DOI:
10.1039/B910219A
(Paper)
CrystEngComm, 2010,
12, 216-225
Controlled synthesis, structures and properties of one-, two-, and three-dimensional lanthanide coordination polymers based on (8-quinolyloxy)acetate†
Received
26th May 2009
, Accepted 14th August 2009
First published on
3rd September 2009
Abstract
Hydrothermal reactions of Ln(NO3)3 and (8-quinolyloxy)acetic acid (HQOA) with auxiliary ligands resulted in a series of new one-, two- and three-dimensional (1D, 2D and 3D) coordination polymers, namely, [Ln(QOA)2 (H2O)2]·NO3·H2O [Ln = Eu (1), Gd (2)], [Ln2(QOA)4(ox)(H2O)]·3H2O [Ln = Pr (3), Er (4)], [Ln2(QOA)4(BDC) (H2O)]·4H2O [Ln = Sm (5)] and [Ln(QOA)(BDC)] [Ln = Sm (6), Eu (7)] [QOA = (8–quinolyloxy)acetate; ox = oxalate; BDC = 1, 4–benzenedicarboxylate], respectively. Compounds 1–2 possess 1D zigzag chains bridged by QOA anions and 3–4 contain 1D folded chains alternately bridged by oxalates and QOA anions. Complex 5 is an ordered (2,4)–connected 2D sheet-like structure. In compounds 6–7, four BDC anions bridge two adjacent metal atoms to form dinuclear lanthanide building blocks and these dinuclear blocks are further connected together to form an 8-connected 3D network. The progressive variation from 1D zigzag chains (1–2) to 1D folded chains (3–4) to a 2D layer network (5) and to 3D frameworks (6–7) is mainly attributed to the change of bridging ligands as well as the different coordination modes of organic carboxylic ligands. Moreover, the thermal stabilities and photoluminescent properties of these compounds show a remarkable difference due to the progressive variation of crystal structures.
Introduction
In recent years, the synthesis and rational design of lanthanide-polycarboxylate coordination polymers remain a popular research area due to their fascinating network topologies and promising applications in fields such as luminescent devices, magnetism, catalyst and selective adsorption.1–5 It is well documented that lanthanide contraction effect,6 the abundant coordination modes of the polycarboxylate ligands as well as the choice of versatile bridging ligands are the key factors that greatly affect the structures of lanthanide complexes.
Carboxylate ligands usually adopt diverse coordination modes such as terminal monodentate, chelating to one metal atom and bridging bidentate in syn–syn, syn–anti or anti–anti configuration to two metal atoms, etc.7 Thus, a great number of lanthanide-carboxylate coordination polymers, which exhibit 1D chains, 2D grids and layers, 3D networks, diamondoids, interpenetrated modes and helical networks have been reported.8 In addition, some suitable bridging ligands such as 4,4′-bipyridine9 and oxalate10 adding to the reaction mixture may act as linkers to connect isolated or low-dimensional metal coordination units, forming high-dimensional coordination networks.
(8-Quinolyloxy)acetic acid (HQOA), with both a flexible and a rigid moiety, has multifunctional coordination sites with chelating and bridging ability through N, O atoms of quinolyl and carboxylate group. In solid structures, intermolecular interactions such as hydrogen bonds and π–π stacking interactions are often observed. Earlier studies have mainly focused in the transition metal (Cu(II), Ni(II), Co(II)) complexes with HQOA molecules, which mostly show low-dimensional (0D or 1D) structural features.11 Herein, we present the synthesis, crystal structures and properties of lanthanide coordination polymers formed with (8-quinolyloxy)acetate under hydrothermal conditions. We also utilized bridging ligands such as oxalic acid and 1,4-benzenedicarboxylic acid to construct unprecedented lanthanide coordination networks. The seven lanthanide complexes are: [Ln(QOA)2(H2O)2]·NO3·H2O [Ln = Eu (1), Gd (2)], [Ln2(QOA)4(ox)(H2O)]·3H2O [Ln = Pr (3), Er (4)], [Ln2(QOA)4(BDC)(H2O)]·4H2O [Ln = Sm (5)] and [Ln(QOA)(BDC)] [Ln = Sm (6), Eu (7)] [QOA = (8-quinolyloxy)acetate; ox = oxalate; BDC = 1,4-benzenedicarboxylate].
Experimental
Materials and physical measurements
The ligand (8-quinolyloxy)acetic acid (HQOA) was prepared according to the literature.11 Lanthanide nitrate salts were prepared by dissolving the corresponding lanthanide oxides in 1
:
1 nitric acid (v/v) followed by drying. The other commercially available reagents were of reagent grade and used as received without further purification.
The C,H,N elemental analyses were determined with a Thermo FlashEA112 elemental analyzer. FT-IR spectra were recorded on a Shimadzu IR Prestige–21 spectrometer by using a KBr pellet in the range of 4000∼400 cm−1. Thermogravimetric analyses (TG) were preformed on a Netzsch STA409PC Thermal Analyzer with a heating rate of 10 °C min−1 up to 800 °C. Fluorescent spectra in the solid state were recorded on a Hitachi F – 2500 spectrophotometer with a Xenon arc lamp as the light source at room temperature.
Synthesis of [Eu(QOA)2(H2O)2]·NO3·H2O (1).
Eu(NO3)3·6H2O (89.2 mg, 0.20 mmol) and (8-quinolyloxy)acetic acid (101.5 mg, 0.50 mmol) were mixed in 8 ml deionized water and the pH value of the resulting solution was adjusted to about 7 with triethylamine. Then, the mixture was placed in a Teflon lined stainless steel reactor and heated at 20 °C h−1 to 120 °C for 24 h under autogenous pressure. After the sample was slowly cooled to room temperature at a rate of 5 °C h−1, pale-yellow block crystals of 1 were collected by filtration and washed with water and ethanol several times with a yield of 45%. Anal. calcd for C22H22EuN3O12: C 39.30; H 3.30; N 6.25; found: C 39.43; H 3.52; N 6.42%. IR (KBr pellet, cm−1): 3366 vs, 2963 m, 1657 m, 1622 s, 1580 m, 1510 s, 1418 m, 1383 s, 1315 m, 1260 vs, 1184 m, 1113 vs, 1082 m, 1024 m, 822 m, 763 m.
Synthesis of [Gd(QOA)2(H2O)2]·NO3·H2O (2).
2 was synthesized in a similar procedure to that described for 1, except that Eu(NO3)3 was replaced by Gd(NO3)3 (90.3 mg, 0.20 mmol). Colorless block crystals of 2 were collected with 41% yield. Anal. calcd for C22H22GdN3O12: C 38.99; H 3.27; N 6.20; found: C 38.87; H 3.03; N 6.07%. IR (KBr pellet, cm−1): 3374 vs, 1659 m, 1622 s, 1580 s, 1510 s, 1476 m, 1418 m, 1383 s, 1344 m, 1315 m, 1261 s, 1184 m, 1115 s, 1082 m, 1018 m, 824 m, 779 m, 764 m.
Synthesis of [Pr2(QOA)4(ox)(H2O)]·3H2O (3).
A mixture of Pr(NO3)3·6H2O (130.5 mg, 0.30 mmol), HQOA (121.9 mg, 0.60 mmol), oxalic acid (27.0 mg, 0.30 mmol) and NaOH (40.0 mg, 1.0 mmol) in 8 ml deionized water was placed in a Teflon-lined stainless steel reactor and heated at 120 °C for 24 h under autogenous pressure. Light-green block crystals of 3 were collected by filtration and washed with water and ethanol several times with a yield of 52%. Anal. calcd for C46H40N4O20Pr2: C 44.18; H 3.22; N 4.48; found: C 44.30; H 3.45; N 4.63%. IR (KBr pellet, cm−1): 3418 vs, 1624 vs, 1508 s, 1474 m, 1423 s, 1381 m, 1315 s, 1261 m, 1182 m, 1115 s, 1082 m, 1018 m, 826 m, 789 w, 770 m.
Synthesis of [Er2(QOA)4(ox)(H2O)]·3H2O (4).
The synthesis of 4 was similar to the above description for 3 except that Pr(NO3)3 was replaced by Er(NO3)3 (138.4 mg, 0.30 mmol). The pale-orange block crystals of 4 were isolated in 46% yield. Anal. calcd for C46H40Er2N4O20: C 42.39; H 3.09; N 4.30; found: C 42.52; H 3.27; N 4.45%. IR (KBr pellet, cm−1): 3448 vs, 1628 vs, 1508 m, 1474 m, 1422 m, 1383 m, 1315 s, 1261 m, 1182w, 1113s, 1082w, 1018w, 824 w, 789 m, 772 w.
Synthesis of [Sm2(QOA)4(BDC)(H2O)]·4H2O (5).
Sm(NO3)3·6H2O (88.9 mg, 0.20 mmol), HQOA (81.3 mg, 0.40 mmol) and 1,4-benzenedicarboxylic acid (33.2 mg, 0.20 mmol) were mixed in 8 ml deionized water and the pH value was adjusted to about 5 with 40% NaOH. Then, the mixture was sealed into a Teflon-lined stainless steel autoclave and heated at 120 °C for 48 h under autogenous pressure. After the mixture was slowly cooled to room temperature at a rate of 5 °C h−1, the pale-yellow block crystals of 5 were obtained by filtration and washed with water with a yield of 55%. Anal. calcd for C52H46N4O21Sm2: C 45.80; H 3.40; N 4.11; found: C 45.66; H 3.22; N 4.02%. IR (KBr pellet, cm−1): 3436 vs, 1613 s, 1587 m, 1546 s, 1508 s, 1475 w, 1419 s, 1343 w, 1314 m, 1260 m, 1179 w, 1109 m, 1021 w, 884 w, 827 m, 791 w, 751 m.
Synthesis of [Sm(QOA)(BDC)] (6).
A mixture of Sm(NO3)3·6H2O (88.9 mg, 0.20 mmol), HQOA (121.9 mg, 0.60 mmol), H2BDC (66.5 mg, 0.40 mmol) and 40% NaOH in 8 ml deionized water was placed into a Teflon-lined stainless steel reactor and heated at 130 °C for 24 h under autogenous pressure. After the mixture was slowly cooled to room temperature at a rate of 5 °C h−1, the pale-yellow block crystals of 6 were obtained by filtration and washed with water and ethanol in 59% yield. Anal. calcd for C19H12NO7Sm: C 44.17; H 2.34; N 2.71; found: C 44.35; H 2.52; N 2.92%. IR (KBr pellet, cm−1): 1614 s, 1580 m, 1506 m, 1423 m, 1396 m, 1315 m, 1260 m, 1183 w, 1109 m, 826 m, 791 m, 760 s.
Synthesis of [Eu(QOA)(BDC)] (7).
7 was obtained by a similar method used for 6 except that Sm(NO3)3 was replaced by Eu(NO3)3 (89.2 mg, 0.20 mmol). The pink block crystals of 7 were obtained in 53% yield. Anal calcd for C19H12EuNO7: C 44.03; H 2.33; N 2.70; found: C 44.17; H 2.48; N 2.78%. IR (KBr pellet, cm−1): 1628 vs, 1593 s, 1508 m, 1418 w, 1393 s, 1287 s, 1254 m, 1177 m, 1136 m, 1109 s, 1076 m, 1018 w, 945 m, 885 m, 829 w, 781 w, 746 m.
Diffraction data of compounds 1–7 were collected on a Bruker Apex II Smart CCD diffractometer equipped with a graphite monochromated Mo Kα radiation (λ = 0.71073 Å) with a ω-scan technique at 298(2) K. The data were integrated by using the SAINT program, which was also used for the intensity corrections for the Lorentz and polarization effects. Multi-scan absorption correction was applied with the SADABS program.12 The structures were solved by direct methods using the program SHELXS-97 and all the non-hydrogen atoms were refined anisotropically on F2 with the full-matrix least-squares technique using the SHELXL-97 program.13 The hydrogen atoms of the water molecules were located in the difference Fourier maps and the other hydrogen atoms were placed in calculated positions and refined as riding atoms with isotropic thermal factors. The details of the crystal parameters, data collections and refinement for 1–7 are given in Table 1 and selected bond lengths with the estimated standard deviations are listed in Table 2.
Complex |
1
|
2
|
3
|
4
|
5
|
6
|
7
|
R
1 = ∑ ||Fo| − |Fc||/∑ |Fo|.
wR
2 = {∑ [w(Fo2 − Fc2]2/∑ [w(Fo2)]2}1/2.
|
Chemical formula |
C22H22EuN3O12 |
C22H22GdN3O12 |
C46H40N4O20Pr2 |
C46H40Er2N4O20 |
C52H46N4O21Sm2 |
C19H12NO7Sm |
C19H12EuNO7 |
Formula weight |
672.39 |
677.68 |
1250.64 |
1303.34 |
1363.63 |
516.65 |
518.26 |
Crystal system |
Monoclinic |
Monoclinic |
Monoclinic |
Monoclinic |
Monoclinic |
Monoclinic |
Monoclinic |
Space group |
P 21/c |
P 21/c |
P 21/c |
P 21/c |
P 2/c |
P 21/c |
P 21/c |
a/Å |
18.724(4) |
18.7397(7) |
19.268(4) |
19.266(4) |
12.3342(3) |
10.8836(2) |
10.8671(3) |
b/Å |
15.086(3) |
15.1331(6) |
16.070(4) |
16.039(3) |
11.3752(3) |
10.9362(2) |
10.8974(3) |
c/Å |
8.6362(17) |
8.6165(3) |
15.592(3) |
15.551(3) |
19.0229(6) |
15.8323(2) |
15.8338(4) |
β/° |
93.97(3) |
93.999(2) |
100.38(3) |
100.41(3) |
103.810(2) |
117.444(1) |
117.385(2) |
V/Å3 |
2433.6(9) |
2437.60(16) |
4748.8(16) |
4726.3(16) |
2591.83(12) |
1672.37(5) |
1664.96(8) |
Z
|
4 |
4 |
4 |
4 |
2 |
4 |
4 |
D
c/g cm−3 |
1.835 |
1.847 |
1.749 |
1.832 |
1.747 |
2.052 |
2.068 |
µ/mm−1 |
2.649 |
2.792 |
2.113 |
3.612 |
2.329 |
3.557 |
3.813 |
F(000) |
1336 |
1340 |
2488 |
2560 |
1356 |
1004 |
1008 |
Refl. measured |
21076 |
21452 |
50762 |
41762 |
19266 |
15948 |
11917 |
Unique refl. (Rint) |
4396 (0.0376) |
4398 (0.0990) |
8595 (0.0818) |
8506(0.0876) |
5081 (0.0537) |
3028 (0.0238) |
3011 (0.0269) |
GOF on F2 |
1.028 |
1.079 |
1.016 |
1.054 |
1.026 |
1.030 |
1.010 |
R
1 [I > 2σ(I) ]a |
0.0336 |
0.0490 |
0.0538 |
0.0596 |
0.0332 |
0.0198 |
0.0196 |
wR
2 [I > 2σ(I) ]b |
0.0908 |
0.0864 |
0.1215 |
0.1513 |
0.0738 |
0.0455 |
0.0555 |
Table 2 Selected bonds lengths (Å) for 1–7a
Symmetry transformations used to generate equivalent atoms: #1 x, 1/2 − y, −1/2 + z; #2 x, 3/2 − y, −1/2 + z; # 3 x, 3/2 − y, −1/2 + z; # 4 −x, y, 1/2 − z; #5 x, 1 + y, z; #6 1 − x, y, 1/2 − z; #7 1 − x, 1 + y, 1/2 − z; #8 − x, 1/2 + y, 1/2 − z; #9 1 − x, 1/2 + y, 1/2 − z.
|
[Eu(QOA)2(H2O)2]·NO3·H2O (1) |
Eu(1)–N(1) |
2.597(4) |
Eu(1)–N(2) |
2.586(5) |
Eu(1)–O(2) |
2.377(4) |
Eu(1)–O(1) |
2.612(4) |
Eu(1)–O(3) #1 |
2.297(4) |
Eu(1)–O(4) |
2.631(4) |
Eu(1)–O(5) |
2.343(4) |
Eu(1)–O(2w) |
2.415(4) |
Eu(1)–O(3w) |
2.461(4) |
[Gd(QOA)2(H2O)2]·NO3·H2O (2) |
Gd(1)–O(3)#1 |
2.281(6) |
Gd(1)–O(2w) |
2.435(6) |
Gd(1)–N(2) |
2.590(7) |
Gd(1)–O(5) |
2.334(5) |
Gd(1)–O(3w) |
2.457(5) |
Gd(1)–O(1) |
2.595(5) |
Gd(1)–O(2) |
2.357(5) |
Gd(1)–N(1) |
2.581(7) |
Gd(1)–O(4) |
2.627(5) |
[Pr2(QOA)4(ox)(H2O)]·3H2O (3) |
Pr(1)–N(1) |
2.638(6) |
Pr(1)–N(2) |
2.691(6) |
Pr(1)–O(1) |
2.633(5) |
Pr(1)–O(2) |
2.454(5) |
Pr(1)–O(4) |
2.619(6) |
Pr(1)–O(5) |
2.378(6) |
Pr(1)–O(13) |
2.532(6) |
Pr(1)–O(14) |
2.461(6) |
Pr(1)–O(1w) |
2.449(5) |
Pr(2)–N(3) |
2.645(7) |
Pr(2)–N(4) |
2.684(6) |
Pr(2)–O(3) |
2.447(6) |
Pr(2)–O(7) |
2.662(6) |
Pr(2)–O(8) |
2.403(6) |
Pr(2)–O(10) |
2.629(6) |
Pr(2)–O(11) |
2.371(6) |
Pr(2)–O(15)#2 |
2.542(6) |
Pr(2)–O(16)#2 |
2.458(6) |
[Er2(QOA)4(ox)(H2O)]·3H2O (4) |
Er(1)–N(1) |
2.633(8) |
Er(1)–N(2) |
2.680(8) |
Er(1)–O(1) |
2.622(6) |
Er(1)–O(2) |
2.431(7) |
Er(1)–O(4) |
2.602(6) |
Er(1)–O(5) |
2.385(7) |
Er(1)–O(1w) |
2.426(7) |
Er(1)–O(15) |
2.516(7) |
Er(1)–O(16) |
2.538(7) |
Er(2)–N(3) |
2.633(9) |
Er(2)–N(4) |
2.677(8) |
Er(2)–O(7) |
2.643(7) |
Er(2)–O(8) |
2.405(7) |
Er(2)–O(10) |
2.596(6) |
Er(2)–O(11) |
2.363(7) |
Er(2)–O(3) |
2.453(7) |
Er(2)–O(13)#3 |
2.544(7) |
Er(2)–O(14)#3 |
2.456(7) |
[Sm2(QOA)4 (BDC)(H2O)]·4H2O (5) |
Sm(1)–N(1) |
2.595(4) |
Sm(1)–O(1) |
2.608(4) |
Sm(1)–O(2) |
2.384(4) |
Sm(1)–O(6) |
2.317(4) |
Sm(1)–O(1w) |
2.743(5) |
Sm(1)–O(6)#4 |
2.317(4) |
Sm(1)–O(2)#4 |
2.384(4) |
Sm(1)–N(1)#4 |
2.595(4) |
Sm(1)–O(1)#4 |
2.608(4) |
Sm(2)–N(2) |
2.677(4) |
Sm(2)–O(4) |
2.684(4) |
Sm(2)–O(5) |
2.401(3) |
Sm(2)–O(7) |
2.450(2) |
Sm(2)–O(8)#5 |
2.480(3) |
Sm(2)–O(7)#6 |
2.450(2) |
Sm(2)–O(5)#6 |
2.401(3) |
Sm(2)–N(2)#6 |
2.677(4) |
Sm(2)–O(4)#6 |
2.684(4) |
Sm(2)–O(8)#7 |
2.480(3) |
|
|
|
|
[Sm(QOA)(BDC)] (6) |
Sm(1)–O(5) |
2.308(2) |
Sm(1)–O(7) |
2.350(2) |
Sm(1)–O(6) |
2.373(2) |
Sm(1)–O(3)#8 |
2.414(2) |
Sm(1)–O(4) |
2.451(2) |
Sm(1)–O(2) |
2.475(2) |
Sm(1)–O(1) |
2.589(2) |
Sm(1)–N(1) |
2.619(3) |
|
|
[Eu(QOA)(BDC)] (7) |
Eu(1)–O(1) |
2.575(3) |
Eu(1)–O(2) |
2.465(3) |
Eu(1)–O(3)#9 |
2.408(3) |
Eu(1)–O(4) |
2.360(3) |
Eu(1)–O(5) |
2.331(3) |
Eu(1)–O(6) |
2.301(3) |
Eu(1)–O(7) |
2.442(3) |
Eu(1)–N(1) |
2.601(3) |
|
|
Results and discussion
All the compounds 1–7 have been successfully synthesized under hydrothermal conditions. These crystalline solids are stable in air and insoluble in water or common organic solvent such as ethanol and acetone. Scheme 1 summarized the coordination modes of QOA and BDC anions in 1–7.
 |
| Scheme 1 Coordination modes of the organic ligands in 1–7 | |
The IR spectra of these compounds show features attributable to the carboxylate stretching vibrations. The characteristic bands of carboxylate groups are shown in the range 1508–1659 cm−1 for asymmetric stretching and 1379–1475 cm−1 for symmetric stretching.14 The absence of strong absorption peaks around 1700 cm−1 indicates that all carboxyl groups (–COOH) of the organic compounds are deprotonated. For 1 and 2, the strong signals at near 1383 cm−1 can be assigned to the stretching vibrations of free NO3− anions.14 Moreover, the strong and broad absorption bands observed at around 3366∼3448 cm−1 are probably assigned to the νO–H vibrations of water molecules in compounds 1–5.
1D chains of 1–4
Single crystal X-ray diffraction analyses reveal that 1–2 and 3–4 are two pairs of isomorphous compounds, so 1 and 3 are selected as the representative examples to be described in detail.
Complex 1 exhibits a 1D infinite zigzag chain-like coordination polymer consisting of [Eu(QOA)2] building blocks, crystallizing in space group P21/c. The asymmetric unit consists of one europium atom, two QOA ligands, one free nitrate anion, two coordinated and one lattice water molecules. As illustrated in Fig. 1, the europium atom is nine-coordinated in a N2O7 donor set with the coordination geometry of a distorted tri-capped trigonal prism by two quinolyl nitrogen atoms, four oxygen atoms from two chelating QOA ligands, one carboxylate oxygen atom from another bridging QOA ligand and two oxygen atoms from two coordinated water molecules. The Eu–O bond distances are in the range from 2.297(4) to 2.631(4) Å, and the Eu–N distances are 2.586(5) and 2.597(4) Å, respectively, which are similar to the reported Eu–O and Eu–N bond distances.6a,8a,15 The average distance of Eu–O (carboxylate oxygen) is 2.339 Å, which is significantly shorter than those of Eu–N (2.592 Å) and Eu–O (ether oxygen, 2.622 Å), indicating that the coordination ability of the carboxylate oxygen atom is the strongest among three donor atoms from the QOA molecule. The QOA ligand adopts two kinds of coordination modes in 1, such as tridentate-chelating (Scheme 1a) and tridentate-chelating bridging (Scheme 1b). Further investigation of the crystal structure of 1 reveals that the [Eu(QOA)2] units are connected to each other with Eu⋯Eu separation of 6.675 Å through the bridging QOA ligands leading to a 1D infinite zigzag chain-like structure parallel to the c–axis (Fig. 2c and d). In addition, there are O–H⋯O hydrogen bonds in 1, which include the following contacts: (a) between coordinated water molecule and carboxylate oxygen atoms of the QOA ligands [O⋯O, 2.701(6) Å], (b) between a coordinated water molecule and a nitrate ion [O⋯O, 2.748(8)∼3.042(13) Å] and (c) between coordinated water and lattice water molecules [O⋯O, 2.716(8) Å] (Table S1†). Thus, these adjacent 1D polymeric chains are linked together by these hydrogen bonds, forming an extended 2D layer-like structure, as depicted in Fig. 2e. Moreover, these layers stack together via π⋯π packing attractions [centroid–centroid distances = 3.660∼3.978 Å], resulting in formation of a 3D supramolecular network (Fig. S1†).
 |
| Fig. 1 ORTEP view of 1 with 30% thermal ellipsoids. All hydrogen atoms are omitted for clarity. | |
![(a) Ball and stick and (b) polyhedral representation of lanthanide building block [Ln(QOA)2] in 1. (c) Structural and (d) schematic view of a 1D zigzag chain in 1. (e) View of a 2D layer-like network in 1 assembled by the hydrogen bonds.](/image/article/2010/CE/b910219a/b910219a-f2.gif) |
| Fig. 2 (a) Ball and stick and (b) polyhedral representation of lanthanide building block [Ln(QOA)2] in 1. (c) Structural and (d) schematic view of a 1D zigzag chain in 1. (e) View of a 2D layer-like network in 1 assembled by the hydrogen bonds. | |
The X-ray structural analysis of 3 indicates that each asymmetric unit contains two nine-coordinated praseodymium atoms, four QOA ligands, one oxalate anion, one coordinated and three lattice water molecules (Fig. 3). Both Pr atoms exhibit distorted tricapped trigonal prism coordination geometries with different coordination environments. The Pr1 atom coordinates to two tridentate chelating QOA anions, one water molecules as well as one bridging oxalate anion. Unlike Pr1 atom, Pr2 atom is coordinated with six donor atoms from two tridentate QOA anions, one bridging carboxylate oxygen atom from an adjacent QOA ligand and two carboxylate oxygen atoms from one bridging oxalate anion. The Pr–O and Pr–N bond distances are in the range of 2.371(6)–2.662(6) Å and 2.638(6)–2.691(6) Å, respectively, all of which are comparable to those reported for the Pr(III) complexes.7b,16 In each asymmetric unit, Pr(1) and Pr(2) atoms are linked by the carboxylate-bridge from the QOA anion to form dinuclear praseodymium building blocks [Pr2(QOA)4], with a Pr⋯Pr separation of 6.936 Å (Fig. 4a). Then, these adjacent dinuclear blocks [Pr2(QOA)4] are connected together through bis-bidentate oxalate anions, resulting in the formation of a 1D infinite folded chain-like structure parallel to the c-axis, as depicted in Fig. 4c. The lattice water molecules occupies in the space of the two neighboring chains, in addition, there are O–H⋯O hydrogen bonds between water molecules and carboxylate-oxygen atoms from the QOA ligand [O⋯O, 2.711(8)∼2.993(10) Å] (Table S1†). Thus, these adjacent 1D folded chains are connected by these hydrogen bonds to form a 2D layer-like network (Fig. 4e). Moreover, the adjacent quinolyl rings are involved in π–π packing attractions by partial overlapping of π–electron densities and the centroid–centroid separations between two aromatic rings are in the range from 3.553 to 3.955 Å. These π–π stacking interactions lead to the formation of a 3D supramolecular network (Fig. S2†).
 |
| Fig. 3 ORTEP view of 3 with 30% thermal ellipsoids. All hydrogen atoms are omitted for clarity. | |
![(a) Ball and stick and (b) polyhedral representation of a lanthanide building block [Ln2(QOA)4] in 3. (c) Structural and (d) schematic view of a 1-D folded chain in 3. (e) View of the 2D layer in 3 assembled by the hydrogen bonds.](/image/article/2010/CE/b910219a/b910219a-f4.gif) |
| Fig. 4 (a) Ball and stick and (b) polyhedral representation of a lanthanide building block [Ln2(QOA)4] in 3. (c) Structural and (d) schematic view of a 1-D folded chain in 3. (e) View of the 2D layer in 3 assembled by the hydrogen bonds. | |
The structure of 2 is similar to that of 1 and compound 4 is the same structure as 3. Selected bond lengths are listed in Table 2. As the ionic radii decreasing from Pr to Er atom, the average Ln–N distance (Ln = Pr: 2.665 Å; Er: 2.656 Å) decrease due to the lanthanide contraction effect. Similar changes are also observed in the average Ln–O bond length and the Ln⋯Ln separations (Pr⋯Pr: 6.382 and 6.936 Å; Er⋯Er: 6.356 and 6.914 Å).
2D sheet of 5
X-Ray crystallography reveals that complex 5 crystallizes in a monoclinic fashion with space group P2/c. As illustrated in Fig. 5, each asymmetric unit comprises two types of coordination environments of Sm atoms. The Sm1 atom is nine-coordinated and the polyhedron consists of six donor atoms from two tridentate QOA anions, two carboxylate oxygen atoms from two adjacent bridging QOA anions and one water molecule, and it is a distorted tricapped trigonal prism. The Sm2 atom is ten-coordinated by six donor atoms from two tridentate QOA anions and four carboxylate oxygen atoms from two BDC anions, and the geometry of the coordination polyhedron can be best described as a distorted bicapped square antiprism. The Sm1 atom lies on a twofold axis. The Sm–O and Sm–N bond distances are in the range of 2.317(4)∼2.743(5) Å and 2.595(4)∼2.677(4) Å, respectively, all of which are within the normal range.7d,17
 |
| Fig. 5 ORTEP view of 5 with 30% thermal ellipsoids. All hydrogen atoms are omitted for clarity. | |
Further investigation on the crystal structure of 5 indicates that the [Sm(QOA)2] units are firstly connected to each other with a short separation of Sm1⋯Sm2 (6.772 Å) through bridging QOA anions, resulting in a 1D zigzag chain-like structure parallel to the a-axis, which is similar to that of compound 1; furthermore, these adjacent 1D chains [Sm(QOA)2]n are linked together through bis-bidentate carboxylate bridges from the BDC anions (Scheme 1c) (Sm⋯Sm distance, 11.375 Å) to generate an ordered (2,4)-connected 2D sheet-like framework (Fig. 6). Lattice water molecules occupy the space of the 2D grids. Moreover, these 2D sheets are connected into a 3D supramolecular network (Fig. S3†) via the hydrogen bonds (Table S1†) and π–π stacking interactions between the adjacent quinolyl rings [centroid–centroid distances, 3.741∼3.995 Å].
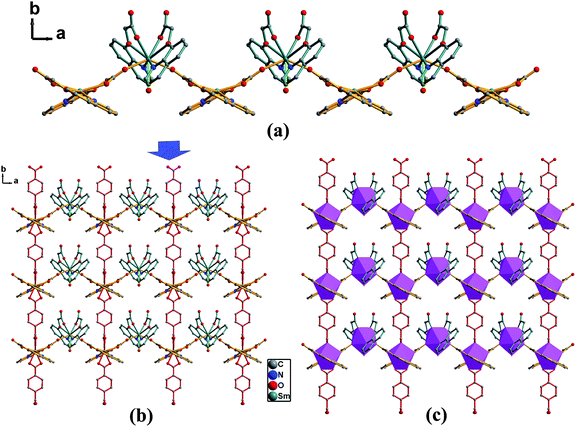 |
| Fig. 6 (a) Structural view of a 1D zigzag chain in 5. (b) Structural view and (c) polyhedral representation of a (2,4)-connected 2D sheet in 5. Uncoordinated water and all hydrogen atoms are omitted for clarity. | |
3D frameworks of 6 and 7
Compounds 6 and 7 are isomorphous, so complex 7 is selected as a representation to be discussed in detail. Complex 7 exhibits an 8-connected 3D framework, crystallizing in monoclinic space group P 21/c. Each asymmetric unit consists of one eight-coordinated europium atom, one QOA ligand and two half BDC ligands, each one lying on an inversion centre. The structure is significantly different from those of 1–5. As illustrated in Fig. 7, each Eu atom coordinates to three donor atoms from one QOA anion, one bridging carboxylate oxygen atom from one adjacent QOA anion and four oxygen atoms from four BDC anions: eight donor atom forms a distorted dodecahedron configuration (Fig. 8). The distances of Eu–O bonds range from 2.301(3) to 2.575(3) Å, similar to those found in the reported Eu(III) complexes.6a, 8a, 15 Two crystallographically equivalent Eu atoms are bridged by four carboxylate groups of four BDC anions in di-monodentate fashion (Scheme 1d) to give a dinuclear europium building block [Eu2(QOA)2(RCO2)4] with the Eu⋯Eu separation of 4.456 Å (Fig. 8a). Then, these dinuclear units are interconnected through the phenyl groups of BDC and QOA anions to generate an extended 3D infinite framework (Fig. 8d). The topological analysis approach was employed to better describe the structure characteristic of 7. The structure of 7 can be described as an 8-connected 3D framework (Fig. S4†): each building unit is connected to eight adjacent building blocks through four phenyl groups and four quinolyl groups. Moreover, π–π stacking interactions between two adjacent quinolyl rings are observed with a centroid–centroid separation of 3.487 Å, which also contributes to the stability of this 3D structure.
 |
| Fig. 7 ORTEP view of 7 with 30% thermal ellipsoids. All hydrogen atoms are omitted for clarity. | |
![(a) Ball and stick and (b) polyhedral representation of the dinuclear building block [Eu2(QOA)2(RCO2)4] in 7. (c) View of a 2D layer structure and (d) 3D framework in 7; all quinolyl rings from the QOA ligands are simplified for clarity.](/image/article/2010/CE/b910219a/b910219a-f8.gif) |
| Fig. 8 (a) Ball and stick and (b) polyhedral representation of the dinuclear building block [Eu2(QOA)2(RCO2)4] in 7. (c) View of a 2D layer structure and (d) 3D framework in 7; all quinolyl rings from the QOA ligands are simplified for clarity. | |
Structural comparison of the frameworks 1–7
For seven lanthanide complexes reported in this work, X-ray diffraction studies reveal that they can be classified into four kinds of typical structure features: 1D zigzag chains for 1–2; 1D folded chains for 3–4; an ordered (2,4)-connected 2D sheet for 5, and 8-connected 3D frameworks for 6–7. As shown in Scheme 2, it is clearly demonstrated that choosing suitable bridging organic ligands is crucial for the formation of 1–7. For 1–2, no additional bridging ligands are present. The QOA molecule acts as a bridge connecting the adjacent coordination units [Ln(QOA)2], resulting in the formation of 1D zigzag chains. In 3–4, QOA molecules and oxalate anions alternately serve as the bridges between the adjacent lanthanide ions. Due to the short and rigid skeleton structure for oxalate anions, the alternate connection between QOA and ox molecules seems to be in favor of forming a 1D folded chain-like structure. For compound 5, the BDC molecule adopts bis-bidentate chelating coordination mode as the linker to connect two Sm atoms that may be at unsaturated coordination state in two adjacent chains parallel to the b-axial direction, forming a (2,4)-connected 2D sheet structure. The frameworks 6–7 are composed of the dinuclear building blocks [Ln2(QOA)2(RCO2)4], in which the BDC molecule adopts another coordination mode (Scheme 1d) to link the adjacent Ln(III) anions to a great extent. Finally, the 8-connected 3D frameworks are constructed through the connectivity of carboxylate bridges.
 |
| Scheme 2 | |
The progressive variation from the 1D zigzag chains (1–2) to 1D folded chains (3–4) to 2D network (5) and to 3D frameworks (6–7) is mainly attributed to the change of bridging ligands as well as the different coordination modes of organic ligands. In other words, the structural diversity and the dimensional change of these Ln-QOA complexes are effectively controlled by choosing suitable bridging organic ligands.
Thermal behaviors
Owing to the similarity of the structures for 1–2 and 3–4, compounds 1, 3 and 5 were selected for thermogravimetric analysis (TGA) to examine the thermal stability. TGA curves have been obtained in N2 atmosphere for crystalline samples of 1, 3 and 5 in the temperature range 25–800 °C (Fig. S5†). All TGA results display two obvious steps of weight loss.
For 1, the first weight loss of 3.05% from 30 to 160 °C corresponds to the removal of the uncoordinated water molecule (calcd 2.68%) in the asymmetric unit. The second weight loss above 250 °C is attributable to decomposition of the whole coordination network. The TGA study of 3 shows that the first weight loss of 6.60% in the range of 30–160 °C is ascribed to the loss of three uncoordinated water molecules (calcd 5.76%). A major weight loss occurred in the next step, which may correspond to the collapse of the coordination structure. Compound 5 shows similar thermal behavior. Four lattice water molecules were gradually lost in the temperature range of 30–220 °C (calcd/found: 5.27/4.67%). The decomposition of the coordination framework occurred immediately when the temperature was above 300 °C. Compared to their decomposition stages, the results reveal that the decomposition temperature gradually increases with progressive structural variation from the 1D chains (1–4) to a 2D sheet-like network (5) and their thermal stabilities also gradually rise.
Photoluminescent properties
Taking into account the excellent luminescent properties of the Eu(III) ions, the luminescence of 1 and 7 at the solid state was investigated at room temperature. Their emission spectra (Fig. 9) at the excited wavelength of 340 nm exhibit the characteristic transition of 5D0 → 7FJ (J = 0–4) of Eu(III) ions in the range of 570–705 nm, implying the ligand-to-europium energy transfer is efficient under the experimental conditions.18 The appearance of the symmetry-forbidden emission 5D0 → 7F0 at 580 nm indicates that the Eu(III) ions occupy sites with low symmetry in the crystals, which is in agreement with the single-crystal analyses. The emission spectrum is dominated by the characteristic 5D0 → 7F2 transition at about 619 nm which is more intense than the 5D0 → 7F1 transition at about 592 nm, and the intensity ratios I (5D0 → 7F2)/I (5D0 → 7F1) are equal to 1.75 for 1 and 1.60 for 7, respectively, which also suggests the non-centrosymmetric coordination environment of the Eu(III) ions.19 Therefore, the solid samples 1 and 7 exhibit strong red emission under UV light excitation.
Compared with their emission spectra, the transition intensity changes in the order of 7 > 1 under the same measurement conditions, which means that the coordination structure of the compound has an obvious effect on the emission behavior of the Eu(III) ion. For a 1D zigzag chain of 1, the QOA ligands don't completely encapsulate the Eu(III) ions, leaving two sites ligated with water, whilst some lattice water molecules occupy the space of the two neighboring chains. For a 3D framework of 7, the lanthanide ions are encapsulated by the QOA and BDC anions. Earlier results have revealed that a weak vibronic coupling between the lanthanides and OH oscillators of water molecules provides a facile path for radiationless de-excitation of the metal ions.20
Conclusion
In summary, seven new lanthanide coordination polymers based on (8-quinolyloxy)-acetate (QOA), namely, [Ln(QOA)2(H2O)2]·NO3·H2O [Ln = Eu (1), Gd (2)], [Ln2(QOA)4(ox) (H2O)]·3H2O [Ln = Pr(3), Er(4)], [Ln2(QOA)4(BDC)(H2O)]·4H2O [Ln = Sm (5)] and [Ln(QOA)(BDC)] [Ln = Sm(6), Eu(7)] (ox = oxalate; BDC = 1, 4 –benzenedicarboxylate) have been synthesized under hydrothermal conditions. X-Ray crystallography reveals that the choice of different organic bridging ligands could promote different topological structures. These coordination polymers have 1D zigzag chains (1–2) and 1D folded chains (3–4) and an ordered (2,4)-connected 2D sheet-like structure (5) as well as 8-connected 3D frameworks (6–7). Compounds 1–5 are further connected into 3D supramolecular networks via hydrogen bonds and π–π stacking interactions. Moreover, complexes 1 and 7 in the solid state exhibit characteristic red emission of Eu(III) ions corresponding to electronic transitions from the excited state 5D0 to the multiplets 7FJ (J = 0–4) at room temperature.
Acknowledgements
This work was supported by the National Natural Science Foundation of China (No. 20771040).
References
-
(a) L. Ma, O. R. Evans, B. M. Foxma and W. B. Lin, Inorg. Chem., 1999, 38, 5837 CrossRef CAS;
(b) C. M. G. dos Santos, A. J. Harte, S. J. Quinn and T. Gunnlaugsson, Coord. Chem. Rev., 2008, 252, 2512 CrossRef CAS;
(c) G. L. Law, K. L. Wong, K. K. Lau, H. L. Tam, K. W. Cheah and W. T. Wong, Eur. J. Inorg. Chem., 2007, 5419 CrossRef CAS;
(d) P. Wang, J. P. Ma, Y. B. Dong and R. Q. Huang, J. Am. Chem. Soc., 2007, 129, 10620 CrossRef CAS.
-
(a) A. Bencini, C. Benelli, A. Caneschi, R. L. Carlin, A. Dei and D. Gatteschi, J. Am. Chem. Soc., 1985, 107, 8128 CrossRef CAS;
(b) Z. Wang, C. M. Jin, T. Shao, Y. Z. Li, K. L. Zhang, H. T. Zhang and X. Z. You, Inorg. Chem. Commun., 2002, 5, 642 CrossRef CAS;
(c) Y. Huang, B. Yan and M. Shao, J. Solid State Chem., 2008, 181, 2935 CrossRef CAS;
(d) Y. C. Liang, R. Cao, W. P. Su, M. C. Hong and W. J. Zhang, Angew. Chem., Int. Ed., 2000, 39, 3304 CrossRef CAS.
-
(a) P. Lebduskova, P. Hermann, L. Helm, E. Toth, J. Kotek, K. Binnemans, J. Rudovsky, I. Lukes and A. E. Merbach, Dalton Trans., 2007, 493 RSC;
(b) B. Moulton and M. J. Zaworotko, Chem. Rev., 2001, 101, 1629 CrossRef CAS;
(c) X. Y. Chen, Y. Bretonnière, J. Pécaut, D. Imbert, J. C. G. Bünzli and M. Mazzanti, Inorg. Chem., 2007, 46, 625 CrossRef CAS;
(d) A. J. Harte, P. Jensen, S. E. Plush, P. E. Kruger and T. Gunnlaugsson, Inorg. Chem., 2006, 45, 9465 CrossRef CAS.
-
(a) M. Shibasaki and N. Yoshikawa, Chem. Rev., 2002, 102, 2187 CrossRef CAS;
(b) J. Inanaga, H. Furuno and T. Hayano, Chem. Rev., 2002, 102, 2211 CrossRef CAS.
- B. Zhao, P. Cheng, X. Y. Chen, C. Cheng, W. Shi, D. Z. Liao, S. P. Yan and Z. H. Jiang, J. Am. Chem. Soc., 2004, 126, 3012 CrossRef CAS.
-
(a) M. S. Liu, Q. Y. Yu, Y. P. Cai, C. Y. Su, X. M. Lin, X. X. Zhou and J. W. Cai, Cryst. Growth Des., 2008, 8, 4083 CrossRef CAS;
(b) X. F. Li, Z. B. Han, X. N. Chen and X. M. Chen, Inorg. Chem. Commun., 2006, 9, 1091 CrossRef CAS;
(c) Q. Y. Liu and L. Xu, Eur. J. Inorg. Chem., 2005, 3458 CrossRef CAS.
-
(a) Z. He, E. Q. Gao, Z. M. Wang, C. H. Yan and M. Kurmoo, Inorg. Chem., 2005, 44, 862 CrossRef CAS;
(b) J. W. Ye, J. Y. Zhang, G. L. Ning, G. Tian, Y. Chen and Y. Wang, Cryst. Growth Des., 2008, 8, 3098 CrossRef CAS;
(c) C. B. Liu, H. L. Wen, S. S. Tan and X. G. Yi, J. Mol. Struct., 2008, 879, 25 CrossRef CAS;
(d) C. Qin, X. L. Wang, E. B. Wang and L. Xu, Inorg. Chim. Acta, 2006, 359, 417 CrossRef CAS.
-
(a) L. Cañadillas-Delgado, O. Fabelo, J. Pasán, F. S. Delgado, M. Déniz, E. Sepúlveda, M. M. Laz, M. Julve and C. Ruiz-Pérez, Cryst. Growth Des., 2008, 8, 1313 CrossRef CAS;
(b) Y. Q. Sun, J. Zhang, Y. M. Chen and G. Y. Yang, Angew. Chem., Int. Ed., 2005, 44, 5814 CrossRef CAS;
(c) S. F. Tang, J. L. Song, X. L. Li and J. G. Mao, Cryst. Growth Des., 2006, 6, 2322 CrossRef CAS;
(d) B. Q. Ma, S. Gao, G. Su and G. X. Xu, Angew. Chem., Int. Ed., 2001, 40, 434 CrossRef CAS.
-
(a) R. J. Hill, D. L. Long, N. R. Champness, P. Hubberstey and M. Schröder, Acc. Chem. Res., 2005, 38, 335 CrossRef CAS;
(b) D. L. Long, A. J. Blake, N. R. Champness, C. Wilson and M. Schröder, Angew. Chem., Int. Ed., 2001, 40, 2444 CAS.
-
(a) Y. H. Wang, L. P. Zhang, L. P. Jin, S. Gao and S. Z. Lu, Inorg. Chem., 2003, 42, 4985 CrossRef CAS;
(b) X. J. Zhang, Y. H. Xing, J. Han, M. F. Ge and S. Y. Niu, Z. Anorg. Allg. Chem., 2008, 634, 1765 CrossRef CAS;
(c) X. J. Gu and D. F. Xue, Cryst. Growth Des., 2007, 7, 1726 CrossRef CAS;
(d) H. Deng, Y. H. Li, Y. C. Qiu, Z. H. Liu and M. Zeller, Inorg. Chem. Commun., 2008, 11, 1151 CrossRef CAS.
-
(a) X. N. Cheng, W. X. Zhang and X. M. Chen, J. Am. Chem. Soc., 2007, 129, 15738 CrossRef CAS;
(b) Z. H. Wang, J. Fan, W. G. Zhang and J. Wang, Acta Crystallogr., Sect. E: Struct. Rep. Online, 2008, 64, m1446 CrossRef;
(c) Y. H. Wang, R. F. Song and F. Y. Zhang, J. Mol. Struct., 2005, 752, 104 CrossRef CAS;
(d) Y. H. Wang and F. Lu, Acta Crystallogr., Sect. C: Cryst. Struct. Commun., 2004, 60, m557 CrossRef.
-
Bruker, SMART and SAINT; Bruker AXS Inc.: Madison, Wisconsin, 2004 Search PubMed.
-
(a)
G. M. Sheldrick, SHELXS-97, Program for Crystal Structure Solution, University of Göttingen, Göttingen, Germany, 1997 Search PubMed;
(b)
G. M. Sheldrick, SHELXL-97, Program for Crystal Structure Refinement, University of Göttingen, Göttingen, Germany, 1997 Search PubMed.
-
K. Nakamoto, Infrared and Raman Spectra of Inorganic and Coordination Compounds, 5th edn, John Wiley & Sons, New York, 1997 Search PubMed.
- F. Kirby, D. Foster and F. S. Richardson, Chem. Phys. Lett., 1983, 95, 507 CrossRef CAS.
- P. Ren, W. Shi and P. Cheng, Inorg. Chem. Commun., 2008, 11, 125 CrossRef CAS.
- H. Y. Zhang, J. J. Zhang, N. Ren, S. L. Xu, L. Tian and J. H. Bai, J. Alloys Compd., 2008, 464, 277 CrossRef CAS.
- Betterncourt Dias and S. Viswanathan, Chem. Commun., 2004, 1024 RSC.
-
J. C. G. Bünzli, In Lanthanide Probes in Life, Chemical, and Earth Sciences. Theory and Practice, J. C. G. Bünzli and G. R. Choppin, Eds., Elsevier Scientific Publishers, Amsterdam, 1989, Chapter 7 Search PubMed.
- M. Latva, H. Takalo, V.-M. Mukkala, C. Matachescu, J. C. Rodríguez-Ubis and J. Kankare, J. Lumin., 1997, 75, 149 CrossRef CAS.
Footnote |
† Electronic supplementary information (ESI) available: Additional structural figures, TGA curves, the tables of hydrogen bonds and selected bond angles and X-ray crystallographic information files of 1–7. CCDC reference numbers 717618–717624. For ESI and crystallographic data in CIF or other electronic format see DOI: 10.1039/b910219a |
|
This journal is © The Royal Society of Chemistry 2010 |