DOI:
10.1039/B9AY00217K
(Paper)
Anal. Methods, 2010,
2, 149-153
Electrocatalytic oxidation of dopamine on 2,2′-[3,6-dioxa-1,8-octanediylbis(nitriloethylidyne)]-bis-hydroquinone modified carbon paste electrode
Received
12th October 2009
, Accepted 12th November 2009
First published on
23rd November 2009
Abstract
A chemically modified carbon paste electrode with 2,2′-[3,6-dioxa-1,8-octanediylbis(nitriloethylidyne)]-bis-hydroquinone (DOH) was employed to study the electrocatalytic oxidation of dopamine (DA) in buffer solution using cyclic voltammetry (CV), square wave voltammetry (SWV) and chronoamperometry (CHA). The diffusion coefficient (D = 7.4 × 10−6 cm2 s−1), and the kinetic parameter such as the electron transfer coefficient (α = 0.33) of DA oxidation at the surface of 2,2′-[3,6-dioxa-1,8-octanediylbis(nitriloethylidyne)]-bis-hydroquinone modified carbon paste electrode (DOHMCPE) was determined using electrochemical approaches. It has been found that under an optimum condition (pH 7.0), the oxidation of DA at the surface of the modified electrode occurs at a potential about 290 mV less positive than that of an unmodified carbon paste electrode. The catalytic oxidation peak currents showed a linear dependence on the DA concentration and linear analytical curves were obtained in the ranges of 3.0 × 10−5 M–2 × 10−3 M of DA with SWV. The detection limits (2σ) were determined to be 3.2 × 10−6 M. This method was also used for the determination of DA in a pharmaceutical preparation (injection) by the standard addition method.
1. Introduction
Dopamine (DA) is an important neurotransmitter molecule belonging to a family of molecules called catecholamines. It is widely distributed in the mammalian central nervous system for message transfer.1 DA has also been associated with the reward system, the circuitry in the brain responsible for the motivation to seek out stimuli as well as the emotions of feeling satisfied and satiated in one's environment.2 Deficits in brain DA cause Parkinson's disease, Alzheimer’s and schizophrenia in humans.1 Therefore, it is essential to develop simple and rapid methods for determination of this biological molecule in routine analysis. The voltammetric methods have been used for the determination of DA and other neurotransmitters. It is generally believed that direct redox reactions of these species at bare electrode are irreversible and require high overpotentials.3 Moreover, the direct redox reaction of these species often suffers from a pronounced fouling effect, which results in rather poor selectivity and reproducibility. A promising approach to overcome difficulties arising from overpotential problems and fouling properties of the biological substrate is the use of chemically modified electrodes (CMEs).4
Different modified electrodes such as polyvinyl alcohol,5 zirconium phosphated silica gel,6 poly(phenosafranine),7 cetylpyridinium bromide,8 triazole self-assembled monolayer9 and cobalt(II) hexacyanoferrate10 have been used for detection of DA in the presence of other species. Recently, much interest has centered on the use of carbon as an inexpensive substrate for electrochemical techniques. The ease and speed of preparation and of obtaining a new reproducible surface, the low residual current and low cost of carbon paste are some advantages of carbon paste electrodes (CPEs) that make them suitable substrate for electrochemical experiments.
To our knowledge, no study has reported the electrocatalytic determination of DA using a 2,2′-[3,6-dioxa-1,8-octanediylbis(nitriloethylidyne)]-bis-hydroquinone modified carbon paste electrode (DOHMCPE). Thus, in continuation of our studies concerning the preparation of chemically modified electrodes (CME),11–14 in the present work, we discuss the preparation and suitability of DOHMCPE as a new electrode for the electrocatalysis and determination of DA by CV and SWV. In order to demonstrate the catalytic ability of this modified electrode in the oxidation of DA in a real sample, we examined this ability in the voltammetric determination of DA in a pharmaceutical preparation.
![The molecular structure of 2,2′-[3,6-dioxa-1,8-octanediylbis(nitriloethylidyne)]-bis-hydroquinone](/image/article/2010/AY/b9ay00217k/b9ay00217k-s1.gif) |
| Scheme 1 The molecular structure of 2,2′-[3,6-dioxa-1,8-octanediylbis(nitriloethylidyne)]-bis-hydroquinone | |
2. Experimental
2.1. Chemical
DOH has been synthesized in our laboratory. All reagents and solvents were of the highest purity available from Merck and were used without further purification. The buffer solutions (0.1 M) were made up from H3PO4 and their salts, adjusting the pH with 0.2 M NaOH. The standard solution of the analyte was prepared daily. All solutions were prepared with doubly distilled water.
2.2 Apparatus
Electrochemical experiments were carried out using a potentiostat/galvanostat (Electroanalyzer system, SAMA 500, Iran) coupled with a personal computer for data acquisition and potential control. A three-electrode cell, including a DOHMCPE as working electrode, a platinum wire and an Ag/AgCl (saturated KCl) electrode were used as counter and reference electrodes, respectively. All potentials were measured and reported versus the Ag/AgCl reference electrode.
A 1% (w/w) modified carbon paste electrode with DOH was made up by dissolving 0.01 g of modifier in chloroform and hand mixing with 99-times it's weight of graphite powder with a mortar and pestle. Stirring evaporated the solvent. After complete evaporation of the chloroform, 3 drops (about 0.2 mL) of liquid paraffin were added to the mixture and then mixed well into a uniform paste. The resulting paste was inserted in the bottom of a glass tube. The electrical connection was implemented by a copper wire lead fitted into a glass tube. A fresh electrode surface was obtained by squeezing out a small amount of paste by piston, scrapping off the excess against a conventional paper and polishing the electrode on a smooth paper to obtain a shiny appearance.
3. Results and discussion
3.1. Electrochemical properties of DOHMCPE
As DOH is insoluble in aqueous media; therefore, we prepared DOHMCPE and studied its electrochemical properties in buffered aqueous solution (pH 7.0) using cyclic voltammetry. Cyclic voltammograms of DOH into the DOHMCPE exhibited an anodic and corresponding cathodic peak, whereas cyclic voltammograms of unmodified carbon paste electrodes in supporting electrolyte showed no anodic and cathodic peaks. Experimental results showed that DOH has well-defined and reproducible anodic and cathodic peaks (with Epa = 0.2 V, Epc = 0.125 V, E°′ = 0.162 V versus Ag/AgCl electrode and ΔEp = 0.075 V), then DOH can be used as mediator for the electrocatalysis of some important biological compounds with slow electron transfer.
As shown, the peak separation potential, ΔEp = (Epa − Epc), was greater than the 59/n mV expected for a reversible system. This result suggests that the redox couple in DOHMCPE shows quasi-reversible behavior in an aqueous medium.
In addition, the effect of the potential scan rate on electrochemical properties of the DOHMCPE was studied in an aqueous solution with cyclic voltammetry. Plots of the anodic and cathodic peak currents (Ip) were linearly dependent on ν at scan rates from 4 to 1000 mV s−1 (Fig. 1). A linear correlation obtained between peak currents and the scan rate indicates that the nature of redox process was controlled in a diffusionless manner (Fig. 1A).
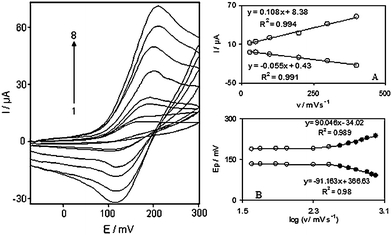 |
| Fig. 1 Cyclic voltammograms of DOHMCPE in 0.1 M phosphate buffer (pH 7.0) at various scan rates: The numbers 1–8 correspond to 4, 7, 10, 25, 50, 100, 150 and 200 mVs−1 scan rates, respectively. Insets: (A) Variations of Ip versus scan rates. (B) Variation of Ep versus the logarithm of the scan rates. | |
The apparent charge transfer rate constant, ks, and the charge transfer coefficient, α, of a surface-confined redox couple can be evaluated from cyclic voltammetric experiments and by using the variation of anodic and cathodic peak potentials with logarithm of scan rate, according to the procedure of Laviron.15Fig. 1B shows the variations of peak potentials (Ep) as a function of the logarithm of the potential scan rate. We found that the Ep values are proportional to the logarithm of the potential scan rate, for scan rates higher than 300 mV s−1 (Fig. 1B). The slopes of Fig. 1B plots can be used to extract the kinetic parameters αc (cathodic transfer coefficient) and αa (anodic transfer coefficient). The slope of the linear segment is equal to −2.303RT/αnF and 2.303RT/(1 − α)nF for the cathodic and anodic peaks, respectively. The evaluated value for the anodic transfer coefficient (αa) was 0.66.
Also, the following equation can be used to determine the electron transfer rate constant between modifier (DOH) and carbon paste electrode (CPE) for scan rates higher than 300 mV s−1:
| logks = α log (1 − α) + (1 − α) log α − log (RT/nFν) − α (1 − α) nFΔEp/2.3RT | (1) |
where (1 − α)n
α = 0.34,
v is the sweep rate and all other symbols having their conventional meanings. The value of k
s = 3.71 s
−1 was evaluated by calculation of ΔE
p (in different scan rates) and using
eq. (1).
3.2. Effect of pH
Since the voltammetric behavior of DOHMCPE is dependent on the pH value, we therefore studied the voltammetric response of the modified electrode in buffer solutions of varying pH from 2.0–12.0. The formal potential (E°′) decreased by increasing the pH value, and the slope of E°′ vs. pH was −50.45 mV/pH unit, which is very close to the anticipated Nernstian value for a two-electron two-proton process.16 Also, there was no change in the slope for pH values from 2.0 to 12.0. The peak current at pH 7.0 was higher than other pHs, therefore, we chose this pH as the optimum solution pH to carry out the voltammetric and chronoamperometric determination of DA.
3.3. Electrocatalytic oxidation of DA
The utility of the modified electrode for oxidation of DA was evaluated by cyclic voltammetry. The cyclic voltammetric responses of an unmodified carbon paste electrode in 0.1 M phosphate buffer (pH 7.0), without and with 1.0 mM DA in solution, are shown in Fig. 2 (curves a and b respectively). DOHMCPE cyclic voltammetric responses in 0.1 M phosphate buffer (pH 7.0), without and with 1.0 mM DA in solution, are shown in Fig. 2 (curves c and d respectively). DOHMCPE in 0.1 M phosphate buffer (pH 7.0), with 1.0 mM DA in solution showed a large anodic peak (without a cathodic counterpart). That the current observed is associated with DA oxidation and not with the oxidation of surface-confined DOH is demonstrated by comparing the current in Fig. 2, curve d with those in Fig. 2, curve c, which shows the cyclic voltammetric behavior of an electrode modified with DOH in a DA-free electrolyte (0.1 M phosphate buffer with pH 7.0). It is apparent that the anodic current associated with the surface attached materials is significantly less than that obtained in the solution containing DA. As can be seen, electrocatalytic activity toward DA on the modified electrode was significant (Fig. 2, curves c and d), with a defined peak potential, around 170 mV vs. Ag/AgCl electrode. Thus, a decrease in over-potential and enhancement of peak current for DA oxidation are achieved with the modified electrodes. The comparison of curves c and d (Fig. 2) shows that the anodic peak current of the mediator was greatly increased in the presence of DA over that ordinarily observed just for the DOH redox couple spiked in carbon paste electrode, while the corresponding cathodic peak disappears on the reverse scan of the potential.
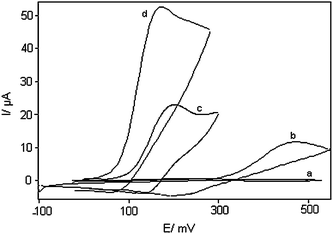 |
| Fig. 2 Cyclic voltammograms of: (a) an unmodified CPE in 0.1M phosphate buffer (pH 7.0) solution and (b) the same electrode in 1.0 mM DA, pH 7.0 solution. (c) as (a) and (d) as (b) for DOHMCPE. In all cases the scan rate was 25 mV s−1. | |
The results show that DA oxidation at an unmodified carbon paste electrode occurred irreversibly with a peak potential of 460 mV vs. Ag/AgCl (pH 7.0), while its oxidation potential at the DOH appeared at 170 mV vs. Ag/AgCl. The oxidation of DA at the surface of the DOH occurs in neutral pH (pH = 7) at a potential about 290 mV less positive than that at an unmodified carbon paste electrode. This value is more than values reported by other research groups for electrocatalytic oxidation of DA at the surface of chemically modified electrodes by other mediators (Table 1).
Table 1 Comparison of some modified electrodes used for voltammetric determination of DA
3.4. Chronoamperometric measurements
Double potential step chronoamperometry, as well as other electrochemical methods were employed to investigate electrode processes at chemically modified electrodes. Current–time curves of the DOHMCPE were obtained by setting the working electrode potential at 350 mV (at the first potential step) and 100 mV (at the second potential step) versus Ag/AgCl electrode for various concentrations of DA in a buffer solution (pH 7.0). Plots of I versus t−1/2 with the best fits for different concentrations of DA were employed. The slopes of the resulting straight lines were then plotted versus the DA concentration, from that slope and using the Cottrell equation:16 | I = nFAD1/2Cb/π1/2t1/2 | (2) |
Where D and Cb are the diffusion coefficient (cm2 s−1) and the bulk concentration (mol cm−3) respectively. We calculated a diffusion coefficient (D) of 7.4 × 10−6 cm2s−1 for DA.
3.5. Effect of scan rate on the peak current of DA
The effect of scan rate on the electrocatalytic oxidation of 1.0 mM DA at the DOHMCPE was investigated by cyclic voltammetry (Fig. 3). The oxidation peak potential shifts with increasing scan rates towards a more positive potential, confirming the kinetic limitation of the electrochemical reaction.
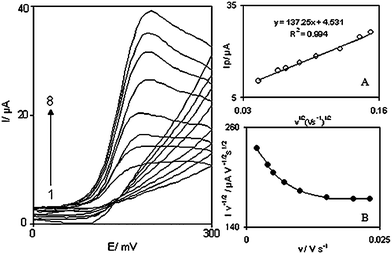 |
| Fig. 3 Cyclic voltammograms of a DOHMCPE in 0.1 M phosphate buffer (pH 7.0) containing 1.0 mM DA at different scan rates, numbers 1 to 8 correspond to 2, 6, 10, 15, 18, 23, 25 and 30 mV s−1 scan rates, respectively. Insets: (A) Variation of the electrocatalytic currents versus the square root of scan rate. (B) Variation of the scan rate normalized current (Ip/v1/2) with scan rate. | |
In order to obtain the information on the rate determining step, the Tafel plot was drawn using the data from the rising part of the linear sweep voltammogram at a scan rate of 10 mVs−1. The number of electrons involved in the rate determining step (nα) can be estimated from the slope of the Tafel plot.16 A mean slope 0.0914 V decade−1 was obtained (Not shown), indicating that a one-electron process was involved in the rate determining step. According to the Tafel slope equation and a slope of 0.0914 V decade−1 the charge transfer coefficient of DA was calculated as α = 0.33.
Also, a plot of peak height (Ip) against the square root of scan rate (v1/2), in the range of 2–30 mVs−1, was constructed (Fig. 3A), which was found to be linear, suggesting that at sufficient overpotential the process is diffusion rather than surface controlled. A plot of the sweep rate normalized current (Ip/v1/2) versus sweep rate (Fig. 3B) exhibits the characteristic shape typical of an EC′cat process.
From the slope of straight line of I versus v1/2 (Fig. 3A) and the following eq. (3),16 the number of electrons that participant in the redox process between the modifier and DA (n) was calculated:
| slope = 3.01 × 105n[(1 − α)nα]1/2ACD1/2 | (3) |
Where (1 − α)n
α = 0.67 (as obtained from the Tafel plot), A, D and C are the
electrode area (cm
2), diffusion coefficient (cm
2s
−1) and substrate concentration (mol cm
−3) respectively. The value obtained for n is 2.3 (n≅ 2).
The electrocatalytic peak current of DA oxidation at the surface of DOHMCPE can be used for determining DA in solution. Therefore, SWV experiments were performed using DOHMCPE in a phosphate buffer solution containing various concentrations of DA. SWV using modified CPE was used as a highly sensitive electrochemical method with a very low detection limit to determine trace amounts of DA. In addition, the charging current contribution to the background current, which is a limiting factor in the analytical determination, is negligible in SWV mode. Fig. 4 shows the SWVs obtained for the oxidation of different concentrations of DA at the modified CPE. The dependence of the peak current on the DA concentration is shown in Fig. 4A and 4B. The oxidation peak current of DA at the surface of MCPE was proportional to the concentration of substrate within the range 3.0 × 10−5 to 2.0 × 10−3 M. Fig. 4A and 4B clearly show that the plot of peak current versus DA concentration is constituted of two linear segments with different slopes, corresponding to two different ranges of substrate concentration. The decrease of sensitivity (slope) in the second linear range is likely to be due to kinetic limitation. From the analysis of these data, we estimate that the lower limit of detection of DA is of the order of 3.2 × 10−6 M according to the definition Cx = 2Sb/m.17
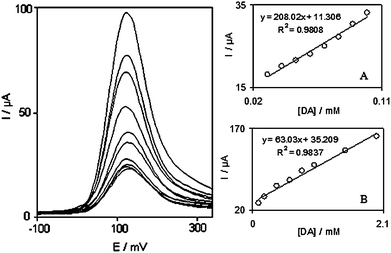 |
| Fig. 4 Square wave voltammograms of DOHMCPE in 0.1 M phosphate buffer solution (pH 7.0) containing different concentrations of DA (from inner to outer): 30.0, 50.0, 70.0, 90.0, 100.0, 200.0, 400.0, 600.0, 800.0 and 1000.0 µM. Insets show the plots of the electrocatalytic peak current as a function of DA concentration in the range of: (A) 30.0 to 100.0 µM and (B) 100.0 to 2000.0 µM. | |
3.7. Determination of DA in dopamine injection
1 mL of the DA injection solution (specified content of DA is 200 mg per 5 mL) was diluted to 50 mL with phosphate buffer, then a 0.8 mL portion of the solution was diluted in a voltammetric cell to 10 mL of 0.1 M phosphate buffer (pH 7.0). The potentials were controlled between −0.1 and 0.35 V at 25 mVs−1. Ipa was measured at the oxidation potential of DA by SWV. The average determination results of DA in the injection were 206 mg/5 mL, which were quite corresponding to the value that was given by injection specification. This experiment was repeated five times and the relative standard deviation obtained was 3.5%. Different standard concentrations of DA were added to the diluted DA injection for testing recovery. The results are shown in Table 2.
Table 2 Determination of DA in injection samples
Sample |
Content (×10−4 M) |
DA added (×10−4 M) |
DA found (×10−4 M) |
Recovery% |
1 |
3.37 |
— |
3.47 |
102.9 |
2 |
3.37 |
2.5 |
5.77 |
98.3 |
3 |
3.37 |
3.5 |
6.73 |
98 |
4 |
3.37 |
4.5 |
7.62 |
97 |
5 |
3.37 |
6.0 |
9.43 |
100.6 |
4. Conclusions
This work demonstrates the construction of a chemically modified carbon paste electrode by incorporation of DOH as the modifying species. The electrochemical behavior of the DOHMCPE has been studied by cyclic voltammetry, chronoamperometry, and square wave voltammetry. The results obtained for rate constant k, diffusion coefficient of DA and number of electrons in the rate determining step by different approaches, are in good agreement. The electrode offers the advantages of easy fabrication, fast response time, high sensitivity, a low background current and detection limit, which are suitable for routine determinations.
Acknowledgements
The authors wish to thank the Yazd University Research Council, IUT Research Council and Excellence in Sensors for financial support of this research.
References
- P. Damier, E. C. Hirsch, Y. Agid and A. M. Graybiel, Brain, 1999, 122, 1437 CrossRef.
-
J. R. Cooper, F. E. Bloom and R. H. Roth, The biochemical basis of neuropharmacology, Oxford University Press, Oxford, UK, 1982 Search PubMed.
- R. N. Adams, Anal. Chem., 1976, 48, 1126A CAS.
- M. Mazloum-Ardakani, H. Beitollahi, B. Ganjipour, H. Naeimi and M. Nejati, Bioelectrochemistry, 2009, 75, 1 CrossRef CAS.
- Y. Li and X. Lin, Sens. Actuators, B, 2006, 115, 134 CrossRef.
- E. Shams, A. Babaei, A. R. Taheri and M. Kooshki, Bioelectrochemistry, 2009, 75, 83 CrossRef CAS.
- T. Selvaraju and R. Ramaraj, Electrochem. Commun., 2003, 5, 667 CrossRef CAS.
- X. Cao, L. Luo, Y. Ding, X. Zou and R. Bian, Sens. Actuators, B, 2008, 129, 941 CrossRef.
- S. Yixin and S. F. Wang, Microchim. Acta, 2006, 154, 115 CrossRef.
- S. M. Chen and K. T. Peng, J. Electroanal. Chem., 2003, 547, 179 CrossRef CAS.
- H. Beitollahi, M. Mazloum Ardakani, B. Ganjipour and H. Naeimi, Biosens. Bioelectron., 2008, 24, 362 CrossRef CAS.
- M. Mazloum Ardakani, Z. Taleat, H. Beitollahi, M. Salavati-Niasari, B. B. F. Mirjalili and N. Taghavinia, J. Electroanal. Chem., 2008, 624, 73 CrossRef CAS.
- M. Mazloum-Ardakani, P. Ebrahimi Karami, P. Rahimi, H. R. Zare and H. Naeimi, Electrochim. Acta, 2007, 52, 6118 CrossRef.
- Z. Taleat, M. Mazloum-Ardakani, H. Naeimi, H. Beitollahi, M. Nejati and H. R. Zare, Anal. Sci., 2008, 24, 1039 CrossRef CAS.
- E. Laviron, J. Electroanal. Chem., 1979, 101, 19 CrossRef CAS.
-
A. J. Bard and L. R. Faulkner, Electrochemical Methods Fundamentals and Applications, Wiely, New York, 2001 Search PubMed.
-
D. A. Skoog, F. J. Holler and T. A. Nieman, Principles of Instrumental Analysis, 5th ed., Saunders College Publishing, 1998 Search PubMed.
- J. Tashkhourian, M. R. Hormozi Nezhad, J. Khodavesi and S. Javadi, J. Electroanal. Chem., 2009, 633, 85 CrossRef CAS.
|
This journal is © The Royal Society of Chemistry 2010 |
Click here to see how this site uses Cookies. View our privacy policy here.