DOI:
10.1039/B916511H
(Paper)
Analyst, 2010,
135, 104-110
Received
11th August 2009
, Accepted 21st October 2009
First published on
2nd November 2009
Abstract
This study reports the development of a simple, sensitive, and selective-detection system for homocysteine (HCys) based on the combination of fluorosurfactant-capped gold nanoparticles (FSN-AuNPs) and o-Phthaldialdehyde (OPA). The proposed assay utilizes FSN-AuNPs as extractors for HCys and cysteine (Cys), which can then be collected by centrifugation. As long as the HCys and Cys are isolated from the initial sample, they can be liberated from the NP surface by 2-mercaptoethanol (2-ME). The derivatization of released HCys with OPA/2-ME has a strong fluorescence maximum at 485 nm, whereas the derivatization of released Cys with the same reagent shows an extremely weak fluorescence maximum at 457 nm. As a result, the selectivity of this system is more than 100-fold for HCys over any aminothiols when excited at 370 nm. The extraction and derivation efficiencies are monitored as functions of the concentration of FSN-AuNPs and OPA, respectively. The proposed system has a detection limit of 180 nM at a signal-to-noise ratio of 3 for HCys. This study validates the applicability of this system by analyzing the amount of HCys in urine samples.
Introduction
HCys is a high risk factor for Alzheimer's disease1 and cardiovascular disease.2 As such, the analysis of homocysteine (HCys) levels in plasma and urine is of continuing interest to researchers. In humans, most of the HCys synthesized each day is converted to cysteine (Cys) and methionine under the control of a specific enzyme. Normal concentrations of HCys in plasma and urine range from 6.7 to 14.5 μM and 1.4 to 0.6 mol mmol−1 creatinine, respectively.3 A plasma HCys concentration greater than 15 μM is termed as hyperhomocysteinemia, which has been classified as moderate (15–30 μM), intermediate (30–100 μM), and severe (> 100 μM).4 A high level of HCys can cause a variety of adverse health effects such as neural tube defects,5 osteoporosis,6 and cancer.7 Thus, clinical diagnosis requires the routine analysis of HCys in biological fluid.
Current methods for determining HCys levels include gas chromatography,8 high performance liquid chromatography,9 and capillary electrophoresis.10 These separation techniques are commonly coupled with laser-induced fluorescence, mass spectrometry, or electrochemical detection. However, all of these methods are rather complicated, time-consuming, and costly. To overcome these problems, researchers have proposed numerous chemosensors for the simple, rapid, and sensitive detection of HCys.11–14 A series of dyes containing the aldehyde group is capable of sensing HCys or Cys based on a ring formation of thiazinane or thiazolidine.11 Adduct formation results in fluorescence quenching or enhancement and color change. For example, researchers have used a xanthene dye containing the aldehyde group to recognize Cys or HCys based on fluorescence quenching.11b Following a similar strategy, selective fluorescent recognition of HCys has been accomplished by selective reaction of the aldehyde group of an iridium complex with HCys.12 Strongin's group introduced dication methyl viologen for sensing HCys in an organic/aqueous solution.13 A selective fluorescence turn-on sensor for the intracellular imaging of Cys and HCys has also been accomplished using a highly electron-deficient system.14 However, the methods mentioned above suffer from sophisticated synthesis, poor aqueous solubility, cross-sensitivity toward Cys, and/or poor sensitivity.
Recently, researchers have used gold nanoparticles (AuNPs) as an alternative for the detection of aminothiols.15 When aminothiols attach to the AuNPs via the formation of Au–S bonds, the AuNPs become aggregated. This is mainly because of electrostatic interaction and hydrogen bonding between aminothiol-capped AuNPs. The aggregated AuNPs lead to a decrease in surface plasmon resonance (SPR) peak at around 520 nm and the formation of a long wavelength band (∼630 nm). As a result, aminothiols can be quantified by monitoring the extinction ratio between dispersed and aggregated NPs. Moreover, AuNPs provide a tremendously high extinction coefficient, over 1000 times higher than that of organic dyes.16 The fluorescence of organic dyes adsorbed on the surface of the AuNPs is severely quenched by energy-transfer and/or charge-transfer processes.17 The presence of aminothiols removes the organic dyes from the NP surface via the formation of Au–S bonds. As a result, the organic dyes restore their fluorescence.15b,15c Although the methods above have high aminothiol sensitivity, they are not selective with respect to specific aminothiols. To achieve better selectivity, researchers have used citrate-capped AuNPs modified with nonionic fluorosurfactant (Zonyl FSN) for selective detection of Cys and HCys.18 The sensing of Cys can be successfully achieved using FSN-capped AuNPs (FSN-AuNPs) after aminothiols have been pretreated with NaOH.19a This may be due to the fact that HCys forms a five-membered ring under the pretreatment of NaOH, thereby decreasing the rate of the aggregation of FSN-AuNPs. Moreover, when the particle size of FSN-AuNPs changes from 12 to 40 nm, the NP aggregation induced by HCys is faster than that induced by Cys.19b Thus, under optimum reaction time, 40-nm FSN-AuNPs enable the selective detection of HCys. However, using 40-nm FSN-AuNPs as an HCys sensor reveals a narrow linear range (0.2–2.5 μM) for the quantification of HCys because the molar ratio of HCys to Cys determines the aggregation of 40-nm FSN-AuNPs.
This study presents a technique for highly selective and sensitive detection of HCys using FSN-AuNPs as an extracting agent and o-Phthaldialdehyde (OPA) as a derivatizing agent. FSN-AuNPs are capable of selectively extracting HCys and Cys from an aqueous solution. 2-Mercaptoethanol (2-ME) removes HCys and Cys from the surface of the AuNPs through ligand exchange.20 Compared to HCys, the derivatization of Cys with OPA/2-ME suffers from a low reaction rate21 and low fluorescence quantum yield.22 Moreover, the excitation and emission wavelengths of OPA/2-ME-derivatized HCys center at 370 and 485 nm, respectively, which are entirely different from the excitation and emission maxima of OPA/2-ME-derivatized Cys centered at 340 and 457 nm. Based on these results, we assume that OPA/2-ME could be used to selectively detect HCys in a mixture of HCys and Cys, which has been extracted by FSN-AuNPs. All important parameters were carefully evaluated, including the pH of the solutions and the concentration of FSN-AuNPs, on the sensing of HCys. To demonstrate the practicality of this approach, this study uses it to determine the total HCys in a urine sample.
Experimental procedures
Chemicals
HCys, Cys, glutathione (GSH), γ-glutamylcysteine (Glu-Cys), cysteinylglycine (Cys-Gly), histidine, 2-ME, trisodium citrate, hydrogen tetrachloroaurate(III) dehydrate, sodium hydroxide, OPA, FSN, H3PO4, NaH2PO4, Na2HPO4, Na3PO4, poly(diallydimethylammonium chloride) (PDDAC; 20 wt% in water; MW 400
000–500
000), and tris(2-carboxyethyl)phosphine (TCEP) were obtained from Sigma-Aldrich (St. Louis, MO, USA). The molecular formula of FSN is F(CF2CF2)3–8CH2CH2O(CH2CH2O)xH. Water used in all experiments was doubly distilled and purified by a Milli-Q system (Millipore, Milford, MA, USA).
Apparatus
The extinction spectra of the FSN-AuNPs were recorded using a double-beam UV-vis spectrophotometer (Cintra 10e; GBC, Victoria, Australia). A H7100 transmission electron microscopy (TEM) (Hitachi High-Technologies Corp., Tokyo, Japan) operating at 75 keV was used to collect TEM images of the FSN-AuNPs. The average diameter of the AuNPs was calculated using ImageJ software (http://rsb.info.nih.gov/ij/). The fluorescence spectra of OPA/2-ME-derivatized analytes were obtained using a Hitachi F-7000 fluorometer (Hitachi, Tokyo, Japan). The fluorescence images were recorded using a Coolpix 5400 digital color camera (Nikon, Tokyo, Japan).
We prepared citrate-capped AuNPs by means of the chemical reduction of a metal salt precursor (hydrogen tetrachloroaurate, HAuCl4) in the liquid phase.23 To achieve this, we rapidly added 38.8 mM of trisodium citrate (40 mL) to a solution of 1-mM HAuCl4 (400 mL) that was heated under reflux. This heating continued for an additional 15 min, during which time the color of the solution changed to a deep red. TEM images (not shown) confirmed that the diameter of the AuNPs is 13.1 nm, with a standard deviation of 1.1 nm. The concentration of spherical AuNPs was estimated by using the following equation:24
where n is the number of AuNPs, m is the molar mass of Au in the substance, r is the radius of the AuNPs, and s is the specific gravity of AuNPs (19.3 g cm−3). The values of m and r were obtained by conducting inductively coupled plasma mass spectroscopy (Perkin Elmer-SCIEX, Thornhill, ON, Canada) and TEM measurements, respectively. Based on this result, we estimated the concentration of the particles in the solution to be 2.57 nM (1.55 × 1012 particles/mL). A solution of the FSN-AuNPs was prepared upon the addition of 240 μL of 10% FSN to 60 mL of 2.57 nM citrate-capped AuNPs. The resulting mixture was stored at 4 °C until further use. Although FSN-AuNPs can not be reused after thiol extraction, the cost for 60 mL of 2.57 nM FSN-AuNPs is only ca. US$ 0.61. In this study, 2.57 nM FSN-AuNPs denotes 1.0×.
We prepared all standard solutions (1.0 mM) in deionized water and then added 500 μL of 10.0 μM aminothiols to 500 μL of FSN-AuNPs (2.0–24.0×) or citrate-capped AuNPs (2.0–24.0×), which has been prepared in 80 mM phosphate solution at pH range of 13.0. The mixtures were equilibrated at ambient temperature for 30 min and then centrifugated at 17000 rpm for 10 min. Subsequently, the supernatant was carefully removed up to a residual volume of 10 μL. The precipitates were resuspended in a fresh prepared solution of 2-ME (5.0–200.0 mM; 100 μL) in order to liberate the extracted aminothiols from the Au surface. After 1 h, the released aminothiols were separated from the precipitates by centrifugation at 17000 rpm for 10 min. The resulting supernatant was placed in another 1.5-mL tube and then derivatized with a solution containing OPA and NaOH. The final concentrations of OPA and NaOH were 10.0–1000.0 μM and 0.1–1.4 M. After 0–40 min, the fluorescence spectra of OPA-derivatized aminothiols were measured using a fluorometer when excited at 370 nm
In the quantitative analysis, we added 500 μL of aminothiols (1.2–40.0 μM) to 500 μL of 40.0× FSN-AuNPs, which has been prepared in 80 mM phosphate at pH 13.0. After 30 min, the resulting solutions were centrifugated at 17000 rpm for 10 min and then removed up to a residual volume of 10 μL. Subsequently, the precipitates were resuspended in 100 μL of 100.0 mM 2-ME. After 1 h, the supernatant were obtained by centrifugation at 17000 rpm for 10 min and then derivatized with a solution containing OPA and NaOH for 2 min. The final concentrations of OPA and NaOH were 1.0 mM and 0.6 M. The fluorescence spectra of OPA-derivatized aminothiols were recorded using a fluorometer when excited at 370 nm.
Detection of total amount of HCys in urine
Urine samples were collected from a healthy female. To determinate the total concentration of HCys in a urine sample, we added 10 μL of 100.0 mM TCEP to 100 μL urine (Note that TCEP can efficiently reduce HCys disulfide to generate HCys). The mixtures were equilibrated at ambient temperature for 10 min. We then prepared a series of samples by “spiking” them with standard solutions (100 μL) of HCys in the range of 1.0 to 10.0 μM. Subsequently, we added these spiked samples (500 μL) to a solution (500 μL) containing 40.0× FSN-AuNPs and 80 mM phosphate (pH 13.0). The following steps were the same as those described above in the quantification of standard solution of HCys. On the other hand, the concentration of creatinine in urine was measured by a TBA-200 FR automatic analyzer (Toshiba, Tokyo, Japan).
A commercial UV–vis absorbance detector (ECOM, Prague, Czech Republic) was used to detect aminothiols while the detection wavelength was set at 200 nm. Electrophoresis was driven by a high-voltage power supply (Bertan, Hicksville, NY). The high-voltage end of the separation system was put in a laboratory-made plexiglass box for safety. Data acquisition (10 Hz) and control were conducted by the use of DataApex Software (DataApex, Prague, Czech Republic). The fused-silica capillaries (Polymicro Technologies, Phoenix, AZ) were 60-cm long and had an i.d. of 75-μm (effective length: 40 cm). Prior to analysis, capillaries were treated with 1.2% v/v PDDAC overnight, resulting in reversed electroosmotic flow. Before conducting separations, the capillary was filled with 1.2% v/v PDDAC by syringe pumping (KD scientific, New Hope, PA) at a flow rate of 1 μL min−1. Note that PDDAC has been prepared in 5.0 mM phosphate buffer at pH 1.0. Subsequently, the released aminothiols were injected by hydrodynamic injection at 20-cm height for 60 s. All separations were performed at 170 V cm−1.
Results and discussion
Extraction of HCys by FSN-AuNPs
Scheme 1 shows that the AuNP-based approach for sensing HCys can be divided into four major steps. First, the FSN-AuNPs selectively capture HCys and Cys. Second, after centrifugation and washing, HCys and Cys attached to the NP surface are isolated from a mixture of analytes. Third, additional 2-ME removes HCys and Cys from the surface of the AuNPs through ligand exchange between 2-ME and aminothiols.20 Finally, a solution of OPA and 2-ME selectively detects HCys in the presence of Cys when excited at 370 nm. Note that the fluorescence excitation and emission spectra of OPA/2ME-derivatized HCys are entirely different from that of OPA/2-ME-derivatized Cys (Fig. S1, ESI†). Table 1 shows that the reaction of Cys with OPA/2-ME exhibits extremely weak fluorescence22 compared to HCys, GSH, and His. Additionally, a previous study reports that the reaction rate between Cys and OPA/2-ME is extremely slow.21 These results and previous studies suggest that Cys does not interfere with the determination of HCys when derivatized with OPA/2-ME (excited at 370 nm). To test whether or not this approach is capable of sensing HCys, an experiment in this study utilized 1.0× FSN-AuNPs to selectively extract 10.0 μM HCys from a 40 mM phosphate solution (pH 13.0), and then used 0.1 M 2-ME to liberate the covalently attached HCys from the Au surfaces. The released HCys was derivatized with a solution of 0.1 M NaOH and 0.1 mM OPA. The fluorescence spectrum of standard HCys derivatized with OPA/2-ME exhibits an emission maximum at 485 nm when excited at 370 nm (spectrum a in Fig. 1A).25 The released HCys derivatized with OPA/2-ME reveal a similar fluorescence feature (spectrum b in Fig. 1A), demonstrating that HCys detection can be conducted by combining FSN-AuNPs extraction and OPA/2-ME derivatization. Nevertheless, the fluorescence intensity of released HCys is lower than that of standard HCys. This is probably because the concentration of FSN-AuNPs is insufficient for the effective extraction of HCys. To support this hypothesis, the supernatant isolated from the centrifugation of a solution of FSN-AuNPs and HCys was also reacted with OPA/2-ME. The strong fluorescence intensity of the OPA derivative of the supernatant reveals that a large number of HCys molecules remained in the supernatant (Spectrum c in Fig. 1A). Note that 1.0× FSN-AuNPs can only extract 24.5% of the HCys from an aqueous solution. Because the concentration of 2-ME (0.1 M) is tremendously greater than that of HCys (10.0 μM), slow ligand exchange between HCys and 2-ME on the surface of the AuNPs is impossible.
Table 1 Relative fluorescence intensities (RFI%) of HCys and related compounds derivatized with 0.1 mM OPA in the presence of 0.1 M 2-ME
|
RFIa |
Standard deviation (%) |
Excitation at 370 nm.
|
HCys |
100.0 |
3.5 |
Cys |
0.4 |
2.1 |
GSH |
63.8 |
2.6 |
Glu-Cys |
177.8 |
4.6 |
Cys-Gly |
0.1 |
1.9 |
His |
89.3 |
3.4 |
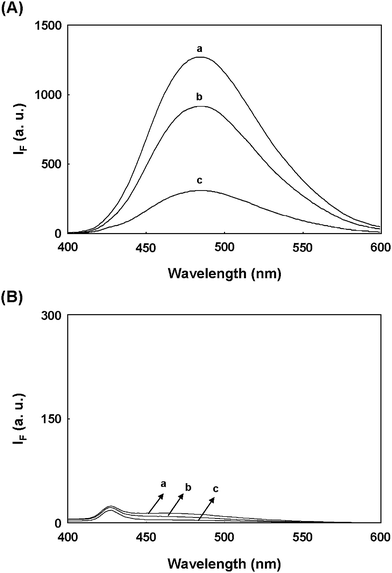 |
| Fig. 1 Comparison of fluorescence spectra of OPA/2-ME-derivatized (A) HCys and (B) Cys. The supernatant and precipitate were obtained by the centrifugation of a solution containing 1.0× FSN-AuNPs and 10.0 μM aminothiols. To liberate aminothiols adsorbed on the NP surface, the precipitate was resuspended in a solution of 0.1 M 2-ME. The released aminothiols were isolated from the precipitate by the centrifugation. (a) standard aminothiols, (b) supernatant, and (c) released aminothiols were derivatized with a solution containing 0.1 mM OPA and 0.1 M NaOH for 10 min. FSN-AuNPs were prepared in 40 mM phosphate solution at pH 13.0. The excitation wavelength was set at 370 nm. | |
Next, under identical extraction conditions, another experiment examined the fluorescence spectra of standard Cys, released Cys, and the supernatant (from the centrifugation of a solution of FSN-AuNPs and Cys) when derivatized with OPA/2-ME. The OPA/2-ME-derivatized standard Cys had an extremely low fluorescence intensity (Spectrum a in Fig. 1B), which may be the result of OPA reagent. Similar fluorescence spectra appear in the case sis of released Cys and the supernatant (Spectrum b and c in Fig. 1B). These results show that OPA/2-ME can be used to discriminate HCys from Cys when excited at 370 nm. A further experiment applied CE to examine whether or not FSN-AuNPs can be used to extract HCys and Cys. After extraction and 2-ME liberation, 2-ME released-aminothiols were directly separated by CE with UV absorbance. The electrophoregram a in Fig. S2 (ESI†) shows three peaks corresponding to 2-ME, FSN, and HCys in the presence of 1.2% PDDAC. With the exception of the Cys migration time, a similar electrophoregram was obtained for released Cys (electrophoregram b in Fig. S2, ESI†). Obviously, these results not only demonstrate the ability of FSN-AuNPs to extract HCys and Cys, but also support the selectivity of OPA/2-ME reagent to HCys.
Effect of the concentration of FSN-AuNPs
The results above imply that a higher concentration of FSN-AuNPs is required to extract 1.0 mL of 10.0 μM HCys. Thus, this study investigates the effect of the concentration of FSN-AuNPs on the extraction efficiency of HCys. Fig. 2 shows that the fluorescence intensity (485 nm) of released HCys derivatized with OPA/2-ME gradually increases as the concentration of FSN-AuNPs increases and reaches a plateau at 7.0× AuNPs. This implies that 7.0× FSN-AuNPs are capable of completely extracting 1.0 mL of 10.0 μM HCys. Accordingly, a single FSN-AuNP (13-nm diameter) can adsorb approximately 560 HCys molecules. This study also examines whether or not the remaining aminothiols (Cys, GSH, Glu-Cys, and Cys-Gly) have the same effect under identical extraction and derivatization conditions. If the extraction of GSH and Glu-Cys by FSN-AuNPs is successful, strong fluorescence signal should appear through OPA/2-ME derivatization. However, when the concentration of FSN-AuNPs increases from 1.0 to 12.0×, the fluorescence intensity for all tested aminothiols remains low (Fig. 2). This implies that FSN-AuNPs have extremely low affinity with GSH and Glu-Cys due to steric effects. Previous studies report the same results.18,19 Moreover, because the derivatization efficiency of Cys-Gly and Cys with OPA/2-ME is extremely poor, the interferences of Cys-Gly and Cys for this approach are negligible. According to these results, the concentration of FSN-AuNPs for the complete extraction of 1.0 mL of 10.0 μM HCys is larger than 7.0×. To show the advantage of FSN-AuNPs over citrate-capped AuNPs, the extraction of each aminothiol was conducted using different concentrations of citrate-capped AuNPs. Fig. S3 (ESI†) shows that the fluorescence intensity (485 nm) of released HCy derivatized with OPA/2-ME randomly changes as the concentration of citrate-capped AuNPs increases. Similar results were observed in the case of GSH and Glu-Cys. This may be attributed to that the fact that high concentration of citrate-capped AuNPs was easily aggregated in a high ionic strength solution. More importantly, this result also indicates that citrate-capped AuNPs were not selective to HCys.
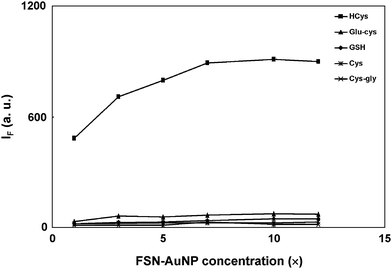 |
| Fig. 2 Effect of the concentration of FSN-AuNPs on the fluorescence intensity at 485 nm of released aminothiols derivatized with OPA/2-ME. The precipitate was collected by the centrifugation of a solution containing 10.0 μM aminothiols and 1.0–12.0× FSN-AuNPs. The extraction and derivatization conditions are the same as those in Fig. 1. FSN-AuNPs were prepared in 40 mM phosphate solution at pH 13.0. The excitation wavelength was set at 370 nm. | |
Selectivity, sensitivity, and application
This study examines the derivatization conditions for released HCys in terms of fluorescence intensity and reaction time. Fig. S4 (ESI†) shows that the optimal concentrations of NaOH and OPA for the derivatization of released HCys are 0.6 M and 1.0 mM, respectively. Under optimum extraction and derivatization conditions, we evaluated the selectivity of our new approach for HCys over aminothiols (Cys, GSH, Cys-Gly, and Glu-Cys) and histidine. Since the precipitate (i.e., analyte-attached AuNPs) is washed twice with distilled water, the pH of the solution in the extraction procedure does not interfere with the derivatization of analyte with OPA/2-ME. Moreover, the FSN-AuNPs concentration was increased to 10.0× to ensure the complete extraction of HCys. Fig. 3 indicates that the combination of FSN-AuNPs extraction and OPA/2-ME derivatization provides high selectivity for HCys over other aminothiols and histidine; Fig. 3 also suggests that the selectivity of this approach is more than 100-fold for HCys over other analytes.
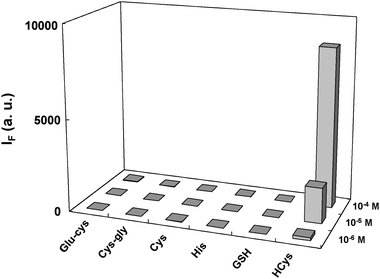 |
| Fig. 3 Fluorescence intensity at 485 nm of 2-ME released analytes derivatized with OPA/2-ME. The precipitate was collected by the centrifugation of a solution containing 1.0–100.0 μM analytes and 10.0× FSN-AuNPs. To liberate analytes adsorbed on the NP surface, the precipitate was resuspended in a solution of 0.1 M 2-ME. The released analytes were isolated from the precipitate by the centrifugation and then derivatized with a solution containing 1.0 mM OPA and 0.6 M NaOH for 2 min. FSN-AuNPs were prepared in 40 mM phosphate solution at pH 13.0. The excitation wavelength was set at 370 nm. | |
The proposed method quantifies HCys by monitoring the fluorescence intensity (485 nm) of OPA-derivatized HCys after extracting various concentrations of HCys with 20.0× FSN-AuNPs. Note that the FSN-AuNPs concentration increases to 20.0× to expand the dynamic range of the measurable concentration. As Fig. 4 indicates, the fluorescence intensity at 485 nm increases as the concentration of released HCys increases. The inset in Fig. 4 shows a linear correlation between the fluorescence intensity (485 nm) and the concentration of HCys range from 0.6–40.0 μM. The limit of detection (LOD) is 180 nM at a signal-to-noise ratio of 3 for HCys, which is below the maximum permissible limit of HCys in a healthy individual.4 Compared to the use of 40-nm FSN-AuNPs for the detection of HCys19 the proposed method provides higher sensitivity and larger linear range for the determination of HCys.
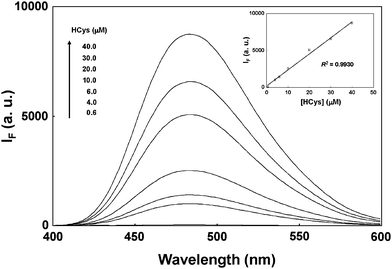 |
| Fig. 4 The quantification of HCys by the combination of 20.0× FSN-AuNPs extraction and OPA/2-ME derivatization. Inset: A plot of fluorescence intensity at 485 nm as a function of the HCys concentration. The concentration of FSN-AuNPs was 20.0×. The error bars represent standard deviations based on five independent measurements. The other extraction and derivatization conditions are the same as those in Fig. 3. | |
This study further applies this highly sensitive and selective approach to the practical analyses of total amount of HCys in urine samples. Although urine samples contain many other aminothiols (Cys, GSH, and Cys-Gly), the interferences of GSH and Cys-Gly are insignificant after pretreating urine samples with FSN-AuNPs.26 Moreover, the quantum yield of Cys derivatized with OPA/2-ME is extremely low and the excitation maximum of Cys is totally different from that of HCys. Thus, the sensing of total HCys using this approach should be straightforward. To reduce HCys disulfide to HCys, the urine samples were pretreated with a reducing agent, TCEP. Fig. 5 shows an apparent increase in the fluorescence intensity at 485 nm after urine samples were spiked with standard solutions containing different concentrations of HCys. Plotting the fluorescence intensity at 485 nm against the concentration of spiked HCys produces a linear (R2 = 0.9999) calibration curve. Standard addition shows that the concentration of total amount of HCys in urine is 0.81 ± 0.12 mmol mol−1 creatinine (n = 3). Note that the concentration of creatinine in the urine samples was 12.6 mM. The concentration ranges obtained in this study are in good agreement with those reported in the literature,3,26 suggesting that this approach is suitable for routine urine assays in clinical studies.
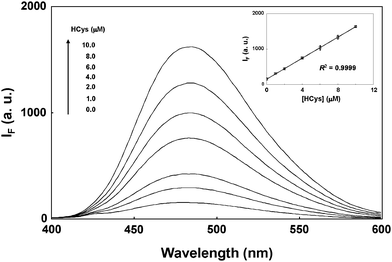 |
| Fig. 5 The quantification of total amount of HCys in urine by the combination of 0.0× FSN-AuNPs extraction and OPA/2-ME derivatization. Urine samples were spiked with standard solutions containing 0, 1.0, 2.0, 4.0, 6.0, 8.0, 10.0 HCys. Inset: A plot of fluorescence intensity at 485 nm as a function of the HCys concentration. The precipitate was obtained by centrifugation of a solution containing spiked-urine samples and 20.0× FSN-AuNPs. The error bars represent standard deviations based on five independent measurements. The other extraction and derivatization conditions are the same as those in Fig. 3. | |
Conclusion
This study reports a new assay for the selective and sensitive detection of HCy using FSN-AuNPs for selective extraction of HCys and Cys and OPA/2-ME for selective derivatization of HCys, respectively. Compared to previous studies on sensing HCys,11–15,18,19 the proposed method has the distinct advantages of high sensitivity (LOD = 180 nM), greater linear range (0.6–40.0 μM), greater selectivity (over 100-fold), and simplicity (easy preparation of FSN-AuNPs). In the opinion of the authors, the sensitivity of this method should be further improved by using FSN-AuNPs to extract the large sample volume. Moreover, we believe that the present approach holds great potential for the analysis of HCys thiolactone25 and protein-bound HCys.4a
Acknowledgements
We would like to thank National Science Council (NSC 96-2113-M-110-008-) and National Sun Yat-sen University-Kaohsiung Medical University Joint Research Center for the financial support of this study. We also thank National Sun Yat-sen University and Center for Nanoscience & Nanotechnology for the measurement of fluorescence spectrum.
References
- H. Refsum, P. M. Ueland, O. Nygård and S. E. Vollset, Annu. Rev. Med., 1998, 49, 31–62 CrossRef CAS.
- S. Seshadri, A. Beiser, J. Selhub, P. F. Jacques, I. H. Rosenberg, R. B. D'Agostino, P. W. Wilson and P. A. Wolf, N. Engl. J. Med., 2002, 346, 476–483 CrossRef CAS.
- P. Lochman, T. Adam, D. Friedecký, E. Hlídková and Z. Skopková, Electrophoresis, 2003, 24, 1200–1207 CrossRef CAS.
-
(a) H. Refsum, A. D. Smith, P. M. Ueland, E. Nexo, R. Clarke, J. McPartlin, C. Johnston, F. Engbaek, J. Schneede, C. McPartlin and J. M. Scott, Clin. Chem., 2004, 50, 3–32 CrossRef CAS;
(b) O. Nekrassova, N. S. Lawrence and R. G. Compton, Talanta, 2003, 60, 1085–1095 CrossRef CAS.
- R. P. Steegers-Theunissen, G. H. Boers, F. J. Trijbels and T. K. Eskes, N. Engl. J. Med., 1991, 324, 199–200 CAS.
- J. B. van Meurs, R. A. Dhonukshe-Rutten, S. M. Pluijm, M. van der Klift, R. de Jonge, J. Lindemans, L. C. de Groot, A. Hofman, J. C. Witteman, J. P. van Leeuwen, M. M. Breteler, P. Lips, H. A. Pols and A. G. Uitterlinden, N. Engl. J. Med., 2004, 350, 2033–2041 CrossRef CAS.
- L. L. Wu and J. T. Wu, Clin. Chim. Acta, 2002, 322, 21–28 CrossRef CAS.
- M. B. Satterfield, L. T. Sniegoski, M. J. Welch, B. C. Nelson and C. M. Pfeiffer, Anal. Chem., 2003, 75, 4631–4638 CrossRef CAS.
-
(a) B. C. Nelson, M. B. Satterfield, L. T. Sniegoski and M. J. Welch, Anal. Chem., 2005, 77, 3586–3593 CrossRef CAS;
(b) B. C. Nelson, C. M. Pfeiffer, L. T. Sniegoski and M. B. Satterfield, Anal. Chem., 2003, 75, 775–784 CrossRef CAS.
- C. Bayle, E. Caussé and F. Couderc, Electrophoresis, 2004, 25, 1457–1472 CrossRef CAS.
-
(a) D. Zhang, M. Zhang, Z. Liu, M. Yu, F. Li, T. Yi and C. Huang, Tetrahedron Lett., 2006, 47, 7093–7096 CrossRef CAS;
(b) O. Rusin, N. N. St Luce, R. A. Agbaria, J. O. Escobedo, S. Jiang, I. M. Warner, F. B. Dawan, K. Lian and R. M. Strongin, J. Am. Chem. Soc., 2004, 126, 438–439 CrossRef CAS;
(c) W. Wang, O. Rusin, X. Xu, K. K. Kim, J. O. Escobedo, S. O. Fakayode, K. A. Fletcher, M. Lowry, C. M. Schowalter, C. M. Lawrence, F. R. Fronczek, I. M. Warner and R. M. Strongin, J. Am. Chem. Soc., 2005, 127, 15949–15958 CrossRef CAS;
(d) M. Kawatsura, F. Ata, T. Hirakawa, S. Hayase and T. Itoh, Tetrahedron Lett., 2008, 49, 4873–4875 CrossRef CAS.
- H. Chen, Q. Zhao, Y. Wu, F. Li, H. Yang, T. Yi and C. Huang, Inorg. Chem., 2007, 46, 11075–11081 CrossRef CAS.
- W. Wang, J. O. Escobedo, C. M. Lawrence and R. M. Strongin, J. Am. Chem. Soc., 2004, 126, 3400–3401 CrossRef CAS.
- M. Zhang, M. Yu, F. Li, M. Zhu, M. Li, Y. Gao, L. Li, Z. Liu, J. Zhang, D. Zhang, T. Yi and C. Huang, J. Am. Chem. Soc., 2007, 129, 10322–10323 CrossRef CAS.
-
(a) F. X. Zhang, L. Han, L. B. Israel, J. G. Daras, M. M. Maye, N. K. Ly and C. J. Zhong, Analyst, 2002, 127, 462–465 RSC;
(b) S.-J. Chen and H.-T. Chang, Anal. Chem., 2004, 76, 3727–3734 CrossRef CAS;
(c) R. Hong, J. M. Fernández, H. Nakade, R. Arvizo, T. Emrick and V. M. Rotello, Chem. Commun., 2006, 2347–2349 RSC;
(d) I. I. Lim, W. Ip, E. Crew, P. N. Njoki, D. Mott, C. J. Zhong, Y. Pan and S. Zhou, Langmuir, 2007, 23, 826–833 CrossRef CAS;
(e) I. I. Lim, D. Mott, M. H. Engelhard, Y. Pan, S. Kamodia, J. Luo, P. N. Njoki, S. Zhou, L. Wang and C. J. Zhong, Anal. Chem., 2009, 81, 689–698 CrossRef CAS.
- G. A. Rance, D. H. Marsh and A. N. Khlobystov, Chem. Phys. Lett., 2008, 460, 230–236 CrossRef.
- K. G. Thomas and P. V. Kamat, Acc. Chem. Res., 2003, 36, 888–898 CrossRef CAS.
-
(a) C. Lu, Y. Zu and V. W. Yam, Anal. Chem., 2007, 79, 666–672 CrossRef CAS;
(b) C.-C. Huang and W.-L. Tseng, Anal. Chem., 2008, 80, 6345–6350 CrossRef CAS.
-
(a) C. Lu and Y. Zu, Chem. Commun., 2007, 3871–3873 RSC;
(b) H.-P. Wu, C.-C. Huang, T.-L. Cheng and W.-L. Tseng, Talanta, 2008, 76, 347–352 CrossRef CAS.
-
(a) C. S. Thaxton, H. D. Hill, D. G. Georganopoulou, S. I. Stoeva and C. A. Mirkin, Anal. Chem., 2005, 77, 8174–8178 CrossRef CAS;
(b) S. J. Hurst, A. K. Lytton-Jean and C. A. Mirkin, Anal. Chem., 2006, 78, 8313–8318 CrossRef CAS;
(c) M.-D. Li, T.-L. Cheng and W.-L. Tseng, Electrophoresis, 2009, 30, 388–395 CrossRef CAS.
- P. Zuman, Chem. Rev., 2004, 104, 3217–3238 CrossRef CAS.
-
(a) J. R. Benson and P. E. Hare, Proc. Natl. Acad. Sci. U.S.A., 1975, 72, 619–622 CAS;
(b) Y. V. Tcherkas and A. D. Denisenko, J. Chromatogr. A, 2001, 913, 309–313 CrossRef CAS.
- P. C. Lee and D. Meisel, J. Phys. Chem., 1982, 86, 3391–3395 CrossRef CAS.
- C.-C. Huang, C.-T. Chen, Y.-C. Shiang, Z.-H. Lin and H.-T. Chang, Anal. Chem., 2009, 81, 875–882 CrossRef CAS.
-
(a) Y. Mukai, T. Togawa, T. Suzuki, K. Ohata and S. Tanabe, J. Chromatogr. B, 2002, 767, 263–268 CrossRef CAS;
(b) G. Chwatko and H. Jakubowski, Clin. Chem., 2005, 51, 408–415 CrossRef CAS.
-
(a) B. Seiwert and U. Karst, Anal. Chem., 2007, 79, 7131–7138 CrossRef CAS;
(b) T. Fiskerstrand, H. Refsum, G. Kvalheim and P. M. Ueland, Clin. Chem., 1993, 39, 263–271 CAS.
|
This journal is © The Royal Society of Chemistry 2010 |