DOI:
10.1039/B9AY00209J
(Paper)
Anal. Methods, 2010,
2, 143-148
Received
8th October 2009
, Accepted 21st November 2009
First published on
4th December 2009
Abstract
Amorphous ternary FeNiPt nanomaterials with tunable length are reported for constructing the electrochemical sensing platform, in which hydrogen peroxide (H2O2) is selected as a model target. It is found that amorphous FeNiPt nanostructures, in particular, the FeNiPt nanorods with large axial-ratio (FeNiPt-NRL) exhibit the enhanced electrocatalytic activity towards both the oxidation and reduction of H2O2. Meanwhile, the comparable corrosion potential Ecorr and lower corrosion current Icorr are observed at GC electrodes modified with FeNiPt-NRL, revealing the good stability of the FeNiPt-NRL surface. On the basis of these results, H2O2 is cathodically determined at glassy carbon (GC) electrodes with FeNiPt-NRL with relatively high selectivity at the appropriate potential of 0 V vs. Ag|AgCl. On the other hand, the sensitivity of the anodic H2O2 detection at the same electrode is achieved to be 2.45 mA cm−2 mM−1, which is 4-fold larger than that of the cathodic detection and those Pt nanoparticles-based H2O2 determination, and the detection limit is also developed to 40 nM. The dynamic linear range is broadened from 100 nM to 30 mM, which is wider than those H2O2 detection based on Pt nanoparticles and binary Pt alloys. In addition, electrochemical results show the high stability and good reproducibility for the present H2O2 sensor. The striking analytical performance combined with the intrinsic properties of amorphous FeNiPt nanomaterials provides a promising technique for the development of non-enzyme H2O2 and other molecule sensors with high sensitivity, broad dynamic linear range, long stability, and good reproducibility.
1. Introduction
Hydrogen peroxide (H2O2) is of great interest in connection with the development of fuel cells,1–5 nanomachines,6–8 and life sciences9–11 because H2O2 is an important intermediate of the oxygen reduction reaction, new fuel, and a product of several biological enzyme-catalyzed reactions. Therefore, it is of great importance to determine the concentration of H2O2 with high sensitivity, low detection limit, long stability, and good reproducibility. Direct electrochemical reduction and oxidation of H2O2 at conventional electrodes have been limited by the slow kinetics and surface fouling onto electrochemical devices. In addition, low sensitivity, poor reproducibility, and low stability are other limitations of using the bare and unmodified electrodes for H2O2 detection. With the development of biology, numerous electrochemical biosensors based on the use of different enzymes have been developed for the determination of H2O2.12–17 Many of the biosensors show a satisfactory sensitivity for the detection of a relatively low concentration of H2O2. However, the limitation of these enzymatic H2O2 sensors is lack of long-term stability and good reproducibility originating from the intrinsic characteristic of biomolecules. Therefore, it is desirable to improve the stability and reproducibility for H2O2 detection with enhanced sensitivity.
For this purpose, plentiful attempts have been made to develop H2O2 sensors without enzymes. In the past couple of decades, there has been a burst of research activities on nanostructured metal particles, particularly noble metal nanoparticles with controlled size and shape, because of their unique physical and chemical properties.18–20 A number of Pt nanomaterials and nanocomposites have been fabricated and widely used for the electrochemical detection of H2O2 because of the excellent stability and striking catalytic ability of Pt. Thus, nanostructured Pt-based H2O2 sensors exhibited high sensitivity, long stability, and immunity to poisoning.
However, few papers were concerned with amorphous ternary alloy nanoparticles, despite their potential applications in data storage, high performance permanent magnets, biomedicine, electrocatalytic activities, and electrochemical detection. As a matter of fact, amorphous alloy materials have attracted a lot of attention due to their superior electronic, magnetic, and chemical properties, as well as practical applications in various fields, such as powder metallurgy, magnetic recording media, ferrofluids, composite materials, and catalysis.21,22 With the development of nanomaterials, great efforts have been paid on preparation of amorphous alloy nanomaterials, which constitutes an overlapping region of two active fields full of challenge, by the combination of the unique properties of amorphous alloys and striking characteristics of nanosized materials.
In our previous work, amorphous ternary FeNiPt nanoparticles with different lengths through phase transfer method were fabricated and used for the electroanalysis of thiols.23 In the present work, the amorphous ternary FeNiPt nanoparticles were employed for constructing an electrochemical sensing platform for determination of H2O2 both anodically and cathodically, since FeNiPt nanostructures showed enhanced electrocatalytic activity towards the oxidation and reduction of H2O2. The sensitivity of the present H2O2 sensor was improved to be 2.45 ± 0.1 mA cm−2 mM−1 over the broad dynamic detection linear range of 100 nM–30 mM for FeNiPt-NRL electrode, and the detection limit was achieved to be 40 nM.
2. Experimental
2.1 Materials
Dihydrogen hexachloroplatinate (H2PtCl6·6H2O, 99%), FeCl2·4H2O (99%), NiCl2·6H2O (99%), oleic acid (C18H34O2, 99%), sodium oleate, propylene glycol (C3H8O2, 99%) were all purchased from Sinopharm Chemical Reagent Co., Ltd. Nafion solution (5 wt%) was received from Sigma and then diluted 50 times with ethanol. Phosphate buffer solution (PBS, pH 7.0) was prepared by mixed stock standard K2HPO4 and KH2PO4 solutions and pH of PBS was adjusted by pH meter. The freshly prepared H2O2 stock solution was stored in an amber glass bottle in a refrigerator (4 °C) prior to experimentation. The serum was separated from the blood by centrifuging at a speed of 4500 rpm for 30 min. Then, the serum was diluted with the same volume of 0.2 M phosphate buffer (pH 7.0) before measurements. All the solutions were prepared with Milli-Q water and were deaerated with high purity nitrogen for 30 min before experiments.
2.2 Synthesis and characterization
Ethyl alcohol absolute solution (2 ml) was mixed with FeCl2·4H2O (30 mM), NiCl2·6H2O (35 mM), and H2PtCl6·6H2O (1 mM) by ultrasound at room temperature, then added to 60 ml autoclave tube. Sodium oleate, propylene glycol, and oleic acid were added into the solution. The reaction system was sealed and heated at a designed heating rate up to 170 °C. After the reaction was cooled to room temperature, the FeNiPt nanoparticles were collected at the bottom of the container. Scanning electron microscopy (SEM) images were taken by a Quanta 200 FEG (FEI Company). XRD pattern was obtained by a D/max2550VB3+/PC X-ray diffractormeter using Cu (40 kV, 100 mA).
2.3 Electrochemical measurements
All electrochemical experiments were performed with CHI 660 and CHI 832 electrochemical workstations (CH Instrument Company). A conventional three-electrode system was adopted. The working electrode was a modified GC electrode (3 mm in diameter). KCl-saturated Ag|AgCl and a Pt coil were used as the reference and counter electrodes, respectively. All electrode potentials in the present study were referred to the Ag|AgCl reference electrode. A GC disk electrode was first polished with alumina slurries (1 and 0.05 μm) and then cleaned by sonication in Milli-Q water for 10 min. The modification of the working electrode was prepared by dropping dispersed FeNiPt nanoparticles onto the clean GC electrode by a microlitre syringe. Then 4 μL of diluted Nafion solution was dropped to form a thin film over the FeNiPt nanoparticles. The modified electrodes were finally dried in air. The FeNiPt-modified electrode was then rinsed with excessive ethanol and Milli-Q water to remove loosely bound particles. The electrodes modified with FeNiPt nanorods with large axial ration, nanorods, and nanospheres were denoted as FeNiPt-NRL/GC, FeNiPt-NR/GC, and FeNiPt-NS/GC electrodes. Before recording the voltammograms, the FeNiPt-modified surface was cleaned in N2-saturated 0.5 M H2SO4 by cycling between −0.2 and 1.3 V (vs. Ag|AgCl) until the steady CVs were observed. In continuous-flow injection system, the thin-layer radial electrochemical flow cell (BAS) consists of a thin-layer radial flow block with the FeNiPt-modified GC electrode as working electrode, stainless steel as a counter electrode, and KCl-saturated Ag|AgCl electrode as a reference electrode. PBS or H2O2/PBS were delivered from gas-impermeable syringes (BAS) and pumped through tetrafluoroethylene hexafluoropropene (FEP) tubing by a microinjection pump (CMA 100, CMA Microdialysis AB, Stockholm, Sweden) to the radial flow cell.
3. Results and discussion
3.1 Surface properties of FeNiPt nanostructures
The amorphous FeNiPt nanostructures with different lengths were prepared as previously reported.23 The morphology of as-synthesized FeNiPt nanomaterials is strongly dependent on the heating rates in this solid–liquid–solution system. As mentioned as in Experimental, the FeNiPt nanoparticles dispersion was dropped onto the carbon electrode and was confined in Nafion. Fairly dispersed large axial-ratio nanorods with the length of ∼700 nm and the diameter of ∼40 nm demonstrated in Fig. 1(A) were obtained at the heating rate of 3–8 °C min−1, while short nanorods with the length of ∼200 nm and the average diameter of ∼20 nm (Fig. 1(B)) were produced at the heating rate of 10–12 °C min−1. On the other hand, when the heating rate reached to 16–18 °C per minute it produced FeNiPt nanoparticles with the average diameter of ∼60 nm as shown in Fig. 1(C).
X-ray diffraction (XRD) pattern of FeNiPt-NRL (Fig. 1(D)) (a), FeNiPt-NR (b), and FeNiPt-NS (c) confined on GC surfaces shows the strong (111) peak at ∼40.0° and a (200) peak at ∼46.5°, corresponding to the standard face-centered cubic (fcc) structure with a random crystalline orientation.24
For testing the stability of these amorphous FeNiPt nanostructures-modified electrodes, linear sweep voltammetry was carried out for four types of electrodes: bare GC (a), FeNiPt-NRL/GC (b), FeNiPt-NR/GC (c), and FeNiPt-NS/GC (d), as demonstrated in Fig. 2. The corrosion potential Ecorr for bare GC electrodes was observed at −230 mV, Ecorr for FeNiPt-NS/GC and FeNiPt-NR/GC was observed at more negative potentials of −252 mV and −243 mV, respectively. However, the presence of FeNiPt-NRL redirected Ecorr in the opposite direction (back to −230 mV) due to the noble electrochemical characteristics of FeNiPt-NRL.
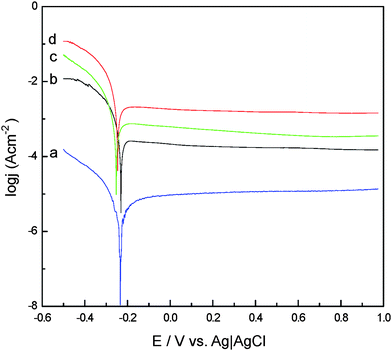 |
| Fig. 2 Polarization curves for (a) bare GC, (b) FeNiPt-NRL/GC, (c) FeNiPt-NS/GC, and (d) FeNiPt-NR/GC electrodes in 0.5 M H2SO4 at 1 mV s−1 with nitrogen bubbling. | |
The electroactive surface areas of all the electrodes could be estimated according to the Randles-Sevcik equation.25
Ip = 2.69 × 105AD1/2n3/2γ1/2C |
Where
n is the number of electrons participating in the
redox reaction,
A is the area of the
electrode (cm
2),
D is the diffusion coefficient of the molecule in solution (cm
2 s
−1),
C is the concentration of the
probe molecule in the bulk solution (mol cm
−3), and
γ is the potential scan rate (V s
−1). The surface areas of FeNiPt-NRL/
GC, FeNiPt-NR/
GC, FeNiPt-NS/
GC electrodes employed in the present work were calculated using Fe(CN)
64− solution. The roughness factor of FeNiPt-NRL/
GC, FeNiPt-NR/
GC, FeNiPt-NS/
GC electrodes was estimated from the electroactive surface area compared to the geometric one to be 2.53, 2.28, and 1.67, respectively, indicating the increased surface area of FeNiPt-modified
electrodes.
3.2 Electrocatalytic ability towards H2O2
Films of FeNiPt nanostructures modified on GC electrodes exhibited electrocatalytic activity for both reduction and oxidation of H2O2. Fig. 3 displays CVs obtained at FeNiPt-NRL/GC (a), FeNiPt-NR/GC (b), and FeNiPt-NS/GC (c) in the presence of 1 mM H2O2 (Fig. 3(A)) and in the absence (Fig. 3(B)) in PBS. For comparison, CV of H2O2 at bare GC electrode was also given curve d in Fig. 3(A). Only the small anodic and cathodic peaks corresponding to Pt oxide and reduction formation were observed at +600 mV and the range of 50–100 mV at FeNiPt-modified electrodes in the absence of H2O2, respectively (Fig. 3B), which are in good agreement with the previous reports.23 Meanwhile, no obvious currents were obtained at bare GC electrode in the presence of H2O2. However, the reduction and oxidation currents were obviously increased at FeNiPt-modified GC electrodes in the presence of H2O2 as shown in Fig. 3(A), indicating redox reaction of H2O2 was enhanced both in anodic and cathodic directions by using FeNiPt nanostructures. The reduction peak of H2O2 was obtained at around 100 mV with a peak current density of 251 μA cm−2 at the FeNiPt-NRL/GC surface, while at FeNiPt-NR/GC and FeNiPt-NS/GC films, the peak potentials were observed for H2O2 reduction at about 85 mV and 56 mV, respectively, and the peak current densities are a little smaller than that obtained at the FeNiPt-NRL/GC surface. On the anodic sweep, H2O2 oxidation occurred with an onset at around 200 mV and reached almost a plateau at 600 mV. The oxidation current increased from 52 μA cm−2 at FeNiPt-NS/GC surface to 90 μA cm−2 at FeNiPt-NRL/GC surface, revealing that the electrocatalytic ability of the present nanostructured FeNiPt surfaces towards H2O2 redox reaction increases in the order: FeNiPt-NS/GC < FeNiPt-NR/GC < FeNiPt-NRL/GC.
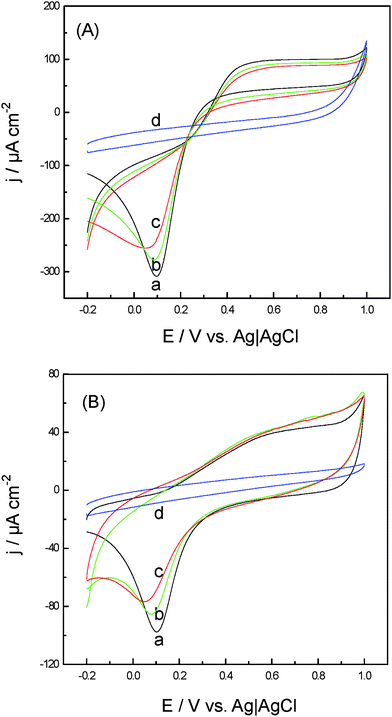 |
| Fig. 3 CVs obtained at (a) FeNiPt-NRL/GC, (b) FeNiPt-NR/GC, (c) FeNiPt-NS/GC, and (d) bare GC electrodes in 0.1 M PBS solution in the presence of H2O2 (A) and in the absence of (B) 1 mM H2O2. Potential scan rate: 20 mV s−1. | |
The enhanced electrocatalytic ability of the FeNiPt nanostructures was proposed to be attributed to the combined properties of amorphous alloys and the nanosized materials. For further understanding of the mechanism, the phase transformation from fcc to long-range chemically ordered fct was carried out by annealing the amorphous FeNiPt-NRL at 823 K for 1 h under Ar atmosphere. The fct phase of the resulted annealed FeNiPt-NRL was clearly observed from the XRD pattern (inset of Fig. S1, ESI†). The electrocatalytic ability of the annealed FeNiPt-NRL were also examined towards H2O2, compared with that of the amorphous one. From Fig. S1, ESI,† it is clear that electrocatalytic ability of the annealed FeNiPt-NRL/GC towards H2O2 (Fig. S1b, ESI†) is obviously weaker either in potential or in current density than those of the amorphous FeNiPt-NRL/GC (Fig. S1a, ESI†). These results suggested that the unique properties of amorphous alloys, i.e. the short-range order and long-range disorder in structure, together with the striking characteristic of nanosized materials, especially, the 1D nanosized materials may play the important role in the electrochemical catalysis.
As demonstrated above, amorphous FeNiPt nanostructures provided a platform for sensing H2O2 both anodically and cathodically. Fig. 4 demonstrates amperometric responses for addition of H2O2 with various concentrations at the applied potential of 0 V vs. Ag|AgCl using FeNiPt-NRL/GC electrode. The cathodic steady-state currents were obtained upon the successive addition of H2O2 from 0.6 μM to 60 mM, and the calibration curve was plotted in the inset of Fig. 4. The detection limit was developed to 200 nM and the sensitivity was estimated to be 0.13 ± 0.01 mA cm−2 mM−1. To test the selectivity of the H2O2 sensor, the interferences that may exist in food and biological samples were examined, such as ethanol, cysteine, xanthine, glucose, urine acid, ascorbic acid, dopamine, Ca2+, Mg2+, Cd2+, Fe2+, Fe3+, Mn2+, Ni2+, Pb2+, Zn2+, Cl−, SO42−, SO32−, NO3−, NO2−, and CO32−. As shown in Fig. S2, ESI,† almost no cathodic current response (< 1%) of the interferences was observed at FeNiPt-NRL/GC electrode at 0 V vs. Ag|AgCl, compared to the cathodic current of H2O2 with the same concentrations, indicating the good selectivity of this sensor at the appropriate working potential of 0 V vs. Ag|AgCl.
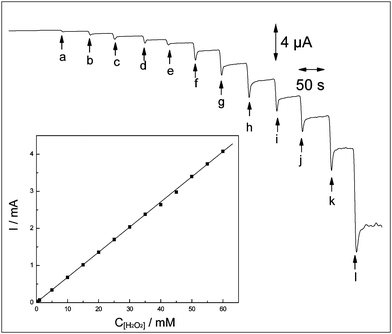 |
| Fig. 4 Amperometric responses of FeNiPt-NRL/GC electrode in 0.1 M PBS with successive addition of (a) 4 μM, (b) 8 μM, (c) 12 μM, (d) 16 μM, (e) 20 μM, (f) 40 μM, (g) 60 μM, (h) 80 μM, (i) 100 μM, (j) 120 μM, (k) 150 μM, (l) 300 μM H2O2. The solution was stirred with a magnetic stirrer at 600 rpm. Potential was kept at 0 V vs. Ag|AgCl. | |
Although the present cathodic H2O2 sensing shows good selectivity and broad detection linear range, the sensitivity is still lower than those anodic H2O2 sensors based on Pt nanoparticles reported previously.23 For this purpose, the anodic amperometric responses were examined at the positive potential of 0.45 V vs. Ag|AgCl. Fig. 5(A) shows continuous steady-state anodic currents at FeNiPt-NRL/GC electrodes polarized at 0.45 V in response to H2O2 additions in N2-saturated 0.1 M PBS (pH 7.0), and Fig. 5(B) depicts the linear calibration plots. The addition of H2O2 resulted in an apparent increase of the oxidation current. The 95% response of the steady-state current was obtained within 4 s. The current response remained constant until a new sample was injected into the electrochemical cell.
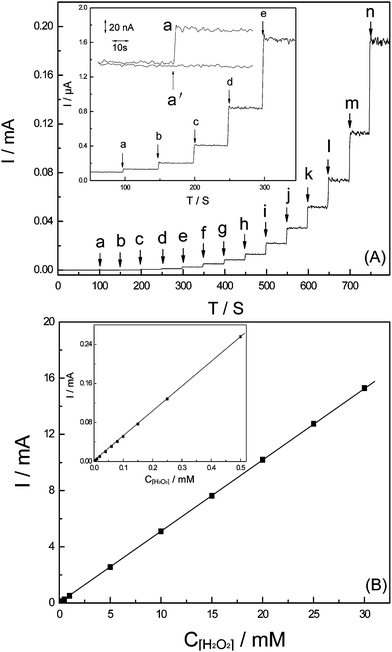 |
| Fig. 5 (A) Amperometric responses of FeNiPt-NRL/GC electrode in 0.1 M PBS with successive addition of (a) 100 nM, (b) 200 nM, (c) 500 nM, (d) 1 μM, (e) 2 μM, (f) 4 μM, (g) 6 μM, (h) 8 μM, (i) 15 μM, (j) 25 μM, (k) 35 μM, (l) 50 μM, (m) 70 μM, (n) 120 μM H2O2. Potential was kept at 0.45 V. Inset: Current responses to addition of 100 nM H2O2 in 0.1 M PBS (a) and the same volume of 0.1 M PBS without the analyte (a′); (B) Calibration plots of anodic currents against the concentration of H2O2 at FeNiPt-NRL/GC electrode. | |
An average sensitivity of 2.45 ± 0.1 mA cm−2 mM−1 with r2 = 0.9966 (n = 10) was measured over the range of 100 nM–30 mM for FeNiPt-NRL/GC electrode. The detection limit was achieved to be 40 nM estimated at an S/N ratio of 3, and for confirming this result, the same volume of 0.1 M PBS (10 μL without the analyte) was injected and caused no measurable response above background current (inset of Fig. 5a). The sensitivity over the broad detection linear range of 100 nM–30 mM is much higher than those obtained at 2.5 nm Pt nanoparticles28 estimated at 0.056 A M−1 cm−2 and at low surface density assemblies of Pt nanoparticles,29 and is comparable with that achieved at mesoporous Pt ultra-microelectrode (25 μm diameter).30 In addition, the detection linear range of the present H2O2 sensor is wider than those obtained at 2.5 nm Pt nanoparticles,28 2.5 nm polyacrylate-capped Pt nanoparticles,29 mesoporous Pt ultramicroelectrode (25 μm diameter),30 Pt nanoparticles-modified single-wall carbon nanotubes (SWCNTs).31
3.5 Reproducibility and stability
Reproducibility of the current response of the electrode was investigated in N2-saturated 0.1 M PBS (pH 7.0) solution containing 2 mM H2O2 by measuring the CVs five times every 30 min after holding the electrode in the solution. The relative standard deviation (RSD) was 1.1% (n = 5). For the interelectrode reproducibility of four electrodes in the same batch, the RSD was 3%. The stability of the electrode was investigated by storing the electrode in air for 4 weeks and then measuring the current response of 2 mM H2O2 in N2-saturated 0.1 M PBS (pH 7.0) solution. Current responses within a variation of 5% were achieved.
3.6 Continuous-flow injection
The high sensitivity of the FeNiPt-NRL/GC electrode towards the oxidation and determination of H2O2 was further confirmed by amperometric measurements in a continuous-flow system, as shown in Fig. 6. When the electrode was poised, the addition of H2O2 resulted in an apparent increase of the oxidation current. The sensitivity was 2.80 ± 0.05 mA cm−2 mM−1. When the PBS containing no analyte was pumped into the electrochemical flow cell, the current was quickly declined to the background current.
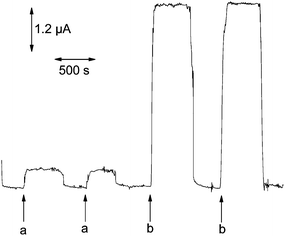 |
| Fig. 6 Typical amperometric responses of H2O2 (a 1 μM; b 10 μM) in a continuous-flow cell with the FeNiPt-NRL/GC electrode. The electrode was poised at +0.7 V. Flow rate, 3 μl min−1. | |
3.7 Real sample detection
Determination of H2O2 at the FeNiPt-NRL/GC electrode in real sample, such as serum was also tested. The human serum sample was diluted with phosphate buffer solution (pH 7.0) before the measurement. The results obtained for H2O2 in real sample are listed in Table 1. To ascertain the correctness of the results, the real samples were added with certain amounts of H2O2 at levels similar to those found in the samples themselves. The recovery rate of 3 samples was determined to be 97– 99%. The total concentrations of H2O2 in the serum samples were found to be in good agreement with previously reported results.32
Table 1 Determination of H2O2 in 3 real serum samples with the FeNiPt-NRL/GC electrode
Sample |
Detected concentration (μM) |
Added concentration (μM) |
Total concentration (μM) |
Recovery rate (%) |
1 |
39.2±3.0 |
50 |
88.0±7.5 |
98.7 |
2 |
45.4±3.3 |
50 |
93.1±7.9 |
97.6 |
3 |
43.0±3.2 |
50 |
90.7±7.7 |
97.5 |
4. Conclusion
A facile and effective electrochemical sensing technique was first developed by using amorphous FeNiPt nanoparticles with tunable length. The FeNiPt nanoparticles showed enhanced electrocatalytic activities towards both the oxidation and reduction of H2O2, and electrocatalytic activity increased in the order: FeNiPt-NS < FeNiPt-NR < FeNiPt-NRL. Meanwhile, the comparable corrosion potential Ecorr and lower corrosion current Icorr were observed at FeNiPt-NRL/GC surface, compared with those obtained at bare GC electrodes, revealing the good stability of FeNiPt-NRL surface. Subsequently, H2O2 was determined cathodically at GC electrode modified with amorphous FeNiPt-NRL with high selectivity at the appreciative potential of 0 V vs. Ag|AgCl. On the other hand, for the anodic detection of H2O2 at the same electrode, the sensitivity was achieved to 2.45 ± 0.1 mA cm−2 mM−1 in the broad dynamic linear range of 100 nM–30 mM, and the detection limit was improved to be 40 nM on the basis of the signal-to-noise ratio of 3. Meanwhile, the present FeNiPt-based H2O2 sensor also exhibited high stability and good reproducibility. This investigation opened an alternative way for determination of H2O2 and other molecules based on amorphous FeNiPt nanoparticles, as well as extending the applications of amorphous nanomaterials, especially the amorphous ternary nanomaterials to electrocatalysis, chemical sensors and biosensors, and other electrochemical purposes.
Acknowledgements
This work was financially supported by National Natural Science Foundation of China (20975057 and 20771085), the Program for New Century Excellent Talents in University (NCET-06-0380), and the project sponsored by the Scientific Research Foundation for the Returned Overseas Chinese Scholars from State Education Ministry, China, and Nanometer Science Foundation of Shanghai (0952nm04900).
Notes and references
- V. R. Stamenkovici, B. S. Mun, M. Arenz, K. J. J. Mayrhofer, C. A. Lucas, G. Wang, P. N. Ross and N. M. Markovici, Nat. Mater., 2007, 6, 241 CrossRef CAS
.
- M. S. El-Deab and T. Ohsaka, Angew. Chem., Int. Ed., 2006, 45, 5963 CrossRef CAS
.
- H. Yano, E. Higuchi, H. Uchida and M. Watanabe, J. Phys. Chem. B, 2006, 110, 16544 CrossRef CAS
.
- P. Yu, J. Yan, H. Zhao, L. Su, J. Zhang and L. Mao, J. Phys. Chem. C, 2008, 112, 2177 CrossRef CAS
.
- M. Hu, Y. Lu, S. Zhang, S. Guo, B. Lin, M. Zhang and S. Yu, J. Am. Chem. Soc., 2008, 130, 11606 CrossRef CAS
.
- G. A. Ozin, I. Manners, S. Fournier-Bidoz and A. Arsenault, Adv. Mater., 2005, 17, 3011 CrossRef CAS
.
- K. Demirok, R. Laocharoensuk, K. M. Manesh and J. Wang, Angew. Chem., Int. Ed., 2008, 47, 9349 CrossRef CAS
.
- M. Sakamoto, T. Tachikawa, M. Fujitsuka and T. Majima, Adv. Funct. Mater., 2007, 17, 857 CrossRef CAS
.
- S. G. Rhee, Science, 2006, 312, 1882 CrossRef
.
- Z. A. Wood, L. B. Poole and P. A. Karplus, Science, 2003, 300, 650 CrossRef CAS
.
- J. P. Collman, L. Fu, P. C. Herrmann and X. Zhang, Science, 1997, 275, 949 CrossRef CAS
.
- A. Liu, M. Wei, I. Honma and H. Zhou, Anal. Chem., 2005, 77, 8068 CrossRef CAS
.
- P. Xia, H. Liu and Y. Tian, Biosens. Bioelectron., 2009, 24, 2470 CrossRef CAS
.
- H. Liu, Y. Tian and Z. Deng, Langmuir, 2007, 23, 9487 CrossRef CAS
.
- Y. Luo, H. Liu, Q. Rui and Y. Tian, Anal. Chem., 2009, 81, 3035 CrossRef CAS
.
- A. Zhu, Y. Tian, H. Liu and Y. Luo, Biomaterials, 2009, 30, 3183 CrossRef CAS
.
- Z. Deng, Y. Gong, Y. Luo and Y. Tian, Biosens. Bioelectron., 2009, 24, 2465 CrossRef CAS
.
- M. C. Daniel and D. Astruc, Chem. Rev., 2004, 104, 293 CrossRef CAS
.
- Y. Tian, H. Liu and Z. Deng, Chem. Mater., 2006, 18, 5820 CrossRef CAS
.
- Y. Tian, H. Liu, G. Zhao and T. Tatsuma, J. Phys. Chem. B, 2006, 110, 23478 CrossRef CAS
.
- M. Wen, Y. Wang, F. Zhang and Q. Wu, J. Phys. Chem. C, 2009, 113, 5960 CrossRef CAS
.
- Y. Wang, X. Ai and H. Yang, Chem. Mater., 2004, 16, 5194 CrossRef CAS
.
- M. Wen, H. Liu, F. Zhang, Y. Zhu, D. Liu, Y. Tian and Q. Wu, Chem. Commun., 2009, 4530 RSC
.
- H. Yano, M. Kataoka, H. Yamashita, H. Uchida and M. Watanabe, Langmuir, 2007, 23, 6438 CrossRef CAS
.
-
A. J. Bard and L. R. Faulkner, Electrochemical Methods-Fundamentals and pplications; John Wiley and Sons: New
York, 2000 Search PubMed
.
- J. Wang, D. F. Thomas and A. Chen, Anal. Chem., 2008, 80, 997 CrossRef CAS
.
- Y. Sun, H. Buck and T. E. Mallouk, Anal. Chem., 2001, 73, 1599 CrossRef CAS
.
- T. You, O. Niwa, M. Tomita and S. Hirono, Anal. Chem., 2003, 75, 2080 CrossRef CAS
.
- P. Karam and L. I. Halaoui, Anal. Chem., 2008, 80, 5441 CrossRef CAS
.
- S. A. G. Evans, J. M. Elliott, L. M. Andrews, P. N. Bartlett, P. J. Doyle and G. Denuault, Anal. Chem., 2002, 74, 1322 CrossRef CAS
.
- S. Hrapovic, Y. Liu, K. B. Male and J. H. T. Luong, Anal. Chem., 2004, 76, 1083 CrossRef CAS
.
- J. Smielecki, A. Wykretowicz, A. Minczykowski, M. Kazmierczak and H. Wysocki, Int. J. Cardiol., 1996, 56, 137 CAS
.
Footnote |
† Electronic supplementary information (ESI) available: CV of H2O2 at annealed FeNiPt-NRL and the affect of some unusual interferences at 0 V. See DOI: 10.1039/b9ay00209j |
|
This journal is © The Royal Society of Chemistry 2010 |