DOI:
10.1039/C0AY00226G
(Paper)
Anal. Methods, 2010,
2, 1008-1012
Received
6th April 2010
, Accepted 9th May 2010
First published on
10th June 2010
1. Introduction
The oligonucleotide microarray technology is a kind of high throughput analytical method which has been widely used to study the gene expression,1–3 methylation,4 and protein-DNA interactions,5 as well as large scale single nucleotide polymorphism (SNPs) genotyping.6,7 The assay's sensitivity and selectivity are two essential aspects in oligonucleotide array applications.8 Therefore, an ideal substrate with high binding capacity has to be readily achieved, most importantly, the substrate must not bind non-specifically to anything else present in the system under investigation and generate excellent signal-to-noise ratio. Up to now, substrates developed for DNA arrays mainly fall into two classes: (1) 2D planar slides with a uniform layer of linear functional groups including aldehyde, epoxy, or carboxylic esters;9–11 and (2) planar solid supports with 3D gel12,13 (e.g. polyacrylamide gel), or macromolecular (e.g. dendrimers)14–19 coatings. Compared to 2D substrates, the 3D surfaces have much higher binding capacity which can offer more reactive sites to bind with the targets, which could greatly improve the sensitivity of the microarray. Dendrimers are a unique class of polymers that do not form entangled chains associated with linear polymers and yet possess numerous chain ends that can be easily functionalized.16,20 In addition, dendrimers have a high biocompatibility which have been used as DNA transport agents in gene therapy to deliver genetic material to diseased sites.21,22 Due to its excellent characters, dendrimers have also been exploited to modify the surface for fabricating 3D DNA microarrays.14–19 The dendrimer modified surface not only has a very high binding capacity for DNA probes, but also reveals significant stability during the subsequently reacting and washing steps.
Currently, the detection of the dendrimer-based 3D microarray techniques mainly relies on the use of fluorescent molecular dye labels (such as cy3 and cy5), which have several potential drawbacks, such as needing expensive instrumentation for array reading, and photo-instability of the dyes employed.23–26 Recently, it has been recognized that metal, semiconductor, and magnetic nanoparticles can offer a unique set of physical properties that may be exploited in biological detection assays as an alternative to the use of fluorescent dyes.26–45 In particular, the detection of RLS by metal particles represents a great step forward, towards higher sensitivity, with the eventual goal of detecting single biomolecular binding events.26–28 Following the pioneering work of Mirkin's group, a series of gold nanoparticle-labelled microarray-based assays have been developed for DNA detection.26,34–45 In these assays, oligonucleotide targets are recognized specially by oligonucleotide-conjugates of gold nanoparticles followed by an enhancement step based on the electrodeless deposition of silver onto the gold nanoparticles for amplifying RLS signals. Under optimized experimental conditions, these assays make is possible to selectively detect oligonucleotide targets without the need for target amplification schemes such as the polymerase chain reaction (PCR).26 Most importantly, the RLS-based microarray format even can be read by a conventional flatbed scanner, thereby providing significant cost savings.
In this paper, a kind of 3D oligonucleotide microarray has been fabricated on the PAMAM modified surface which is generated by covalent reaction of the fourth-generation NH2-terminated poly(amidoamine) dendrimers (G4, PAMAM) with aldehyde terminated glass slides. Combined with the gold nanoparticle labelling strategy, a 3D microarray based RLS assay has been developed which can selectively detect oligonucleotide targets with highly sensitivity.
2. Experimental
2.1. Materials and reagents
DNA oligomers (see Table 1 for details) were purchased from Sangon Ltd. (Shanghai, China). Ethylenediamine (EDA) core poly(amidoamine) starburst generation 4 (G4) dendrimers with 64 surface primary amino groups (PAMAM, 10% (w/w) stock solution in methanol), Glutaraldehyde solution (GA, 25% aqueous solution) and tetrachloroaurate (HAuCl4) were purchased from Sigma Corp. (USA). Aldehyde modified glass microscope slides (named as 2D slides) were obtained from CapitalBio Ltd. (Beijing, China). Other chemicals were analytical grade and used as received. Milli-Q water (18.2 MΩ.cm) was used in all experiments.
Table 1 Sequences used in the experiments
Name |
Sequence |
P1 |
5′-TAA CAA TAA TCC-NH2-3′ |
P2 |
5′-TAA CAA TA TCC-NH2-3′ |
P3 |
5′-TAA CAA TA TCC-NH2-3′ |
P4 |
5′-TAA C A TAA TCC-NH2-3′ |
P5 |
5′-TAA C A TAA TCC-NH2-3′ |
P6 |
5′-T A CAA TAA TCC-NH2-3′ |
P7 |
5′-T A CAA TAA TCC-NH2-3′ |
P8 |
5′-T A C A TAA TCC-NH2-3′ |
P9 |
5′-T A CAA T G TCC-NH2-3′ |
P10 |
5′-TAA C A TA TCC-NH2-3′ |
T |
5′-GGA TTA TTG TTA AAT ATT GAT AAG GAT-3′ |
SP |
5′-HO(CH2)6-S-S-(CH2)6-T10 ATC CTT ATC AAT ATT-3′ |
Gold nanoparticles (13 nm in diameter) were synthesized by the traditional “Turkevich-Frens” method.46,47 Oligonucleotide conjugated gold nanoparticles (DNA@GNPs) were prepared by a previously reported procedure.48 Generally, the gold nanoparticle solution (500 μL, 6 nM) was incubated with disulfide-protected oligonucleotide SP (25 μL, 40 μM) in aqueous solution overnight, then diluted with 0.2 M NaCl and 10 mM phosphate buffer (500 μL). After further incubation for 2 h, the solution was evaporated to 150 μL by vacuum centrifugation. Excess oligonucleotides were removed by repeated centrifugation (9000 rpm, 3 times). Finally, the DNA@GNPs were redispersed into hybridization buffer (4 × SSC, 0.1% (w/v) SDS) with a final concentration of 5 nM.
2.3. Generation of the PAMAM dendrimer modified surface
The aldehyde modified glass slides were incubated in a solution containing PAMAM (0.1% (w/w), 30 mL) in methanol for 12 h at room temperature under gentle agitation. The slides were washed with 30 mL of methanol for 3 min (3 times) to remove excess dendrimers and dried by centrifugation (480 g for 1 min). Then, the slides were reacted with 30 mL of NaBH4 (10 mM) for 30 min. Subsequently, the modified surface was activated by 30 mL of GA (5% in PBS (pH 7.4, 50 mM PB, 0.15 M NaCl)) for 4 h, followed by washing with 30 mL of PBS (3 times), 30 mL of water (3 times), dried by centrifugation (480 g for 1 min) and stored at 4 °C. The PAMAM modified slides were named as 3D slides.
2.4. Fabrication of oligonucleotide microarray
The probe DNA oligomers (P1–P10) were dissolved in 15 μL of spotting buffer (3 × SSC, 1.5 M betaine, 0.005% (w/v) SDS) with desired concentration and spotted on the 3D slides or the commercial 2D slides by a SmartArrayer 48 system (Capitalbio Ltd., Beijing, China) under contact printing mode. After an overnight incubation under 90% humidity at 25 °C and further incubation for 15 min at 100 °C, the slides were rinsed with 30 mL of washing buffer (1 × SSC, 0.01% (w/v) SDS; 3 times) for 3 min and then incubated in 30 mL of blocking buffer (pH 7.4, 50 mM PB, 0.15 M NaCl supplemented with 0.1 M ethanolamine) at 30 °C for 1 h to de-activate remaining free aldehyde groups.
After the blocking step, the oligonucleotide microarrays were hybridized with target to the desired concentration with 20 μL of hybridization buffer (4 × SSC, 0.1% (w/v) SDS) for 20 min at 30 °C. Then, the slides were subjected to a series of rinsing steps: (1) 30 mL of hybridization buffer for 3 min at 30 °C (3 times); (2) 30 mL of hybridization buffer for 3 min at room temperature (3 times); (3) 30 mL of washing buffer for 3 min at room temperature (3 times); (4) 30 mL of Milli-Q water for 3 min (3 times), then dried by centrifugation (480 g for 1 min). Subsequently, the microarrays were labelled by DNA@GNPs (5 nM in hybridization buffer) and washed as previously described.
2.6. Silver enhancement and detection
After being treated with gold nanoparticles, silver enhancer (1
:
1 mixed (1 mL total volume) solutions A (AgNO3) and B (hydroquinone) (sigma-Aldrich)) were applied to each microarray for 8 min, washed with water (3 times), and dried by centrifugation (480 g for 1 min).26,39,40,49,50 Then, the slides were ready for detection by ArrayIt SpotWare Colorimetric Microarray Scanner (Telechem. International Inc.). According to the manufacturer's preset parameters, all images were collected with broad spectrum white light source.51
2.7. Data analysis
The background originating from the slide was recorded and subtracted from each image prior to evaluation. The mean value and standard deviation of the signals were determined for the 20 spot replicates per sample, respectively. The detection limit was determined to be the concentration where signal/standard deviation = 3 was reached. To determine the linear ranges of the curves, the range of concentrations that best fitted the linear equation y = mx + b were specified.
3. Results and discussion
3.1. Reaction capability of the 3D slide
The strategy for preparing the 3D slide is shown in Fig. 1a. First, a monolayer of PAMAM dendrimer was immobilized on the commercial 2D slides (2D planar glass slides with a uniform layer of linear aldehyde group), by a Schiff base linkage. Second, the Schiff base was reduced by NaBH4 which makes it possible to improve stability of the immobilized dendrimer layer. Subsequently, the dendrimer modified surface was activated by GA, a homobifunctional reagent. This procedure leads to the formation of a layer of aldehyde groups on the out end of dendrimers, which can be used directly for the covalent immobilization of amino-modified DNA oligomers. Because of their compact starburst structure, the dendrimers have a number of functionalities on the periphery, which leads to form a spherical layer of aldehyde groups over the 2D planner glass surface. The schematic representation of development of the 3D microarray-based RLS assay is shown in Fig. 1b. After hybridization of the immobilized probes by targets, DNA@GNPs were used to label the hybridization events. Subsequently, a silver enhancement step was applied to the microarrays for signal amplification since the light scattering properties of gold nanoparticles by themselves are relatively poor, if the particles are smaller than ca. 40 nm.26,52 After treatment with the silver enhancement solution, the microarrays were readily detected by the RLS microarray scanner. The effect of the deposition of silver on the surface morphology of the spots has been investigated by AFM and SEM in our previous reports.49,50After the enhancement procedure, the average size of nanoparticles increases due to silver deposition on the gold seeds from ca. 15 to 100 nm. Gold and silver features of this final size are very strong light scatterers and are readily detected even by a common office use flat bed scanner.26
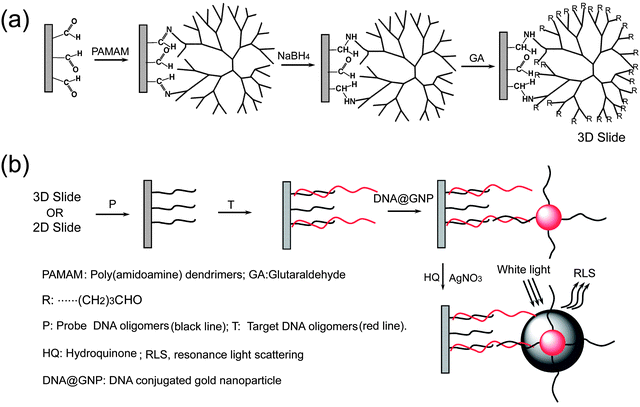 |
| Fig. 1 Schematic representations of the fabrication procedure of the 3D slide (a) and 3D microarray-based RLS assay (b). | |
The reaction capability of the 3D slide was determined by varying the concentration of oligonucleotide P1 in the spotting solution while keeping the concentration of target oligonucleotide T and the DNA@GNPs in the hybridization solution constant. The optimal signal-to-noise ratio (three times the standard deviation) is obtained at P1 concentrations above 50 nM. This signal is increased with increasing concentration of P1 from 50 nM to 5 μM and begins to saturate above 5 μM (as shown in Fig. 2). On the other hand, the 2D microarray shows relatively low RLS signal under the same experimental conditions. The experimental result indicates that the reaction capability of the 3D slide is much better than that of the commercial 2D slide.
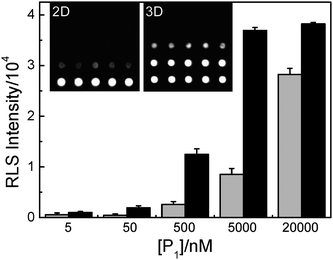 |
| Fig. 2 Light scattering images and corresponding analysis of 2D (gray columns) or 3D (black columns) oligonucleotide microarrays with different P1 (perfect match to T) concentrations. The concentration of T is 100 nM and the concentration of DNA@GNPs is 5 nM. | |
To evaluate the performance of the microarray formats, fifty identical reactions were analyzed, and the signal-to-noise ratio (S/N) and quality factor (Z′) were calculated (shown in Fig. 3), respectively. Comparing the S/N and Z′ values of these two substrates, we find that the performance of 3D microarray is better than that of 2D microarray.
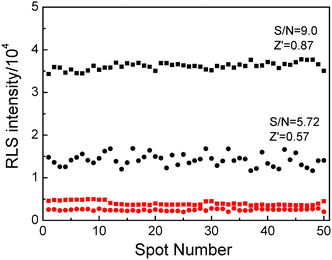 |
| Fig. 3 Evaluation of the assay performance. The observed RLS intensities of the spots both on the commercial 2D slide (black dots) and on the 3D slide (black squares) are shown in comparison with control measurements (red dots for the 2D slide, red squares for the 3D slide). The mean values and standard deviations extracted from the data were used to calculate the S/N ratio and Z′ factor. The concentration of P1 is 5 μM, the concentration of T is 100 nM and the concentration of DNA@GNPs is 5 nM. | |
3.2. Quantitative detection
A set of experiments was designed to determine the detection limit of the method described. As shown in Fig. 4, the target DNA can be detected as low as 100 pM with two orders of magnitude dynamic range (1 to 100 nM) by the 3D microarray-based RLS assay. The 2D technique for detecting the same target under the same conditions just has a detection limit of 10 nM. And the slope of the calibration line of the 3D microarray is 2.95 times higher than that of the 2D microarray. The result of this experiment suggests that the 3D microarray-based RLS assay is more sensitive than 2D microarray-based RLS assay.
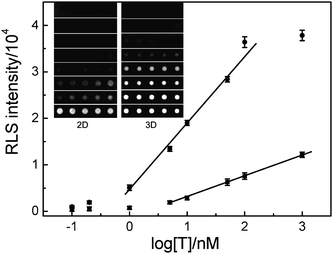 |
| Fig. 4 Light scattering images and the corresponding plots of the light scattering intensities of 2D (squares) or 3D microarray (dots) as a function of logarithm of T concentrations. The concentration of P1 is 5 μM, and the concentration of DNA@GNPs is 5 nM. | |
3.3. Discrimination of SNPs
It is important to develop cost-effective high throughput methods for identifying point mutations in DNA sequences because of the application of the SNPs in medical diagnosis. Search of mutations in a given gene must use dedicated chips that contain all possible sequences of the DNA in which one wishes to seek these mutations. Using our dendrimer-activated support, we have investigated a simple case in which one perfect match and six 12-mer oligonucleotide probes with a single base mutation at different positions (4, 8, and 11 from 3 terminals of DNA oligomers) were hybridized with a 27-mer oligonucleotide target in the solution. As shown in Fig. 5, hybridization is only effective with the oligonucleotide sequence strictly complementary to the 27-mer oligomer, while very weak RLS signals are obtained with the other six 12-mer oligonucleotides. And the RLS signals are dependent on the base types and positions of the mismatched site in the probes. For instance, the RLS signal is enhanced by increasing the distance of the mismatched site from the immobilized end (3 terminals of probe oligomers). At the same mismatched site, the T
:
G base pair containing oligonucleotide gives a higher RLS intensity than that of the T
:
C base pair containing one. This experimental result suggests that the destabilization of duplexes by T
:
G mismatches is slightly weaker than that of T
:
C mismatches. The phenomenon is consistent with the previously report by S. Ikuta et al.53 We also find that the RLS signal ratios of one base mismatch to perfect match of the 3D microarray are 0–4.2%, while the figures increase to 0–24.3% for the 2D microarray. In addition, no clearly measurable RLS signal is recorded with oligonucleotides bearing two mismatched bases (as shown in Fig. 5). The experimental results indicate that the selectivity of 3D microarray is higher than that of 2D microarray.
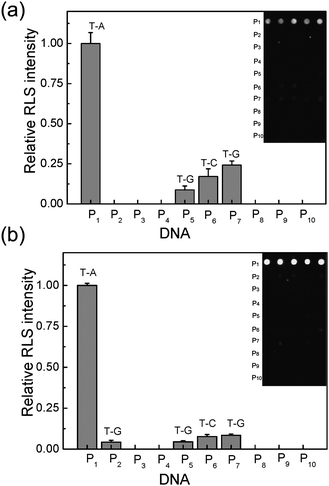 |
| Fig. 5 Light scattering images and the corresponding analysis for discrimination of SNPs. Ten probes (5 μM) were spotted both on the commercial 2D slide (a) and the 3D slide (b), respectively. The concentration of T is 100 nM and the concentration of DNA@GNPs is 5 nM. | |
4. Conclusions
We have generated a very effective chemically activated glass slide based on the use of G4 PAMAM dendrimers. By combining the gold nanoparticle labelling strategy, one kind of accurate, reliable and cost-effective DNA array is developed. As proof-of-principle experiments, the detection and SNP analysis of 27-mer oligonucleotide (based on the anthrax lethal factor sequence) have been carefully studied. The experimental results suggest that our approach could be used as a low cost high throughput technique for genetic studies including single-nucleotide polymorphism analysis and low abundance transcripts in cells.
Acknowledgments
The authors would like to thank the NSFC (Grant No. 20875087) and Chinese Academy of Sciences (Grant No. KJCX2-YW-H11) for financial supports.
References
- R. Lucito, J. Healy, J. Alexander, A. Reiner, D. Esposito, M. Y. Chi, L. Rodgers, A. Brady, J. Sebat, J. Troge, J. A. West, S. Rostan, K. C. Q. Nguyen, S. Powers, K. Q. Ye, A. Olshen, E. Venkatraman, L. Norton and M. Wigler, Genome Res., 2003, 13, 2291–2305 CrossRef CAS.
- C. A. Harrington, C. Rosenow and J. Retief, Curr. Opin. Microbiol., 2000, 3, 285–291 CrossRef CAS.
- M. Schena, D. Shalon, R. W. Davis and P. O. Brown, Science, 1995, 270, 467–470 CrossRef CAS.
- R. P. Balog, Y. E. P. de Souza, H. M. Tang, G. M. DeMasellis, B. Gao, A. Avila, D. J. Gaban, D. Mittelman, J. D. Minna, K. J. Luebke and H. R. Garner, Anal. Biochem., 2002, 309, 301–310 CrossRef CAS.
- C. L. Warren, N. C. Kratochvil, K. E. Hauschild, S. Foister, M. L. Brezinski, P. B. Dervan, G. N. Phillips and A. Z. Ansari, Proc. Natl. Acad. Sci. U. S. A., 2006, 103, 867–872 CrossRef CAS.
- Y. Chen, M. R. Shortreed, M. Olivier and L. M. Smith, Anal. Chem., 2005, 77, 2400–2405 CrossRef CAS.
- H. Matsuzaki, H. Loi, S. Dong, Y. Y. Tsai, J. Fang, J. Law, X. J. Di, W. M. Liu, G. Yang, G. Y. Liu, J. Huang, G. C. Kennedy, T. B. Ryder, G. A. Marcus, P. S. Walsh, M. D. Shriver, J. M. Puck, K. W. Jones and R. Mei, Genome Res., 2004, 14, 414–425 CrossRef CAS.
- A. Sassolas, B. D. Leca-Bouvier and L. J. Blum, Chem. Rev., 2008, 108, 109–139 CrossRef CAS.
- V. G. Cheung, M. Morley, F. Aguilar, A. Massimi, R. Kucherlapati and G. Childs, Nat. Genet., 1999, 21, 15–19 CrossRef CAS.
- M. C. Pirrung, Angew. Chem., Int. Ed., 2002, 41, 1276–1289 CrossRef.
- A. Camp and I. J. Bruce, Top. Curr. Chem., 2005, 260, 77–111.
- E. Fahy, G. R. David, L. J. DiMichele and S. S. Ghosh, Nucleic Acids Res., 1993, 21, 1819–1826 CrossRef CAS.
- V. Afanassiev, V. Hanemann and S. Wolfl, Nucleic Acids Res., 2000, 28, 66e CrossRef.
- M. Beier and J. D. Hoheisel, Nucleic Acids Res., 1999, 27, 1970–1977 CrossRef CAS.
- R. Benters, C. M. Niemeyer and D. Wöhrle, ChemBioChem, 2001, 2, 686–694 CrossRef CAS.
- R. Benters, C. M. Niemeyer and D. Wöhrle, Nucleic Acids Res., 2002, 30, 10e CrossRef.
- E. Tre'visiol, V. L. Berre, J. Leclaire, G. Pratviel, A. M. Caminade, J. P. Majoral, J. M. FrancËois and B. Meunier, New J. Chem., 2003, 27, 1713–1719 RSC.
- V. L. Berre, E. Tre visiol, A. Dagkessamanskaia, S. Sokol, A. M. Caminade, J. P. Majoral, B. Meunier and J. M. FrancËois, Nucleic Acids Res., 2003, 31, 88e CrossRef.
- S. S. Mark, N. Sandhyarani, C. Zhu, C. Campagnolo and C. A. Batt, Langmuir, 2004, 20, 6808–6817 CrossRef CAS.
- A. W. Bosman, H. M. Janssen and E. W. Meijer, Chem. Rev., 1999, 99, 1665–1688 CrossRef CAS.
- B. H. Zinselmeyer, S. P. Mackay, A. G. Schatzlein and I. F. Uchegbu, Pharm. Res., 2002, 19, 960–967 CrossRef CAS.
- C. S. Braun, J. A. Vetro, D. A. Tomalia, G. S. Koe and C. R. Middaugh, J. Pharm. Sci., 2005, 94, 423–436 CrossRef CAS.
- B. Schweitzer, S. Wiltshire, J. Lambert, S. O'Malley, K. Kukanskis, Z. Zhu, S. F. Kingsmore, P. M. Lizardi and D. C. Ward, Proc. Natl. Acad. Sci. U. S. A., 2000, 97, 10113–10119 CrossRef CAS.
- T. Kodadek, Chem. Biol., 2001, 8, 105–115 CrossRef CAS.
-
M. Schena, Microarray analysis, Wiley-Liss: Hoboken, NJ, 2003 Search PubMed.
- T. A. Taton, C. A. Mirkin and R. L. Letsinger, Science, 2000, 289, 1757–1760 CrossRef CAS.
- J. Yguerabide and E. E. Yguerabide, J. Cell. Biochem., 2001, 84(s37), 71–81 CrossRef.
- S. J. Oldenburg, C. C. Genick, K. A. Clark and D. A. Schultz, Anal. Biochem., 2002, 309, 109–116 CrossRef CAS.
- M. Han, X. Gao, J. Z. Su and S. Nie, Nat. Biotechnol., 2001, 19, 631–635 CrossRef CAS.
- A. P. Alivisatos, Nat. Biotechnol., 2004, 22, 47–52 CrossRef.
- E. Katz and I. Willner, Angew. Chem., Int. Ed., 2004, 43, 6042–6108 CrossRef CAS.
- C.-C. You, M. De and V. M. Rotello, Curr. Opin. Chem. Biol., 2005, 9, 639–646 CrossRef CAS.
- C. D. Medley, J. E. Smith, Z. Tang, Y. Wu, S. Bamrungsap and W. Tan, Anal. Chem., 2008, 80, 1067–1072 CrossRef CAS.
- J. Wang, F. Song and F. Zhou, Langmuir, 2002, 18, 6653–6658 CrossRef CAS.
- Y. Chen, J. Aveyard and R. Wilson, Chem. Commun., 2004, 2804–2805 RSC.
- J. J. Storhoff, S. S. Marla, P. Bao, S. Hagenow, H. Mehta, A. Lucas, V. Garimella, T. Patno, W. Buckingham, W. Cork and U. R. Müller, Biosens. Bioelectron., 2004, 19, 875–883 CrossRef CAS.
- G. J. Zhang, R. Möller, R. Kretschmer, A. Csáki and W. Fritzsche, J. Fluoresc., 2004, 14, 369–375 CrossRef CAS.
- R. Q. Liang, W. Li, Y. Li, C.y. Tan, J. X. Li, Y. X. Jin and K. C. Ruan, Nucleic Acids Res., 2005, 33, e17 CrossRef.
- Z. X. Wang, R. Lévy, D. G. Fernig and M. Brust, Bioconjugate Chem., 2005, 16, 497–500 CrossRef CAS.
- Z. X. Wang, J. Lee, A. Cossins and M. Brust, IEE Proc.: Nanobiotechnol., 2005, 152, 85–88 CrossRef CAS.
- G. A. Blab, L. Cognet, S. Berciaud, I. Alexandre, D. Husar, J. Remacle and B. Lounis, Biophys. J., 2006, 90, L13–L15 CAS.
- A. K. R. Lytton, M. S. Han and C. A. Mirkin, Anal. Chem., 2007, 79, 6037–6041 CrossRef CAS.
- A. Girigoswami, T. Li, C. Jung, H. Y. Mun and H. G. Park, J. Nanosci. Nanotechnol., 2009, 9, 1019–1024 CrossRef CAS.
- C. R. Hsiao and C. H. Chen, Anal. Biochem., 2009, 389, 118–123 CrossRef CAS.
- H. Kim, H. Takei and K. Yasuda, Sens. Actuators, B, 2010, 144, 6–10 CrossRef.
- J. Turkevich, P. C. Stevenson and J. Hillier, Discuss. Faraday Soc., 1951, 11, 55–75 RSC.
- G. Frens, Nat. Phys. Sci., 1973, 241, 20–22 Search PubMed.
- A. G. Kanaras, Z. X. Wang, A. D. Bates, R. Cosstick and M. Brust, Angew. Chem., Int. Ed., 2003, 42, 191–194 CrossRef CAS.
- Z. X. Wang, J. Lee, A. Cossins and M. Brust, Anal. Chem., 2005, 77, 5770–5774 CrossRef CAS.
- J. Q. Gao, D. J. Liu and Z. X. Wang, Anal. Chem., 2008, 80, 8822–8827 CrossRef CAS.
-
http://www.arrayit.com/Products/MicroarrayI/Scanner/scanner.html
.
- P. K. Jain, K. S. Lee, I. H. El-Sayed and M. A. El-Sayed, J. Phys. Chem. B, 2006, 110, 7238–7248 CrossRef CAS.
- S. Ikuta, K. Takagi, R. Bruce Wallace and K. Itakura, Nucleic Acids Res., 1987, 15, 797–811 CrossRef CAS.
|
This journal is © The Royal Society of Chemistry 2010 |