DOI:
10.1039/C0AY00105H
(Paper)
Anal. Methods, 2010,
2, 870-877
Received
11th February 2010
, Accepted 25th April 2010
First published on
18th May 2010
Abstract
Electric cell–substrate impedance sensing (ECIS) was used for probing inhibitory effects on Spodoptera frugiperda Sf9 insect cells exposed to five synthesized steroid compounds. The results were compared to the levels obtained using three ergostane-related steroids and five lanosta-related triterpenes purified from the fruiting bodies of Antrodia camphorata. The half-inhibition concentration (ECIS50), the level of 50% inhibition of the resistance response, was determined from the response function to establish inhibitory effects of the different compounds. Significant effects on inhibition as probed by impedance spectroscopy were noticed because of slight changes in chemical structure. Only two of the synthesized compounds, 24(R,S)-5α-lanost-8-ene-3,24,25-triol and 5α-lanost-8-ene-3,7,11-trione, showed inhibitory effects which were much less significant compared to the A. camphorata steroids. The ECIS50 values were 150–250 µM, similar to the value for the least inhibitory lanosta-related triterpene. This noninvasive measurement in combination with Sf9 insect cells has been proven as a simple and reliable tool for screening inhibition/cytotoxicity and designing steroid-related compounds.
Introduction
Electric cell–substrate impedance sensing (ECIS) has been proven as a useful analytical tool for probing cell spreading, morphological change, and micromotion, the three important parameters in cell culturing.1,2 The technique is based on a microarray of circular gold electrodes (250 µm diameter), inserted into the bottom of tissue culture wells. Upon inoculation, cells drift downwards through the culture medium and settle on the bottom of tissue culture wells. The cells then begin to attach and spread on the electrode surface, precoated with a binding protein, known as extracellular matrix (ECM). Under a noninvasive applied potential, the measured impedance changes drastically after cell attachment and spreading, due to an interference with the free space above the electrode. The changing impedance can be monitored and interpreted to reveal information about cell spreading.3,4 The broad response to changes in the environment such as the addition of chemical compounds allows this method to serve as a general tool for probing cell behavior as well as an alternative to animal testing for toxicology studies. The applicability of ECIS for inhibition assay has been demonstrated using toxic or noxious agents such as cytochalasin D (cytoskeletal inhibitor),5 prostaglandin E2 (inflammatory mediator),6 bacterial protease (bacterial proteins that perturb ECM and cytoskeleton),7 environmental toxins such as heavy metals and nitrotoluenes,8–10 as well as nanomaterials including quantum dots and gold nanoparticles.11 Mammalian cells have been used extensively with ECIS to probe cell behavior including spreading, micromotion, and cytotoxicity.9–11 Nevertheless, insect cells (Spodoptera frugiperda Sf9) have also been used with ECIS to probe the inhibitory effects of isolates from Antrodia camphorata12 and destruxins from Metarhizium anisopliae.13 In both cases, cell spreading was inhibited although no cytotoxicity was observed.
In this paper, ECIS together with S. frugiperda Sf9 insect cells has been used to compare cytotoxicity/inhibition of five synthesized steroids (Table 1) and eight natural steroids (Table 2) previously reported.12 Five steroid compounds were synthesized and compared with ergostane-related steroids and lanosta-related triterpenes isolated from A. camphorata in an attempt to elucidate the relationship between inhibitory effects and chemical structures. Important biological activities have been reported for A. camphorata such as anti-cancer,14–17 antihepatotoxic,18,19 antihypertensive,20 antiinflammatory,21,22 antioxidant,23 and neuroprotective.24 The synthesis route was advocated in our study since this mushroom grows only on one species of rainforest tree, Cinnamomum kanehirae, a native of Taiwan. This tree has become increasingly rare and is now protected by the Taiwanese government. Cell-based impedance sensing served as an analytical tool to guide the synthesis of ergostane and lanostane analogs with different side chains and their inhibitory effects. To our knowledge, this was the first attempt to compare the effect of synthesized steroids with chemically similar natural counterparts.
Table 1 Chemical structures and some predicted properties of five synthetic compounds
C32H54O3, Mw: 486.77, no apparent pKa, solubility = 8.32 × 10−5 g L−1, independent of pH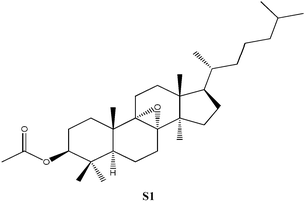 |
C32H56O3, Mw: 468.75, no apparent pKa, solubility = 1.21 × 10−7 g L−1, independent of pH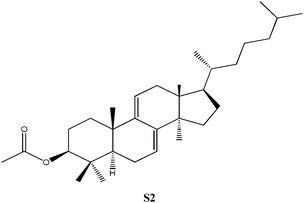 |
C33H54O2, Mw: 484.75, solubility = 1.39 × 10−6 g L−1, no apparent pKa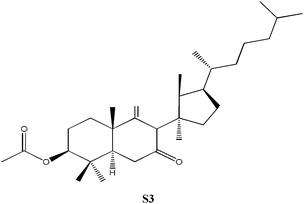 |
C30H46O3, Mw: 454.68, solubility = 1.76 × 10−5 g L−1, no apparent pKa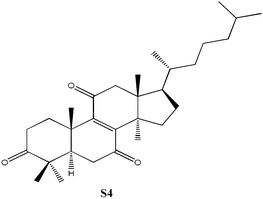 |
C30H52O3, Mw: 460.73, solubility = 8.32 × 10−5 g L−1, pKa = [14.67, 15.16, 16.19]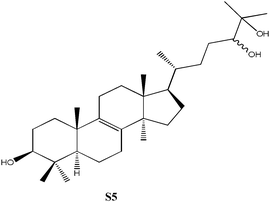 |
|
Table 2 Chemical structures and molecular weights of eight natural compounds isolated from A. camphorata
Dehydroeburicoic acid (or) 24-methylenelanosta-7,9(11)-diene-3β-ol-21-oic acid (N1), Mw: 468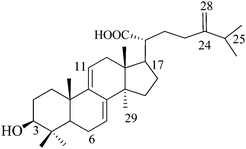 |
Dehydrosulfurenic acid (or) 24-methylenelanosta-7,9(11)-diene-3β,15a-diol-21-oic acid (N2), Mw: 484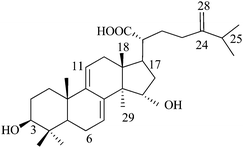 |
15a-Acetyl dehydrosulfurenic acid (or) 15a-acetoxy-24-methylenelanosta-7,9(11)-diene-3β-ol-21-oic acid (N3), Mw: 526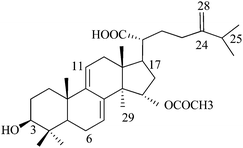 |
3β,15a-Dihydroxy lanosta-7,9(11), 24-triene-21-oic acid (N4), Mw: 470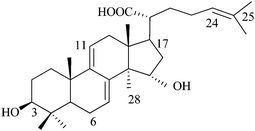 |
Antcin B (or) Zhankuic acid A (or) 4a-methylergosta-8,24(28)-diene-3,7,11-trion-26-oic acid (N5), Mw: 468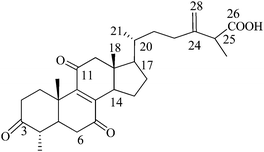 |
Methyl antcinate B (or) Zhankuic acid D (N6), Mw: 482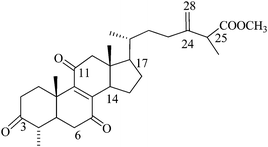 |
Zhankuic acid C (or) 3a,12a-dihydroxy-4a-methylergosta-8,24(28)-diene-7,11-dione-26-oic acid (N7), Mw: 486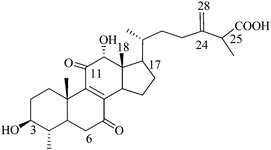 |
Sulfurenic acid (or) 24-methylenelanosta-8-ene-3β,15a-diol-21-oic acid (N8), Mw: 466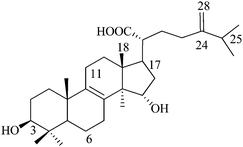 |
Experimental
Materials
All materials were obtained commercially (guaranteed reagent grade) and used without further purification. Concanavalin A (Con A, Type III) from Canavalia ensiformis and Trypan Blue were purchased from Sigma-Aldrich (St Louis, MO).
Synthesis of steroids
3β-Acetoxy-5α-lanost-7,9(11)-diene (S2)12,25,26.
Boron trifluoride diethyl etherate (0.71 mL, 5.52 mmol) was added to a stirring solution of 3β-acetoxy-8α,9α-epoxy-5α-lanostane (S1) (0.400 g in 30 mL toluene). After 2 h at room temperature with stirring, the reaction mixture was washed with sodium bicarbonate solution (3 × 25 mL, 10%), brine (2 × 25 mL) and water (2 × 25 mL). The organic phase was dried over MgSO4 and concentrated under reduced pressure, yielding a white solid. Purification by column chromatography (ethyl acetate/hexane 95 : 5) gave the pure product (S2) as a white crystalline solid (0.360 g, 94%).
3β-Acetoxy-5α-lanost-9(11)-en-7-one (S3)25,29,30.
Chromium trioxide (0.170 g) in 15 mL acetic acid (90%, v/v) was heated to 80 °C and added to a stirring solution of 3β-acetoxy-5α-lanost-8-ene27,28 (0.800 g) in 27 mL glacial acetic acid at 80 °C. After 1 h at 80 °C with stirring, the reaction mixture was cooled on an ice-bath, poured into water (30 mL) and extracted with chloroform (3 × 25 mL). The combined organic extracts were washed with water (4 × 30 mL), dried over MgSO4 and concentrated under reduced pressure, yielding an orange solidifying oil. Purification by column chromatography (ethyl acetate/hexane 95 : 5) led to the isolation of two fractions one of which was the desired product 3β-acetoxy-5α-lanost-9(11)-en-7-one (S3) as a yellow solidifying oil; the other fraction isolated was 3β-acetoxy-5α-lanost-8-ene-7,11-dione. Recrystallization of S3 from methanol gave white crystalline needles (0.300 g, 36%); mp 151–153 °C; νmax (KBr)/cm−1 1731 (C
O ester), 1704 (C
O, ketone), 1466, 1373, 1249.
5α-Lanost-8-ene-3,7,11-trione (S4)12,25,26,31.
Chromium trioxide (0.470 g) in 15 mL acetic acid (90%, v/v) was heated to 80 °C and added to a stirring solution of dihydrolanosterol27,28 (0.500 g) in 20 mL glacial acetic acid at 80 °C. After 1 h at 80 °C with stirring, the reaction mixture was cooled on an ice-bath, poured into water (40 mL) and extracted with chloroform (3 × 40 mL). The combined organic extracts were washed with water (4 × 30 mL), dried over MgSO4 and concentrated under reduced pressure, yielding a yellow solidifying oil. Purification by column chromatography (benzene/dichloromethane 50 : 50) led to the isolation of the desired product 5α-lanost-8-ene-3,7,11-trione (S4) as a cream colored solid (0.125 g, 25%); (found C, 79.07; H, 10.16. C30H46O3 requires C, 79.25; H, 10.20%); νmax (KBr)/cm−1 1711 (C
O, ketone), 1674, 1225.
24(R)-5α-Lanost-8-ene-3,24,25-triol (S5a) and 24(S)-5α-lanost-8-ene-3,24,25-triol (S5b)32.
Acetyl chloride (0.71 mL) was added dropwise to a stirring solution of 3β-acetoxy-5α-lanost-8-ene-24,25-diol27,28 (1.0 g, 1.99 mmol) in a mixture of anhydrous dichloromethane (25 mL) and anhydrous MeOH (25 mL) at 0 °C. After 72 h at room temperature with stirring, the reaction mixture was neutralized with triethylamine (1.4 mL, 9.94 mmol), poured into water (100 mL) and extracted with DCM (3 × 30 mL). The combined organic extracts were washed with brine (2 × 25 mL), water (5 × 25 mL), dried over MgSO4 and concentrated under reduced pressure, yielding a yellow solid. Purification by column chromatography (hexane/ethyl acetate 2 : 1) led to the isolation of the desired products 24(R)-5α-lanost-8-ene-3,24,25-triol (S5a) and 24(S)-5α-lanost-8-ene-3,24,25-triol (S5b) as an off-white crystalline solid and a 1 : 1 mixture of C-24 β- and α-OH. Recrystallization twice from acetone gave the pure product as a white crystalline solid (0.700 g, 76%); νmax (KBr)/cm−1 3414, 2951; ESI-MS (M − OH)+: 443.4 (M+ + H, 90%).
NMR signatures of the five synthesized compounds are given in ESI†. Some physio-chemical properties of the five synthesized steroids given in Table 1 were predicted using ACD/structure designer software (ACDLABS, Toronto, ON, Canada).
Isolation of compounds from A. camphorata
Three ergostane-related steroids and five lanosta-related triterpenes were extracted, isolated and purified from the fruiting bodies of the fungus A. camphorata as previously reported.12 In brief, the fruiting bodies of A. camphorata were obtained through a solid state cultivation (SSC) process (accession number G908AC). The freshly collected materials were dried under shade, sliced into small pieces, pulverized using a mechanical grinder and passed through 40 mesh sieve, and preserved in an airtight container for further use. The powdered fruiting bodies of A. camphorata (30 g) were extracted with CHCl3 (5 × 200 mL), and MeOH (5 × 200 mL), successively using a Soxhlet extractor until the refluxed solvent became colorless. After extensive extraction, all the extracts were filtered individually, and the solvent was dried by rotary evaporation under reduced pressure at a temperature maximum of 35 °C to give brownish-black colored residues in yields of 12.0 g (40.0%, w/w) and 1.78 g (5.9%, w/w), respectively. The chloroform extract was subjected to silica gel chromatography (4 × 90 cm, 0.063–0.200 mesh). The column was eluted with solvents of increasing polarity using a mixture of n-hexane/EtOAc to obtain three ergostane-related steroids and five lanosta-related triterpenes.
Cell line and culture conditions
S. frugiperda Sf9 cells were maintained in 125 mL disposable Erlenmeyer flasks with a working volume of 20 mL in serum-free SF-900 II medium (Gibco BRL, Canadian Life Technologies, Burlington, ON, Canada). Cells were cultured weekly at 0.4 × 106 cells mL−1 at 27 °C, pH 6.2 with agitation at 110 rpm. The monitoring of the cell count and viability by the Trypan Blue exclusion assay during the growth was performed with a CEDEX Innovatis cell counter (Bielefeld, Germany). Sf9 cells, inoculated at an initial cell density of 0.4 × 106 cells mL−1, were grown to the mid-exponential phase of 2.5 to 3 × 106 cells mL−1 and the resulting cells were aseptically centrifuged at 1500 rpm for 4 min. Pellets were thereafter suspended at a cell concentration of 3 × 106 cells mL−1 in fresh medium.
Concanavalin A (Con A, 0.40 mL, 0.5 mg mL−1, prepared fresh daily in 50 mM PBS, pH 7.4 with the aid of sonication for 1 h) was added into each of the 8 wells of a sensing chip (8W1E, Applied Biophysics, Troy, NY) to coat the detecting gold electrodes as previously described elsewhere.8 Con A binds quickly (90% of the change occurs in the first 10 min) to the electrode surface as confirmed by a slight increase in impedance and the attachment is very stable as previously reported.8 After protein adsorption (approx. 30–60 min), the wells were washed 3 times with 0.85% NaCl and 0.4 mL of culture medium was placed in each well and the impedance baseline was monitored in the ECIS humidified chamber for 1–2 h at 27 °C. The wells were then emptied and used for probing the inhibitory effect of steroids. In brief, the synthesized steroids were dissolved in ethanol (approx. 0.5–1.0 mL) to concentrations of approx. 10–100 mM. These samples (30 µL) were added to cell suspensions (1.5 mL at 3 × 106 cells mL−1) at various concentrations before adding 0.4 mL of the resulting suspension to 2 or 3 wells to test for possible inhibitory effects. For each steroid compound, 6 different concentrations including a control with 30 µL ethanol were tested at the same time and each steroid was analyzed 2–3 different times.
Impedance measurement with ECIS
Detailed information on ECIS impedance measurement has been reported elsewhere.8–13 In brief, the system can measure up to 16 sample wells (2 chips of 8 wells, each containing a singly addressable detecting electrode) per experiment. A common counter gold electrode is shared by the 8 detecting electrodes and the two electrodes (detecting gold electrode and counter gold electrode) of the well are connected to a lock-in amplifier of the ECIS system. Cell behavior should not be affected if the applied potential is 1 V AC or less.33,34 The impedance of each well was measured every 2 min at 4 kHz, and the system acquires resistance, impedance and capacitance data. However, as larger changes occurred in the resistance we have focused on these changes in this study. The ECIS50 value derived from the time response function, f(C,t), was calculated as described by Xiao et al.9 For simplification of plots and calculations, data points at 30 min intervals were selected from the raw resistance data. The sensing chip could be temporarily removed (pause function from the software) from the ECIS system incubator and placed on a Wilovert AFL 30 inverted microscope (Hund, Germany) equipped with a digital video camera (KP-D50U, Hitachi, Tokyo, Japan) to observe the cells during experimentation.
Results and discussion
Synthesis of steroid compounds
3β-Acetoxy-5α-lanost-8-ene was prepared using previously published procedures starting from commercial lanosterol.27,28 Epoxidation under standard conditions using m-CPBA gave one stereoisomer, the 8α,9α-epoxide (S1) on the less sterically hindered α-face in 57% yield.25,26 Treatment of the epoxide (S1) with boron trifluroide–diethyl ether gave the diene (S2) in excellent yield (94%).12,25,26 Allylic oxidation of 3β-acetoxy-5α-lanost-8-ene using chromium trioxide leads to the formation of two main products which could be separated by chromatography and which were identified as the desired enone (S3) (36%) and the side-product enedione (22%).25,29,30 Dihydrolanosterol was prepared from commercial lanosterol using previously published procedures.27,28 The 5α-lanost-8-ene-3,7,11-trione (S4) was synthesized in modest yield (25%) from dihydrolanosterol using 4 equivalents of chromium trioxide.12,25,26,31 3β-Acetoxy-5α-lanost-8-ene-24,25-diol was also prepared from commercial lanosterol27,28 and was deacetylated using acetyl chloride in dichloromethane/methanol to give the 24(R)-5α-lanost-8-ene-3,24,25-triol (S5a) and 24(S)-5α-lanost-8-ene-3,24,25-triol (S5b) as a 1 : 1 mixture of diastereomers in 76% yield (C-24 β- and α-OH).32 Recrystallization from acetone twice was necessary to obtain a sample pure enough for testing. 24(R)-5α-Lanost-8-ene-3,24,25-triol (S5a) and 24(S)-5α-lanost-8-ene-3,24,25-triol (S5b) have been recently isolated from the MeOH extract of the sclerotia of Inonotus obliquus (a white-rot fungus used traditionally for treating cancer, heart, liver and stomach diseases) and are reported as new lanostane-type triterpenes, inoterpenes A and B.321H and 13C NMR spectra for all compounds synthesized were assigned based on correlation with published data and NMR studies on similar compounds.
Response of Sf9 insect cells
The gold electrode was fully covered by Con A; a lectin purified from Canavalia ensiformis as reported previously.8 The resistance of the culture medium without cells was ∼2.5 kΩ while with cells there was a slight increase to ∼3.0 kΩ (defined as the reference resistance, Ro). Without the steroid compounds, the cells descended to the bottom of the well within 20 min as observed by the video-enhanced microscope and as they spread, the cells changed from round shapes to flattened forms with much larger dimensions. As the cells spread, they alter the effective area available for current flow, causing a significant increase in the resistance of the well from 3.0 kΩ to 9.6 kΩ (Rt). The resistance change was then defined as ΔRs = (Rt − Ro) and shown in Fig. 1A (curve a). The number of normal Sf9 cells to completely cover an 8W1E detecting electrode coated with Con A was previously reported8 to be between 150 and 200 cells and therefore the estimated resistance change contributed by each attached cell was about 35–45 Ω per cell.
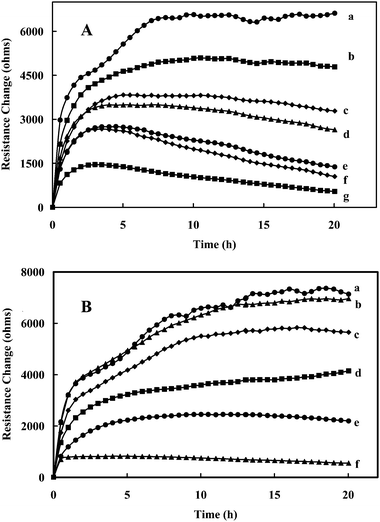 |
| Fig. 1 (A) Increase in resistance response (ΔRs) of Sf9 insect cells to various concentrations (µM) of 5α-lanost-8-ene-3,7,11-trione (S4): (a) 0, (b) 40, (c) 100, (d) 200, (e) 400 (f) 1000, and (g) 2000. (B) Increase in resistance response (ΔRs) of Sf9 insect cells to various concentrations (µM) of Antcin B (Zhankuic acid A) (N5): (a) 0, (b) 1.2, (c) 2.3, (d) 4.6, (e) 11.6, and (f) 23.2. | |
Addition of a low concentration (40 µM) of the 5α-lanost-8-ene-3,7,11-trione (S4, Table 1) to the cell suspension only exhibited a slight effect on the resistance signal (Fig. 1A, curve b). However, as the concentration was increased (100 µM–2.0 mM) the resistance change was significantly decreased (Fig. 1A, curves c–g). Inverted fluorescent microscopy (Fig. 2A) confirmed that the insect cells in the absence of the steroid were intact and well spread on the Con A coated electrode surface even after washing the wells with saline 3 times. However, insect cells exposed to the steroid (400 µM) for 24 h were more spherical (Fig. 2B), compared to the control cells. For comparison, Fig. 1B shows the effects of Antcin B (N5—Zhankuic acid A) from A. camphorata on the Sf9 insect cells for experiments performed in a similar manner. As can be observed, the inhibitory effect was much more pronounced than with the synthesized 5α-lanost-8-ene-3,7,11-trione (S4) since much lower concentrations (1.2–23.2 µM) of Antcin B gave similar effects.
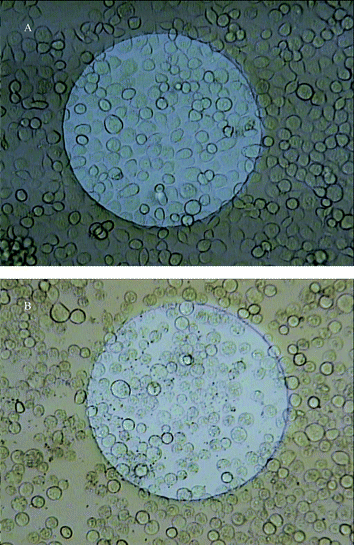 |
| Fig. 2 Microscopic photos of the electrode surface after 24 h: (A) electrode at 0 µM 5α-lanost-8-ene-3,7,11-trione (S4), (B) electrode at 400 µM 5α-lanost-8-ene-3,7,11-trione (S4). | |
Half-inhibition concentration (ECIS50) for 5α-lanost-8-ene-3,7,11-trione (S4)
For the effector cells, the resistance change (ΔRs) of the well is dependent on the number (No) of initial cells attached on the detecting electrode, the toxicant concentration (C) and the exposure time (t) as reported by Xiao et al.9 The resistance change normalized by No is defined as the cell response to the toxicant measured by ECIS, f(C,t) = ΔRs/No. As a control with no toxicant, C is equal to zero and f(0,t) increases as the cells spread on the electrode and reaches a plateau. In the presence of toxicant, f(C,t) after an initial increase the value decreases and can even approach zero, indicating total cell death at high toxicant concentrations. The inhibitor concentration required to achieve 50% inhibition of the response is defined as the half-inhibition concentration (ECIS50) or f(ECIS50,t)/f(0,t) = 50%.
As an example, the ECIS50 for 5α-lanost-8-ene-3,7,11-trione (S4) was calculated from the data obtained in Fig. 1A. For S4, the time response function f(C,t) was used to construct a series of inhibition curves at any given time t0 (>5 h) for the series of S4 concentrations used in Fig. 1A. Very few cells (<10%) were observed in the medium of the well, while 164 cells were counted on the electrode surface after the removal of this medium. After 5 h, the cells on the electrode surface spread out to form a confluent layer and affected an increase in resistance. The presence of the S4 did not interfere with the initial settling of the insect cells, although the spreading was not as evident as time progressed especially at high concentrations of S4. As a result, No for each well was assumed to be equivalent, therefore no adjustment for ΔRs was required due to different No values. The time response function, f(C,t), was then normalized by simply taking the ΔRs, i.e., Rt − R0 at different steroid concentrations and dividing these values by the ΔRs value at f(0,t). As expected, the normalized time response function decreased as the concentration of S4 increased for all exposure times considered (Fig. 3).
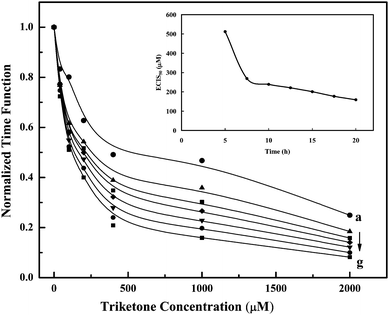 |
| Fig. 3 5α-Lanost-8-ene-3,7,11-trione (S4): inhibition curves were obtained for each S4 concentration (Fig. 1A, curves a–g) at various exposure times (h): (a) 5.0, (b) 7.5, (c) 10, (d) 12.5, (e) 15, (f) 17.5 and (g) 20. The normalized time response function (y-axis), f(C,t), was determined by taking the ΔRs (Fig. 1A, curves b–g), i.e., Rt − R0 at different S4 concentrations and dividing the values by the ΔRs (∼6.6 kΩ, Fig. 2, curve a) at f(0,t). Inset: relationship between the half-inhibition concentration (ECIS50) and exposure time during cell culture for 5α-lanost-8-ene-3,7,11-trione (S4). The ECIS50 value obtained for S4 was determined for each exposure time (Fig. 3, curves a–g) by extrapolating the value for the x-axis from the y-axis (0.5). | |
The ECIS50 value for S4 was determined for each exposure time by extrapolating the value on the concentration axis when the normalized time response function was 0.5. Fig. 3 (inset) shows the relationship between the half-inhibition concentration and exposure time, indicating that the ECIS50 for S4 leveled off at approx. 150–200 µM after an exposure time of 10 h. There was a lag time before S4 generates the inhibitory effect as indicated by higher ECIS50 values at lower exposure times. In this study, due to the rapid settling of the cells on the surface at high cell concentration, the ECIS50 value was a reflection of the interference or inhibition capacity of the steroid with respect to the cell spreading on the substratum. Therefore, the effect of S4 on the ECIS response was inhibitory, not cytotoxic. The ECIS50 value (approx. 150–200 µM, Fig. 4A) for S4 was much higher than those previously reported12 for the two ergostane-related steroids (N5 and N6 in Table 2) isolated from A. camphorata, which also contain the 3,7,11-trione structure. For example, Zhankuic acid D (methyl antcinate B) and Zhankuic acid A (Antcin B) had ECIS50 values of 12.9 and 6.8 µM (Fig. 4B), respectively (Table 3). The major structural differences between the synthesized S4 and these two ergostane-related steroids are the absence of the diene at position 24, as well as the absence of the carboxylic acid moiety at position 25. The S4 also has additional methyl groups at positions 4 and 14. These slight structural changes resulted in significant reduction in inhibition as quantified by the ECIS approach.
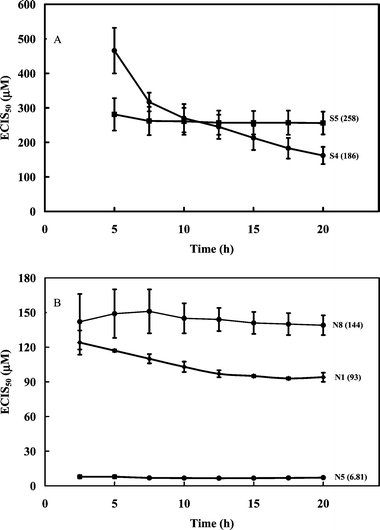 |
| Fig. 4 (A) Relationship between the half-inhibition concentration (ECIS50) and time, during cell culture for 5α-lanost-8-ene-3,7,11-trione (S4) and 24(R,S)-5α-lanost-8-ene-3,24,25-triol (S5a,b). (B) Relationship between the half-inhibition concentration (ECIS50) and time, during cell culture for 24-methylenelanosta-7,9(11)-diene-3β-ol-21-oic acid (N1, dehydroeburicoic acid), 4a-methylergosta-8,24(28)-diene-3,7,11-trion-26-oic acid (N5, Antcin B or Zhankuic acid A) and 24-methylenelanosta-8-ene-3β,15a-diol-21-oic acid (N8, sulfurenic acid). Data expressed as SEM, n = 2–3. ECIS50 values (µM) given in brackets. | |
Table 3 ECIS50 values for A. camphorata isolates (N1–N8) and synthetic compounds (S1–S5)
Compound |
ECIS50/µM |
Comments and remarks |
N1
|
93 |
Lanostane steroid with 7,9(11)-diene. |
N2
|
29 |
Similar to N1 except hydroxyl at position 15, more inhibitory than N1. |
N3
|
77 |
Similar to N2, except bulky acetoxy group at position 15. Less inhibitory than N2. |
N4
|
59 |
Similar to N1 and N2 except for no double bond at position 24, has hydoxyl at position 15. Less inhibitory than N2, but more inhibitory than N1. |
N5
|
6.8 |
Ergostane steroid. Difference from lanostanes: three ketone groups, carboxylic acid at 26 not 21, methyl at position 4 rather than dimethyl. More inhibitory than lanostanes. |
N6
|
12.9 |
Similar to N5 except methyl ester group at position 26, less inhibitory than N5. |
N7
|
16.4 |
Similar to N5 except only 2 ketone groups. Ketone at position 3 replaced by hydroxyl, less inhibitory than N5. |
N8
|
144 |
Similar to N1, but no diene at 7,9(11) only ene at position 8. Least inhibitory of all steroid isolates. |
S1
|
No effect up to 150. |
Similar to N1, but acetoxy group at position 3 and no carboxylic group at position 21, no inhibition. |
S2
|
No effect up to 300. |
Similar to S1, but contains an epoxy group at positions 8 and 9, no inhibition. |
S3
|
No effect up to 100. |
Similar to S1, but has a ketone group at position 7, no inhibition. |
S4
|
150–200 |
Similar to N5, but dimethyl at position 4 and no carboxylic group at position 26, less inhibitory than N5. |
S5
|
250 |
Similar to N8, but methyl rather than carboxylic acid at position 21, no hydroxyl at position 15 and two hydroxyls at positions 24 and 25. Less inhibitory than N8. |
Inhibitory effect versus chemical structural change
Eight natural compounds and five synthesized steroids possess similar chemical structures except for some minor variation. Therefore, they were very useful in our systematic study to decipher a relationship between inhibition and chemical structure. The two natural isolates (N1 and N2) are the two original compounds synthesized by A. camphorata that undergo photochemical and enzymatic transformation to other isolates.35 Notice also that cyclodextrin-modified capillary electrophoresis using two effective chiral selectors, methyl-β-cyclodextrin and sulfobutyl ether-β-cyclodextrin, only shows a single peak for both N1 and N2. Considering the ECIS50 values obtained for the four lanosta compounds (N1–N4, Table 3), the hydroxylation at C15 of N1 significantly increased the inhibition effect (N1 and N2). However, the presence of an acetoxyl group at this position (N3) or any change in the double bond position in the side chain resulted in much lesser effects. The presence of two double bonds in the ring also played an important role in inhibition as reflected by the ECIS50 value for N8, 144 µM, compared to 29 µM for N2. The three most potent compounds (N5–N7) possessed only one methyl group at C4 instead of two as observed for (N1–N4 and N8). In addition, the three ketone compounds (N5 and N6) were more inhibitory than the two ketone compound (N7). Modification of the side chain also played a noticeable inhibition effect and again the replacement of the carboxyl group by an acetoxyl group appeared to reduce inhibition (N5 and N6).
A series of experiments was conducted using the other four synthetic steroid compounds (S1, S2, S3, and S5) to confirm the role of side chain on inhibition. All five synthetic compounds possessed two methyl groups at C4. Impedance analysis indicated that S5 had an inhibitory effect similar to S4 as the ECIS50 value leveled off at approximately 250 µM (Fig. 4A). S5 (Table 1) differs from S4 in that position 3 contains a hydroxyl group rather than the ketone group. In addition, the ketones at positions 7 and 11 have been removed and hydroxyls were added at positions 24 and 25. The S5 structure is more similar to the lanosta-related triterpenes isolated from A. camphorata which as previously reported12 had ECIS50 values ranging from 29–144 µM (Table 3 and Fig. 4B—N2 and N8). A major structural difference is that S5 does not contain the 7,9-diene present in the majority of the more inhibitory lanosta-related triterpenes (N1, N2, N3, and N4 in Table 2), but rather has a single ene group at position 8 similar to sulfurenic acid (N8 in Table 2), the least inhibitory (ECIS50 = 144 µM) lanosta-related triterpene (Table 3). S5 also lacks the hydoxyl group at position 15 contained in sulfurenic acid, which could explain the reason why it is even less inhibitory.
S1, S2 and S3 were tested, but found to have no significant inhibitory effects on the Sf9 insect cells. A lanosta-related steroid similar to those isolated from A. camphorata with a conjugated diene at positions 7 and 9 (S2, Table 1) was tested up to 150 µM without effect. Rather than a hydroxyl group at position 3, this compound had a bulky acetate group which could be responsible for the lack of inhibition. Similarly, when the 7,9-diene was replaced by an 8α,9α-epoxide group (S1, Table 1) no inhibition was observed up to 300 µM. The last compound tested (S3, Table 1), contained a ketone moiety at position 7, but no inhibition was observed up to 100 µM. The attempt to replace the hydroxyl at C3 by an acetoxyl group again reduced inhibition significantly (S1–S3). Such behavior was also observed for N3 and N6 as discussed earlier. Epoxidation of the aromatic ring did not improve inhibition (S1). Chirality versus inhibition is another important issue, a subject for future endeavor. Both N5 with ECIS50 of 6.8 µM and N7 with ECIS50 of 16.4 µM appear as a chiral pair in cyclodextrin-modified capillary electrophoresis.35 However, N6 with ECIS50 of 12.9 µM only appears as one single peak. The synthesized S5 compound consists of a two chiral pair as discussed earlier.
Conclusion
In brief, an online and continuous technique based on electric cell–substrate impedance sensing (ECIS) was used to study the time response function of Sf9 insect cells exposed to a series of synthesized steroid-related compounds. The technique was extremely sensitive to detecting inhibition in response to slight changes in the chemical structures of such compounds. This noninvasive measurement in combination with Sf9 insect cells is a simple and reliable tool for screening and designing steroid-related compounds. Together with natural steroids isolated from A. camphorata, the five synthesized compounds were useful to provide more in-depth information about the relationship between inhibition and chemical structure. Work is in progress to synthesize more effective steroids which are comparable to the most effective natural steroids. It has become increasingly difficult to find Antrodia fruit bodies in the forest and the price of such material is exorbitant.
Acknowledgements
The authors thank Johnny Montes of the Biotechnology Research Institute (BRI), National Research Council Canada (NRC), Montreal, Quebec, Canada for proving the Sf9 insect cells.
References
- P. Mitra, C. R. Keese and I. Giaever, BioTechniques, 1991, 11, 504–509 CAS.
- I. Giaever and C. R. Keese, Nature, 1993, 366, 591–592 CrossRef.
- M. Kowolenko, C. R. Keese, D. A. Lawrence and I. Giaever, J. Immunol. Methods, 1990, 127, 71–77 CrossRef CAS.
- C.-M. Lo, C. R. Keese and I. Giaever, Biophys. J., 1995, 69, 2800–2807 CrossRef CAS.
- C.-M. Lo, C. R. Keese and I. Giaever, Exp. Cell Res., 1993, 204, 102–109 CrossRef CAS.
- T. J. Smith, H. S. Wang, M. G. Hogg, R. C. Henrikson, C. R. Keese and I. Giaever, Proc. Natl. Acad. Sci. U. S. A., 1994, 91, 5094–5098 CrossRef CAS.
- K. S.-C. Ko, C.-M. Lo, J. Ferrier, P. Hannam, M. Tamura, B. C. McBride and R. P. Ellen, J. Microbiol. Methods, 1998, 34, 125–133 CrossRef CAS.
- J. H. T. Luong, M. Habibi-Razaei, J. Meghrous, C. Xiao, K. B. Male and A. Kamen, Anal. Chem., 2001, 73, 1844–1848 CrossRef.
- C. Xiao, B. Lachance, G. Sunahara and J. H. T. Luong, Anal. Chem., 2002, 74, 5748–5753 CrossRef CAS.
- C. Xiao and J. H. T. Luong, Biotechnol. Prog., 2003, 19, 1000–1005 CrossRef CAS.
- K. B. Male, B. Lachance, S. Hrapovic, G. Sunahara and J. H. T. Luong, Anal. Chem., 2008, 80, 5487–5493 CrossRef CAS.
- K. B. Male, Y. K. Rao, Y.-M. Tzeng, J. Montes, A. Kamen and J. H. T. Luong, Chem. Res. Toxicol., 2008, 21, 2127–2133 CrossRef CAS.
- K. B. Male, Y.-M. Tzeng, J. Montes, B.-L. Liu, W.-C. Liao, A. Kamen and J. H. T. Luong, Analyst, 2009, 134, 1447–1452 RSC.
- Y. C. Hseu, H. L. Yang, Y. C. Lai, J. G. Lin, G. W. Chen and Y. H. Chang, Nutr. Cancer, 2004, 48, 189–197 Search PubMed.
- C. C. Peng, K. C. Chen, R. Y. Peng, C. H. Su and H. M. Hsieh-Li, Cancer Lett. (Shannon, Irel.), 2006, 243, 109–119 Search PubMed.
- H. L. Yang, C. S. Chen, W. H. Chang, F. J. Lu, Y. C. Lai, C. C. Chen, T. H. Hseu, C. T. Kuo and Y. C. Hseu, Cancer Lett. (Shannon, Irel.), 2006, 231, 215–227 Search PubMed.
- Y. L. Hsu, P. L. Kuo, C. Y. Cho, W. C. Ni, T. F. Tzeng, L. T. Ng, Y. H. Kuo and C. C. Lin, Food Chem. Toxicol., 2007, 45, 1249–1257 CrossRef CAS.
- Y. L. Hsu, Y. C. Kuo, P. L. Kuo, L. T. Ng, Y. H. Kuo and C. C. Lin, Cancer Lett. (Shannon, Irel.), 2005, 221, 77–89 Search PubMed.
- Z. M. Lu, W. Y. Tao, X. L. Zou, H. Z. Fu and Z. H. Ao, J. Ethnopharmacol., 2007, 110, 160–164 CrossRef CAS.
- D. Z. Liu, Y. C. Liang, S. Y. Lin, Y. S. Lin, W. C. Wu, W. C. Hou and C. H. Su, Biosci., Biotechnol., Biochem., 2007, 71, 23–30 CrossRef CAS.
- Y. C. Shen, Y. H. Wang, Y. C. Chou, C. F. Chen, L. C. Lin, T. T. Chang, J. H. Tien and C. J. Chou, Planta Med., 2004, 70, 310–314 CrossRef CAS.
- J. J. Chen, W. J. Lin, C. H. Liao and P. C. Shieh, J. Nat. Prod., 2007, 70(6), 989–992 CrossRef CAS.
- T. Y. Song and G. C. Yen, J. Agric. Food Chem., 2002, 50, 3322–3327 CrossRef CAS.
- C. C. Chen, Y. L. Shiao, R. D. Lin, Y. Y. Shao, M. N. Lai, C. C. Lin, L. T. Ng and Y. H. Kuo, J. Nat. Prod., 2006, 69, 689–691 CrossRef CAS.
- Z. Paryzek and J. Martynow, J. Chem. Soc., Perkin Trans. 1, 1995, 201–207 Search PubMed.
- G. T. Emmons, W. W. Wilson and G. J. Schroepfer, Jr, Magn. Reson. Chem., 1989, 27, 1012–1024 CAS.
- L. K. Kavtaradze, M. Manley-Harris and B. K. Nicholson, Steroids, 2004, 69, 227–233 CrossRef.
- L. K. Kavtaradze, M. Manley-Harris and B. K. Nicholson, Steroids, 2004, 69, 697–700 CrossRef.
- G. V. Baddeley, H. J. Samaan, J. J. H. Simes and T. Hoa Ai, J. Chem. Soc., Perkin Trans. 1, 1979, 7–14 RSC.
- R. B. Boar, J. F. McGhie and D. A. Lewis, J. Chem. Soc., Perkin Trans. 1, 1972, 2590–2592 RSC.
- Y.-Y. Li, Z.-Y. Mi, T. Tang, G. Wang, D.-S. Li and Y.-J. Tang, Helv. Chim. Acta, 2009, 92, 1586–1593 CrossRef CAS.
- S. Nakamura, J. Iwami, H. Matsuda, S. Mizuno and M. Yoshikawa, Tetrahedron, 2009, 65, 2443–2450 CrossRef CAS.
- P. M. Ghosh, C. R. Keese and I. Giaever, Bioelectrochem. Bioenerg., 1994, 33, 121–133 CrossRef.
- C. R. Keese, N. Karra, B. Dillon, A. M. Goldberg and I. Giaever, In Vitro Mol. Toxicol., 1998, 11, 183–192 Search PubMed.
- E. Majid, K. B. Male, Y.-M. Tzeng, J. O. Omamogho, J. D. Glennon and J. H. T. Luong, Electrophoresis, 2009, 30, 1967–1975 CrossRef CAS.
|
This journal is © The Royal Society of Chemistry 2010 |