 | 0.00 | 0.95 | 0.00 |
{2} Sn6Pb (LC) | 5.15 | 5.15 | 7.11 | 0.00 | 0.00 | 0.55 | 0.55 | 0.06 |
{1} Sn6Pb (SC) | 4.70 | 5.75 | 8.18 | 0.65 | 0.00 | 0.00 | 0.65 | 0.00 |
{2} Sn6Pb (SC) | 5.15 | 5.15 | 7.11 | 0.00 | 0.00 | 0.49 | 0.49 | 0.11 |
The simulation results are shown in Fig. 6. While the quantum chemical calculation for the small basis set overestimates the dipole moment and therefore the beam broadening, the single point calculation improve the simulation results but a satisfying description of the beam profiles can not be obtained. Obviously the second isomer ({2}), a prolate rotor, fits the experimental data points better than the asymmetric rotor ({1}), which is predicted as the ground-state structure by Waldschmidt et al.27 and by our calculations. When the dipole moments of the SC method are virtually scaled by a factor of 0.8 the isomer {2} nearly perfectly agrees with the experimental data points whereas isomer {1} is not able to reproduce the beam profile. This still existing discrepancy could be addressable to some theoretical factors. For lead clusters it is well known, that the spin–orbit (SO) coupling can have great impact on the observed properties and the geometric structure.11,50,51 Since our calculations do not include SO coupling, and the foregoing theoretical investigation only include SO coupling to correct the absolute energy, it is unclear what effect the SO coupling has on the dipole moment, the geometrical structure and the relative energy scale in this particular case. Furthermore there are no theoretical investigations which systematically search the configuration space for possible SnMPbN cluster isomers and perhaps reveal other than substitution alloy ground-state structures. Our experimental results point to a substitution “alloy” formation but this idea can not be validated by MD simulations because a sufficiently large number of isomers is not available yet. In addition there are also experimental observations which can be responsible for the observed discrepancy. Tin clusters at 50 K nozzle temperature show a decrease of the observed dipole moments due to the excitation of vibrational modes in comparison to the 40 K measurements. A nozzle temperature of 100 K is high enough to quench the dipole moment for some cluster sizes completely.32 Likewise experimental results for lead clusters also point to a vibrationally induced quenching of the dipole moment at 50 K.33 Therefore the discrepancy between theoretical and experimental results for Sn6Pb can also be caused by vibrationally excited clusters. Furthermore, it has been shown52,53 that strongly asymmetric rotors in an electric field can have chaotic rotational trajectories, which can quench the observed beam broadening. This might also explain the difference in the observed deflected beam profile compared to the simulation utilizing the putative global minimum structure {1}. Hence a systematic theoretical investigation and beam deflection experiments with reduced nozzle temperatures are needed to clarify the reason for the observed discrepancy.
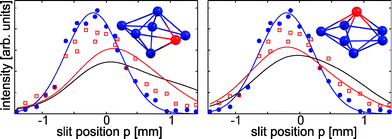 |
| Fig. 6 The experimental data points without (with) deflection field (1.0 × 107 V m−1) for Sn6Pb are given by blue circles (red squares). When only the valence electrons are treated explicitly, the simulated black beam profiles poorly describe the measured data points. If a computationally more demanding basis set is used the red beam profiles were obtained. For the simulations a rotational temperature of 3 K yielded the best results. In the upper right panel of the figure, the corresponding isomers (Sn: blue, Pb: red) are shown. | |
C Conclusion
By analyzing the photo-ionization mass spectra of mixed SnMPbN clusters with different compositions we have shown, that there are indications for substitutional alloy formation in this system. We corroborated this idea by molecular beam electric field deflection experiments, which revealed a strong composition dependent polarizability and dipole moment of SnMPbN alloy clusters which is clearly distinguishable from pure clusters. The polarizabilities for the “alloy” clusters were analysed within a semiquantitative adiabatic polarization model and the dielectric properties were used to obtain first evidences for structural motifs of SnMPbN clusters.
Acknowledgements
We acknowledge financial support by the Deutsche Forschungsgemeinschaft through Grant No. SCHA885/7-2.References
- R. Ferrando, J. Jellinek and R. L. Johnston, Chem. Rev., 2008, 108, 845–910 CrossRef CAS.
- W. Benten, N. Nilius, N. Ernst and H.-J. Freund, Phys. Rev. B: Condens. Matter Mater. Phys., 2005, 72, 045403 CrossRef.
- V. Abdelsayed, G. Glaspell, M. Nguyen, J. M. Howe and M. S. El-Shall, Faraday Discuss., 2008, 138, 163–180 RSC.
- S. Zhou, B. Varughese, B. Eichhorn, G. Jackson and K. McIlwrath, Angew. Chem., Int. Ed., 2005, 44, 4539–4543 CrossRef CAS.
- J. Jellinek, Faraday Discuss., 2008, 138, 11–35 RSC.
- H. Xiang, J. Kang, S.-H. Wei, Y.-H. Kim, C. Curtis and D. Blake, J. Am. Chem. Soc., 2009, 131, 8522–8526 CrossRef CAS.
- B. Yoon, H. Hakkinen, U. Landman, A. S. Wörz, J.-M. Antonietti, S. Abbet, K. Judai and U. Heiz, Science, 2005, 307, 403–407 CrossRef CAS.
- Z. Chen, S. Neukermans, X. Wang, E. Janssens, Z. Zhou, R. E. Silverans, R. B. King, P. v. R. Schleyer and P. Lievens, J. Am. Chem. Soc., 2006, 128, 12829–12834 CrossRef CAS.
- E. Janssens, S. Neukermans, H. M. T. Nguyen, M. T. Nguyen and P. Lievens, Phys. Rev. Lett., 2005, 94, 113401 CrossRef CAS.
- S. Neukermans, E. Janssens, Z. F. Chen, R. E. Silverans, P. v. R. Schleyer and P. Lievens, Phys. Rev. Lett., 2004, 92, 163401 CrossRef CAS.
- L.-F. Cui, X. Huang, L.-M. Wang, J. Li and L.-S. Wang, Angew. Chem., Int. Ed., 2007, 46, 742–745 CrossRef CAS.
- L.-M. Wang, J. Bai, A. Lechtken, W. Huang, D. Schooss, M. M. Kappes, X. C. Zeng and L.-S. Wang, Phys. Rev. B: Condens. Matter Mater. Phys., 2009, 79, 033413 CrossRef.
- G. M. Koretsky, K. P. Kerns, G. C. Nieman, M. B. Knickelbein and S. J. Riley, J. Phys. Chem. A, 1999, 103, 1997–2006 CrossRef CAS.
- M. Neumaier, F. Weigend, O. Hampe and M. M. Kappes, J. Chem. Phys., 2006, 125, 104308 CrossRef.
- P. Weis, O. Welz, E. Vollmer and M. M. Kappes, J. Chem. Phys., 2004, 120, 677–684 CrossRef CAS.
- L. Lin, T. Höltzl, P. Gruene, P. Claes, G. Meijer, A. Fielicke, P. Lievens and M. T. Nguyen, ChemPhysChem, 2008, 9, 2471–2474 CrossRef CAS.
- P. Gruene, A. Fielicke, G. Meijer, E. Janssens, V. T. Ngan, M. T. Nguyen and P. Lievens, ChemPhysChem, 2008, 9, 703–706 CrossRef CAS.
- S. Yin, R. Moro, X. Xu and W. A. de Heer, Phys. Rev. Lett., 2007, 98, 113401 CrossRef.
- U. Rohrmann, S. Schäfer and R. Schäfer, J. Phys. Chem. A, 2009, 113, 12115, DOI:10.1021/jp906140b.
- D. Rayane, A. Allouche, E. Benichou, R. Antoine, M. Aubert-Frecon, P. Dugourd, M. Broyer, C. Ristori, F. Chandezon, B. Huber and C. Guet, Eur. Phys. J. D, 1999, 9, 243–248 CrossRef CAS.
- R. Antoine, D. Rayane, A. R. Allouche, M. Aubert-Frecon, E. Benichou, F. W. Dalby, P. Dugourd, M. Broyer and C. Guet, J. Chem. Phys., 1999, 110, 5568–5577 CrossRef CAS.
- S. Schäfer and R. Schäfer, Phys. Rev. B: Condens. Matter Mater. Phys., 2008, 77, 205211 CrossRef.
- A. Carrera, M. Mobbili and E. Marceca, J. Phys. Chem. A, 2009, 113, 2711–2714 CrossRef CAS.
- R. Antoine, D. Rayane, E. Benichou, P. Dugourd and M. Broyer, Eur. Phys. J. D, 2000, 12, 147–151 CrossRef CAS.
- D. Rayane, R. Antoine, P. Dugourd, E. Benichou, A. R. Allouche, M. Aubert-Frécon and M. Broyer, Phys. Rev. Lett., 2000, 84, 1962 CrossRef CAS.
- I. Compagnon, R. Antoine, D. Rayane, M. Broyer and P. Dugourd, Phys. Rev. Lett., 2002, 89, 253001 CrossRef CAS.
- B. Waldschmidt, S. Barman, C. Rajesh, C. Majumder, G. P. Das and R. Schäfer, Phys. Rev. B: Condens. Matter Mater. Phys., 2009, 79, 045422 CrossRef.
- S. Schäfer, M. Mehring, R. Schäfer and P. Schwerdtfeger, Phys. Rev. A: At., Mol., Opt. Phys., 2007, 76, 052515 CrossRef.
- L. P. Maguire, S. Szilagyi and R. E. Scholten, Rev. Sci. Instrum., 2004, 75, 3077–3079 CrossRef CAS.
- A. Salop, E. Pollack and B. Bederson, Phys. Rev., 1961, 124, 1431–1438 CrossRef CAS.
- H. L. Schwartz, T. M. Miller and B. Bederson, Phys. Rev. A: At., Mol., Opt. Phys., 1974, 10, 1924–1926 CrossRef CAS.
- S. Schäfer, B. Assadollahzadeh, M. Mehring, P. Schwerdtfeger and R. Schäfer, J. Phys. Chem. A, 2008, 112, 12312–12319 CrossRef CAS.
- S. Schäfer, S. Heiles, J. A. Becker and R. Schäfer, J. Chem. Phys., 2008, 129, 044304 CrossRef.
- D. N. Shin, Y. Matsuda and E. R. Bernstein, J. Chem. Phys., 2004, 120, 4157–4164 CrossRef CAS.
- B. Assadollahzadeh, S. Schäfer and P. Schwerdtfeger, J. Comput. Chem., 2009 DOI:10.1002/jcc.
- W. L. Wilson, R. W. Rudolph, L. L. Lohr, R. C. Taylor and P. Pyykko, Inorg. Chem., 1986, 25, 1535–1541 CrossRef CAS.
- T. M. Miller and B. Bederson, Adv. At. Mol. Phys., 1989, 25, 37–60 Search PubMed.
- D. R. Snider and R. S. Sorbello, Phys. Rev. B: Condens. Matter, 1983, 28, 5702 CrossRef.
- M. Schnell, C. Herwig and J. A. Becker, Z. Phys. Chem., 2003, 217, 1003–1030 CrossRef CAS.
- J. Bulthuis, J. A. Becker, R. Moro and V. V. Kresin, J. Chem. Phys., 2008, 129, 024101 CrossRef CAS.
- S. Pokrant, Phys. Rev. A: At., Mol., Opt. Phys., 2000, 62, 051201 CrossRef.
- C. Majumder, V. Kumar, H. Mizuseki and Y. Kawazoe, Phys. Rev. B: Condens. Matter Mater. Phys., 2001, 64, 233405 CrossRef.
- X.-P. Li, W.-C. Lu, Q.-J. Zang, G.-J. Chen, C. Z. Wang and K. M. Ho, J. Phys. Chem. A, 2009, 113, 6217–6221 CrossRef CAS.
- C. Rajesh and C. Majumder, J. Chem. Phys., 2007, 126, 244704 CrossRef.
- P. Dugourd, R. Antoine, M. A. E. Rahim, D. Rayane, M. Broyer and F. Calvo, Chem. Phys. Lett., 2006, 423, 13–16 CrossRef CAS.
- A. Carrera, M. Mobbili, G. Moriena and E. Marceca, Chem. Phys. Lett., 2008, 467, 14–17 CrossRef CAS.
- R. Moro, J. Bulthuis, J. Heinrich and V. V. Kresin, Phys. Rev. A: At., Mol., Opt. Phys., 2007, 75, 013415 CrossRef.
- M. J. Frisch, G. W. Trucks, H. B. Schlegel, G. E. Scuseria, M. A. Robb, J. R. Cheeseman, J. A. Montgomery, T. Vreven, K. N. Kudin, J. C. Burant, J. M. Millam, S. S. Iyengar, J. Tomasi, V. Barone, B. Mennucci, M. Cossi, G. Scalmani, N. Rega, G. A. Petersson, H. Nakatsuji, M. Hada, M. Ehara, K. Toyota, R. Fukuda, J. Hasegawa, M. Ishida, T. Nakajima, Y. Honda, O. Kitao, H. Nakai, M. Klene, X. Li, J. E. Knox, H. P. Hratchian, J. B. Cross, C. Adamo, J. Jaramillo, R. Gomperts, R. E. Stratmann, O. Yazyev, A. J. Austin, R. Cammi, C. Pomelli, J. W. Ochterski, P. Y. Ayala, K. Morokuma, G. A. Voth, P. Salvador, J. J. Dannenberg, V. G. Zakrzewski, S. Dapprich, A. D. Daniels, M. C. Strain, O. Farkas, D. K. Malick, A. D. Rabuck, K. Raghavachari, J. B. Foresman, J. V. Ortiz, Q. Cui, A. G. Baboul, S. Clifford, J. Cioslowski, B. B. Stefanov, G. Liu, A. Liashenko, P. Piskorz, I. Komaromi, R. L. Martin, D. J. Fox, T. Keith, M. A. Al-Laham, C. Y. Peng, A. Nanayakkara, M. Challacombe, P. M. W. Gill, B. Johnson, W. Chen, M. W. Wong, C. Gonzalez and J. A. Pople, GAUSSIAN 03 (Revision B.02), Gaussian, Inc., Pittsburgh, PA, 2003 Search PubMed.
- B. Metz, H. Stoll and M. Dolg, J. Chem. Phys., 2000, 113, 2563–2569 CrossRef CAS.
- K. Balasubramanian and D. Majumdar, J. Chem. Phys., 2001, 115, 8795–8809 CrossRef CAS.
- C. Zhao and K. Balasubramanian, J. Chem. Phys., 2002, 116, 10287–10296 CrossRef CAS.
- M. Abd El Rahim, R. Antoine, M. Broyer, D. Rayane and P. Dugourd, J. Phys. Chem. A, 2005, 109, 8507–8514 CrossRef CAS.
- R. Antoine, M. A. El Rahim, M. Broyer, D. Rayane and P. Dugourd, J. Phys. Chem. A, 2006, 110, 10006–10011 CrossRef CAS.
Footnotes |
† Electronic supplementary information (ESI) available: Fig. S1: Comparison of the obtained beam profiles for rod 1 and rod 2. Fig. S2: Molecular beam profiles of Sn8Pb3 and Sn3Pb8. Fig. S3–S8: Deflection and squared broadening vs. the squared deflection voltage for a number of SnMPbN clusters. See DOI: 10.1039/b917206h |
‡ Present address: Arthur Amos Noyes Laboratory of Chemical Physics, California Institute of Technology, Mail Code 127-72, 1200 East California Boulevard, Pasadena, CA 91125, USA. |
|
This journal is © the Owner Societies 2010 |