DOI:
10.1039/B915975D
(Paper)
Analyst, 2010,
135, 149-156
Functionalized monolayers on mesoporous silica and on titania nanoparticles for mercuric sensing†
Received
4th August 2009
, Accepted 4th November 2009
First published on
13th November 2009
Abstract
Heterogeneous “naked-eye” colorimetric and spectrophotometric cation sensors were prepared by immobilization of an azobenzene-coupled receptor onto mesoporous silica (AR-SiO2) or titania nanoparticles (AR-TiO2) via sol–gel or hydrolysis reactions. The optical sensing ability of AR-SiO2 was studied by addition of metal ions such as K+, Ca2+, Sr2+, Co2+, Cd2+, Pb2+, Zn2+, Fe3+, Cu2+ and Hg2+ ions (all as chlorides) in aqueous solution. Upon the addition of Hg2+ ion in suspension, the AR-SiO2 resulted in a color change from yellow to deep red. No significant color changes were observed in the parallel experiments with K+, Ca2+, Sr2+, Co2+, Cd2+, Pb2+, Zn2+, Fe3+ or Cu2+ ion. These findings confirm that the AR-SiO2 can be useful as chemosensors for selective detection of Hg2+ ion over a range of metal ions in aqueous solution. Also, the color change of AR-SiO2 was independent of the presence of anions NO3−, ClO4−, Br− and I−. We also prepared a portable chemosensor kit by coating a 4 μm thick film of AR-TiO2 onto a glass substrate. We found that this AR-TiO2 film detects Hg2+ ion at pH 7.4 with a sensitivity of 28 nM. Finally, we tested the effect of pH on AR-TiO2 with Hg2+ ion between pH 1.0 to 11.0. The absorbance and color changes of AR-TiO2 were almost constant between pH 4 and 11. The results imply that the AR-TiO2 film is applicable as a portable colorimetric sensor for the detection of Hg2+ ion in the environmental field.
Introduction
Chemical sensors are molecular receptors that transform into analytically useful signals upon binding to specific guests. Such sensors have attracted interest in fields such as waste management, environmental chemistry, clinical toxicology, and bioremediation of radionuclides due to their potential for easy detection, and quantification of pollutant species.1–9 Among these, the selective detection of metal ions such as mercury and lead is critical for environmental monitoring, as these are highly toxic and common pollutants.
Various approaches for mercury screening, including atomic absorption/emission spectroscopy and inductively coupled plasma mass spectrometry, have been investigated.10–12 Although these instrumental analyses are currently used in applications relevant to the detection of metal toxins, there is still a need to develop inexpensive and simple methods for the detection of metal toxins. Among the various sensor approaches, optical sensors that allow on-site, real-time, qualitative, or semiquantitative detection without the use of bulky or complex spectroscopic instrumentation have received a great deal of attention as promising methods for the determination of pollutant species in environmental analysis.13–16 However, most of the sensors developed so far are kinetically slow with a limited sensitivity for detection below the permissible level of metal toxins, indicating a lack of control of the remote sensing of pollutant species.
Very recently, organic-inorganic hybrid materials have been investigated as new materials for ion recognition and sensing. Receptors immobilized on inorganic nanomaterials such as SiO2, Al2O3 and TiO2 constitute solid chemosensors and, as such, have important advantages when used in the heterogeneous solid-liquid phase.17–28 Firstly, receptors immobilized on an inorganic support can remove the guest molecules (toxic metal ions or anions) from the pollutant solution. Secondly, the inorganic oxides can be fabricated as functionalized porous nanomaterials. In particular, nanocrystalline TiO2 films are potentially useful materials for optical sensors due to their high surface area and excellent optical transparency in the visible region of the spectrum (λ > 400 nm).
Mesoporous silica and titania nanoparticles (SBA-15 and MCM-41) are promising as inorganic support materials due to their homogeneous porosity and large surface area. Only limited examples of such heterogeneous sensors have been reported,17–28 however. Such sensors take advantage of the independent solubility of the receptor in water and in organic solvents. Very recently, for example, Martínez-Máñez et al. reported on a fabrication of the squaraine-based receptor immobilized on a mesoporous 3D hybrid material for Hg2+ ion detection and adsorption.29 Addition of the colorless hybrid material to a solution containing Hg2+ ion resulted in a rapid and dramatic change to deep blue. The solution also showed a remarkable fluorescence. However, the hybrid material could not be renewed after adsorption of Hg2+ ion because it acts as the chemodosimeter for these ions. In addition, this hybrid material was selectively detectable for Hg2+ in a mixed solvent of acetonitrile and water due to the solubility of the squaraine receptor at high Hg2+ concentration.
Furthermore, Willner et al. reported that oligonucleotides immobilized on gold nanoparticles enabled the colorimetric detection of Hg2+.30 The gold nanoparticles were highly aggregated in the presence of Hg2+ ion, which changed from red to blue. Also, the gold nanoparticles were not renewable after adsorption of Hg2+ ion.
Based on this idea, in this work we explore a new approach to the development of nanomaterial chemosensors. We synthesized the azobenzene-coupled acyclic receptor 6 containing a triethoxylsilane moiety (Scheme 1) and immobilized 6 onto the surface of mesoporous silica by sol–gel reaction. We also attached 4 containing a carboxylic acid to titania nanoparticles of 50 nm diameter in order to prepare a portable chromogenic sensor. The receptors 4 and 6 were immobilized by different methods on the two different supports. To the surface of mesoporous silica, the receptors were attached by covalent bonds via a sol–gel grafting method. To the TiO2 nanoparticles, the receptors were attached via hydrogen bonds. After immobilizing the receptors, we transformed the ester of receptor 4 or 6 into an acid-type receptor attached onto mesoporous silica and titania by hydrolysis. We herein describe the fabrication of an acyclic acid-type receptor immobilized on mesoporous silica (AR-SiO2) and the same receptor on titanium oxide nanoparticles (AR-TiO2), both of which selectively change color upon the addition of Hg2+.
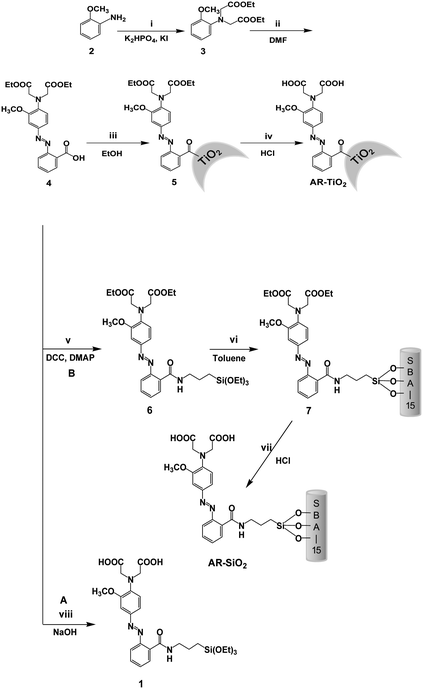 |
| Scheme 1 Synthetic method. Reaction conditions: (i) BrCH2CO2Et, 60 °C; (ii) diazonium salt, DMF, 0 °C; (iii) TiO2 nanoparticles, reflux; DMF = N,N′-dimethylformamide; (iv) HCl, methanol; (v) 3-Aminopropyltriethoxysilane, DCC, DMAP, EA, RT; (vi) mesoporous silica, toluene, reflux; (vii) HCl, methanol; (viii) NaOH. | |
Experimental
Characterization
1H and 13C NMR spectra were recorded on a Bruker 300 apparatus. Infrared spectra of silica powder pellets were obtained using a Shimadzu FT-IR 8400S and the mass spectrum was obtained with a JEOL JMS-700 mass spectrometer. Powder X-ray diffraction patterns were recorded on a Siemens D8 Advance diffractometer using Cu-Kα radiation and a secondary monochromator. Transmission electron microscopy (TEM) images were captured with a JEOL JEM – 2100 F microscope. Nitrogen-adsorption isotherms were measured at 78 K on a Micromeritics ASAP 2010 analyzer. Thermal gravimetry analysis was conducted on a TA SDT Q600 with a heating rate of 10 °C min−1 using a Pt pan in air. The temperature was scanned from 25 °C to 900 °C.
Preparation of mesoporous silica
Mesoporous silica was synthesized starting from the preparation of a hydrochloric acid solution of P-123 [(poly(ethylene oxide)-poly(propylene oxide)-poly(ethylene oxide) triblock copolymer)]. Tetraethyl orthosilicate (TEOS) was then added and the mixture was stirred at 40 °C for 20 h. The molar composition was 1
:
5.9
:
193
:
0.017 TEOS
:
HCl
:
H2O
:
P-123. The solid was aged at 65 °C for 1 day and then was filtered, washed and dried at 90 °C. To cleave the template to generate mesopores, 1.0 g of as-synthesized SBA-15 was mixed with 100 mL of 60 wt % H2SO4 solution and refluxed at 95 °C for 1 day. The product was recovered by washing with water and dried at 90 °C. To generate mesopores, the acid-treated sample was heated to 200 °C in air. To remove cationic surfactants from the resulting dried fiber-like flocculates and particles, the sample was calcined in a box furnace in air at 500 °C for 5 h, with a ramp rate of 1 °C min−1.
Immobilization of receptor 6 onto mesoporous silica
Compound 6 (100 mg) was dissolved in toluene (10 mL). The mesoporous silica (100 mg) was added as a solid. The suspension of silica was stirred in under reflux conditions for 24 h in toluene. Then, the collected solid was washed copiously with toluene (50 mL) to rinse away any surplus 5 and dried under vacuum.
Hydrolysis of mesoporous silica immobilized with 6
To obtain the acid from the receptor (7) attached to mesoporous silica, the mesoporous silica immobilized with receptor 6 was first dispersed in methanol (2 mL). The suspension of silica was maintained for 10 h at room temperature. After filtration, the solid product was washed with Na2CO3 solution and water and dried.
The TiO2 nanoparticles were obtained by a method reported previously.25
Preparation of receptor 4 immobilized TiO2 films
TiO2 paste was prepared from a sol–gel colloidal suspension containing 12.5 wt% TiO2 and 6.2 wt% Carbowax 20000. The nanocrystalline TiO2 particles were synthesized by the following procedure: 20 mL of titanium iso-propoxide (Aldrich Co.) were injected into 5.5 g of glacial acetic acid under argon atmosphere and stirred for 10 min. The mixture was transferred into a flask containing 120 mL of 0.1 M nitric acid at room temperature and stirred vigorously. The flask was left uncovered and heated at 80 °C for 8 h. After cooling, the solution was filtered using a 0.45 mm syringe filter, diluted to 5 wt% TiO2 by adding H2O and then autoclaved at 220 °C for 12 h. The colloids were re-dispersed with a 60 s cycle burst from an LDU Soniprobe. Finally, the solution was concentrated to 12.5 wt% TiO2 on a rotary evaporator using a membrane vacuum pump at a temperature of 40 °C. Carbowax 20.000 was added and the resulting paste was stirred slowly overnight to ensure homogeneity. For the film TiO2 preparation, 3 mL of the solution detailed above were spread on glass slides with a glass rod and using adhesive tape as spacers. After the films were dried in air, they were sintered at 450 °C for 20 min. All samples used in this work were films of 4 μm thickness. Functionalization of the TiO2 films was achieved by immersing the films in a 1 mM solution of receptor 4 in 1
:
1 acetonitrile/tert-butyl alcohol mixture overnight, followed by rinsing in ethanol to remove unadsorbed receptor 4.
Coloration and extraction of metal ions by AR-SiO2 particles and AR-TiO2 films
The AR-SiO2 (10 mg) was added to metal ion solutions (5.0 equiv with respect to 1 anchored to the AR-SiO2). The mixture was stirred for 10 min. After filtration, the concentration of metal ions was analyzed by ICP (DX-500, DIONEX). The UV-vis spectra of metal-loaded AR-SiO2 were measured by a Shimadzu UV-2401 PC UV-Vis spectrophotometer. In addition, the reversibility of metal adsorption was measured. EDTA (10.0 μM, 2 mL) was added to the AR-SiO2 suspension (at pH 7.4) to remove Hg2+ ion bound to AR-SiO2. Then, the UV-vis absorption change of the H2O suspension of AR-SiO2 was measured. In addition, the AR-TiO2 films were dipped in cation aqueous solutions of interest in buffered condition, and then dried in air, with changes in coloration noted by visual inspection as well as by measurement of UV-vis absorption.
Compound 3
To a 50 mL round bottom flask, o-anisidine (2) was added to ethylbromoacetate in potassium dihydrogen phosphate in CH3CN (10 mL). The reaction mixture was maintained for 10 h at 60 °C. After cooling to room temperature, the reaction mixture was reduced, then was dissolved into CHCl3 (50 mL) and washed with water (2 × 30 mL). The organic phase was dried over MgSO4 and reduced to oil. The crude product was purified on a silica column using 70
:
30 Hex
:
EtOAc to produce 3 as a white solid. Properties of 3 are as follows: m.p. 78–80 °C; 1H NMR (300 MHz, CDCl3, TMS) δ = 6.87 (d, 2J(H,H) = 12.3 Hz, 1H; Ar–H), 4.20 (d, 2J (H,H) = 7.2 Hz, 1H; Ar–H), 3.80 (s, 3H; CH3) 1.26 (t, 3J(H,H) = 7.2 Hz, 6H; CH3); 13C NMR (300 MHz, CDCl3, TMS) δ = 171, 151, 122, 120, 119, 111, 77, 77, 77, 76, 60, 55, 53, 30, 14; IR (KBr): ν = 3393, 3090, 2931, 2853, 1740, 1653, 1373, 1032, 751 cm−1; High resolution mass spectrum (HRMS) (FAB+) m/z 295.14 [(M + H)+ calcd for C15H21NO5:295.16]. Anal. Cald for C15H22NO5: C, 61.00; H, 7.17; N, 4.74. found: C, 60.68; H, 7.23; N, 4.69.
Compound 4
To a 50 mL round bottom flask, o-amino benzoic acid (1.047 g, 7.5 mmol) was added which contained a solution of 1
:
1, THF
:
H2O (20 mL) and NaNO2 (0.5261 g, 7.6 mmol). While this mixture was being stirred at 0 °C, HCl (1.0 mL, 12 M) was slowly added. The resulting solution was added dropwise to a 100 mL single neck round bottom flask containing the appropriate ester (3) (6.8 mmol) in a solution of 1
:
1 THF
:
H2O (40 mL). This solution was held at 0 °C for approximately 2 h and then was left stirring overnight at room temperature. The resulting dark red solution was reduced down, dissolved into CHCl3 (60 mL) and washed with water (2 × 30 mL). The organic phase was dried over MgSO4 and reduced to oil. The crude product was purified on a silica column using 70
:
30 Hex
:
EtOAc, to produce 4 as a bright red solid. Properties of 4 are as follows: m.p. 101–103 °C; 1H NMR (300 MHz, CDCl3, TMS) δ = 8.10 (d, 2J(H,H) = 7.75 Hz, 1H; Ar–H), 7.59 (d, 2J(H,H) = 7.2 Hz, 1H; Ar–H), 7.48 (t, 3J(H,H) = 7.8 Hz, 1H; Ar–H), 6.9 (d, 2J(H,H) = 4.5 Hz, 1H; Ar–H), 4.18 (m, 8H), 3.8 (s, 3H; CH3), 1.26 (t, 3J(H,H) = 7.2 Hz, 6H; CH3); 13C NMR (300 MHz, CDCl3, TMS) δ = 187, 182, 154, 144, 135, 134, 134, 133, 133, 127, 127, 125, 120, 116, 58, 43, 42, 23, 18,; IR (KBr): ν = 3414, 3270, 2940, 2856, 1870, 1735, 1637, 968, 804 cm−1; HRMS (FAB+) m/z 443.17 [(M + H)+ calcd for C22H25N3O7: 443.20]. Anal. Cald for C22H25N3O7: C, 59.59; H, 5.68; N, 9.48. found: C, 60.11; H, 5.80; N, 9.35.
Compound 6
To a 20 mL round bottom flask, 4 (250 mg, 0.42 mmol) was added to aminopropyltriethoxysilane (1.0 g, 5.58 mmol) in ethyl acetate (10 mL). The mixture was stirred under nitrogen for two minutes and then cooled to 0 °C. 1-Hydroxybenzotriazole (HOBt, 68 mg, 0.5 mmol) and dicyclohexyl carbodiimide (DCC, 1.870 g, 9.07 mmol) were added simultaneously as a mixture of solids. The reaction mixture was allowed to warm to room temperature and stirred for 24 h. The precipitate was removed by filtration. The filtrate was then washed with aq. NaHCO3 (satd.) and aq. NaHSO4 (satd.). The solution was dried with MgSO4 and the solvent was removed by rotary evaporation to produce a reddish crude. This crude product was purified by column chromatography (silica-gel, n-hexane: ethyl acetate, 1
:
1, Rf 0.45) to give the product (1.24 g, 65%). Properties of 6 are as follows: m.p. 115–117 °C; 1H NMR (300 MHz, DMSO) δ = 7.5, 7.5, 7.4 (m, 3H) 6.9, 6.8, 6.8, 6.7 (m, 4H), 4.17 (t, 3J(H,H) = 6.9 Hz, 1H; Ar–H), 3.79 (s, 3H), 1.73 (t, 3J(H,H) = 2.4 Hz, 1H; Ar–H) 1.24 (m, 15H); IR (KBr): ν = 3414, 3270, 2940, 2856, 1870, 1735, 1637, 1121, 968, 804 cm−1; HRMS (FAB+) m/z 646.30 [(M + H)+ calcd for C31H46N4O9Si: 646.34]. Anal. Cald for C31H46N4O9Si: C, 57.56; H, 7.17; N, 8.66. found: C, 57.71; H, 7.02; N, 8.33.
Compounds 5 and 7
The synthetic methods will be described in detail later.
Results and discussion
The synthesis of the target compound 1 is difficult due to the polymerization of the triethoxyl group during hydrolysis, which removes the ethyl groups of 4 by pathway A in Scheme 1. Thus, we tried to obtain 1 by hydrolysis of 4 in acidic conditions after immobilization of receptor 6 onto the surface of mesoporous silica by pathway B. To prepare a selective chromogenic receptor for Hg2+, we began with 2, then formed o-anisidine (3) by alkylation using two equivalents of ethylbromoacetate in the presence of potassium dihydrogen phosphate and potassium iodide in CH3CN. The azobenzene ester 4 was made by the reaction of the diazonium salt of o-aminobenzoic acid with 3. Subsequently, treatment with 4 and aminopropyltriethoxysilane as precursor for the sol–gel reaction afforded compound 6 as a yellow powder. Then, immobilization of the chromogenic receptor 6 to mesoporous silica was conducted under reflux for 24 h in toluene. In this process, the triethoxylsilyl group of 6 attached onto mesoporous silica undergoes hydrolysis and attaches covalently to the surface of the mesoporous silica.15 After cooling to room temperature, the yellow solid product was filtered, washed with THF, and dried. Finally, a chemosensing material was obtained as a light yellow solid by hydrolysis of 7 attached onto mesoporous silica under acidic conditions. The acyclic receptor 1 immobilized mesoporous silica (AR-SiO2) was characterized by transmission electron microscopy (TEM), UV-vis spectroscopy, thermogravimetric analysis (TGA) and FT-IR.
Preparation of AR-SiO2
In Fig. 1 and Fig. S1 (ESI),† TEM images of AR-SiO2 and the mesoporous silica clearly show the formation of a well-ordered hexagonal arrangement of mesoporous channels before and after attaching the receptor 1. To investigate the porosity changes of the mesoporous silica induced by the introduction of the azobenzene-coupled derivative 1, we measured the surface area, pore volumes and pore diameters of both mesoporous silica and AR-SiO2 using nitrogen adsorption-desorption isotherms (Fig. 1B). The mesoporous silica has a BET (Brunauer-Emmett-Teller) surface area of 1119.72 m2 g−1 and a pore volume of 0.49 cm3 g−1. On the other hand, we observed that the AR-SiO2 has a BET surface area of 673.21 m2 g−1 and a pore volume of 0.26 cm3 g−1. The mesoporous silica and the AR-SiO2 have BJH (Barrett-Joyner-Halenda) pore diameters of 2.24 and 2.15 nm, respectively (Fig. S2, ESI).† The decreased surface area and pore diameter in AR-SiO2 are attributable to the attachment of the acyclic azo-coupled derivative to the mesoporous silica. Furthermore, from the results of the TGA measurement (Fig. S3, ESI),† we determined that the AR-SiO2 consists of only 18.0 wt% of 1.
For further structural proof of the AR-SiO2, we carried out IR spectroscopy of both mesoporous silica and AR-SiO2. For the mesoporous silica, IR peaks appear at 3450, 1658 and 1084 cm−1. For AR-SiO2 (Fig. S4, ESI),† peaks appear at 3382, 2976, 2933, 2884, 1626, 1615, 1570, 1471, 1446, 1428 and 1382 cm−1. These new peaks originate from the acyclic azo-coupled receptor 1, providing solid evidence that 1 is indeed attached to the surface of the mesoporous silica.
Preparation of the azobenzene-based receptor 4 immobilized TiO2 film (AR-TiO2)
In consideration of extending its usefulness, immobilization of the chromogenic receptor 4 into the TiO2 nanoparticles was conducted under reflux conditions for 24 h in toluene. In this process, the receptor 4 undergoes hydrolysis and attaches covalently to the surface of TiO2.9,13 After cooling to room temperature, the red solid product was filtered, washed with ethanol, and then dried. For the structural proof of the TiO2, we took IR spectroscopy of both TiO2 and AR-TiO2. For TiO2, IR peaks appear at 3391, 1639 and 655 cm−1 whereas for AR-TiO2 appeared at 3421, 2977, 2926, 2874, 1728, 1633, 1594, 1513, 1439, 1371, 1284, 1131, 1069 and 655 cm−1 (Fig. S5, ESI)† where new peaks are originated from azobenzene-coupled receptor 4, giving us a solid evidence that 4 is certainly attached onto the surface of the TiO2 nanoparticles. In addition, TEM images of TiO2 nanoparticles and AR-TiO2 were observed (Fig. 2). Both TiO2 nanoparticles before and after immobilization of the receptor 4 showed 30∼60 nm of diameters without any morphological change.
To prepare AR-TiO2 films onto glass slides, 3 mL of the solution detailed above were spread on the glass slides with a glass rod and using adhesive tape as spacers. After the films were dried in air, they were sintered at 450 °C for 20 min. Sensitization of the TiO2 films was achieved by immersing the films in a 1.0 mM solution of receptor 4 in 1
:
1 acetonitrile/tert-butyl alcohol overnight, followed by rinsing in ethanol to remove unadsorbed receptor 4. Finally, the slight yellow color of the AR-TiO2 film was obtained.
Coloration of AR-SiO2 with metal ions
We investigated the spectroscopic properties of the AR-SiO2 towards the metal ions K+, Ca2+, Sr2+, Co2+, Cd2+, Pb2+, Zn2+, Fe3+, Cu2+ and Hg2+ in aqueous solution (pH = 7.4, 5.0 equiv with respect to 1 anchored to the mesoporous silica). Interestingly, upon the addition of Hg2+ in H2O suspension, AR-SiO2 resulted in a color change from light yellow to red within 10 s (Fig. 3A(b) and 3B(b)). In this category of chromophore, photoexitation causes a net electronic charge transfer from the donor end (bridgehead nitrogen) to the acceptor end within the chromophore. Thus, the observed color change is ascribed to the formation of a complex with strong coordination bonding between the bridgehead nitrogen atom of aniline group of 1 and Hg2+.7,23 Except for Hg2+, no significant color changes were observed in the experiments using other metal ions (Fig. 3A(c–i) and Fig. S6, ESI).† These findings confirm that AR-SiO2 can be useful as a colorimetric sensing material for the selective detection of Hg2+ in the presence of other metal ions. This is a rare example of chromogenic sensing for a specific metal ion by functional inorganic nanomaterials. The association constant (K) for the Hg2+ coordination to AR-SiO2 was calculated to be 3.05 × 105 M−1 (log K = 5.48).28
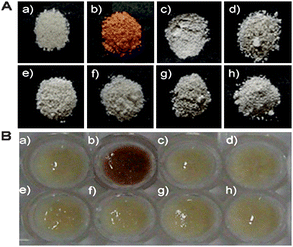 |
| Fig. 3 The colorimetric response of (A) dried and (B) H2O suspension samples of AR-SiO2 (5.0 mg) in the (a) absence and the presence of (b) HgCl2 (5.0 equiv), (c) CoCl2 (5.0 equiv), (d) CdCl2 (5.0 equiv), (e) PbCl2 (5.0 equiv), (f) ZnCl2 (5.0 equiv), (g) FeCl3 (5.0 equiv) and (h) CuCl2 (5.0 equiv) at pH = 7.4. | |
The UV-vis spectrum of Hg2+-loaded AR-SiO2 is unchanged in the presence of an excess amount of K+, Ca2+, Sr2+, Co2+, Cd2+, Pb2+, Fe3+, Cu2+ and Zn2+ (Fig. S7, ESI),† indicating that AR-SiO2 shows great promise as a selective chemosensor for the detection of Hg2+.
To develop the AR-SiO2 as a general mercury cation sensor which is independent of the anion present, we investigated the anion effect by addition of other anion such as NO3−, ClO4− and Br− (Fig. S8, ESI).† As observed for the HgCl2 solution, the color of the AR-SiO2 also changed from yellow to red. This finding indicates that the AR-SiO2 can be employed for the detection of Hg2+ independently of the other anion(s) present.
The Hg2+-loaded AR-SiO2 was isolated to confirm the binding efficiency of Hg2+ by AR-SiO2. Then, the solid UV-vis spectrum for the Hg2+-loaded AR-SiO2 was compared with that for AR-SiO2 alone (Fig. 4A). The Hg2+-loaded AR-SiO2 spectrum exhibits an absorption maximum at 495 nm, whereas the absorption peak appears at 310 nm for the Hg2+-free one, indicating that the Hg2+ is efficiently bound to 1 attached in the AR-SiO2 by covalent bonds.
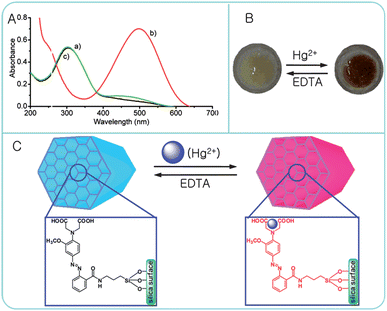 |
| Fig. 4 (A) Solid UV-vis spectra of AR-SiO2 (5.0 mg) in the (a) absence and (b) the presence of HgCl2 (5.0 equiv) and (c) after addition of EDTA (10.0 μM, 2 mL). (B) Picture and (C) proposed structure of AR-SiO2·Hg2+ before and after treatment of EDTA (10.0 μM). | |
In addition, we confirmed the reversibility of the color change of AR-SiO2 by removing the Hg2+ ion bound to AR-SiO2 by treatment with EDTA. As expected, the red color of AR-SiO2 in the presence of Hg2+ ion was changed into light yellow upon the EDTA (10.0 μM) treatment (Fig. 4B). Once again, the color change was fully reversible with the addition of EDTA (10.0 μM). Because the Hg2+ ion bound to AR-SiO2 is dissociated by EDTA, the AR-SiO2 can be repeatedly used by renewing with EDTA (Fig. 4C). Clearly, AR-SiO2 exhibits excellent reusability, as almost no loss in AR-SiO2 sensitivity was observed after eight repeated dipping/rinsing cycles.
In order to understand the coordination behavior between the receptor 1 attached to AR-SiO2 and the Hg2+ ion, we made repeated attempts to obtain the crystal structure of the complex 1 with the Hg2+ ion, but were not successful. As an alternative, we measured the UV-vis spectra of AR-SiO2 with the addition of Hg2+ ion to confirm the stoichiometry between 1 attached onto AR-SiO2 and the Hg2+ ion. A spectral variation of AR-SiO2 in H2O was observed upon the gradual addition of HgCl2. As a function of the Hg2+ concentration, a new absorption band centered at 495 nm leading to an obvious color change from light yellow to red was observed. The red-shift from 310 to 495 nm of the absorption of AR-SiO2 is attributed to a strong binding affinity between the nitrogen atom of 1 attached to AR-SiO2 and the Hg2+ ion (Fig. S9, ESI†).7 The stoichiometry for the 1 complex with Hg2+ was examined by a Job plot. As shown in Fig. S8,† it was found that the 1-Hg2+ complex concentration approaches a maximum when the molar faction of [1]/[1] + [Hg2+] is about 0.5, indicating that it forms a 1
:
1 complex of 1 attached to AR-SiO2 with Hg2+ ion, as show in Fig. S10, ESI.†
Fig. S11 (ESI)† shows the standard calibration data (Abs. vs. [Hg2+]) for AR-SiO2. A linear response is observed (between 1.0 μM and 10 μM) with a sensitivity of ∼1.0 μM. This sensitivity is equivalent to those previously reported for spectrophotometric sensors anchored to mesoporous aluminosilicates.24
The above results encouraged us to test the separation of Hg2+ from the waste solution. The test sample was prepared by adding AR-SiO2 (10 mg) to 1 mL of waste containing 2.0 μM Hg2+. The Hg2+-loaded AR-SiO2 was then removed by filtration from the waste. To determine the amount of Hg2+ separated by the AR-SiO2, the amount of Hg2+ left in the waste solution was determined by inductively coupled plasma mass spectrometry (ICP-MS). The ICP-MS measurements indicated that only 7% of the original Hg2+ remained in the waste solution, suggesting that the AR-SiO2 removed 93% of the Hg2+. We also analyzed the Hg2+-loaded AR-SiO2 sample by UV-vis spectroscopy (Fig. S12, ESI).† The absorbance of the Hg2+-loaded AR-SiO2 was 0.26, coinciding with the calibration curve (Fig. S11, ESI).†
Furthermore, we examined the pH effect on the Hg(II) uptake by measuring the absorbance of AR-SiO2 treated with Hg(II) solutions (pH 1.0–12.0). As shown in Fig. S13 (ESI),† no significant absorbance or color changes were observed between pH 2–9, suggesting the proposed AR-SiO2 sensor can be used in pH 2–9.
The extraction ability of the AR-SiO2 was also estimated by measuring the amount of Hg2+ ion adsorbed on the AR-SiO2 by ICP, resulting in 90% of Hg2+ ion being extracted by AR-SiO2. This result suggests that the AR-SiO2 is potentially useful as a stationary phase for the separation of Hg2+ ion in liquid chromatography. Also, the adsorption capacity of the AR-SiO2 was measured through the solid extraction using solutions of binary metal ions (Hg2+/Fe3+, Hg2+/Co2+, Hg2+/Cd2+, Hg2+/Pb2+ and Hg2+/Zn2+) resulting in 85.7–90.5% of Hg2+ ion being adsorbed by AR-SiO2 (Fig. 5). In contrast, other metal ions such as Zn2+, Cd2+, Pb2+ and Zn2+ were extracted into the solid phase at percentages of only 2.5–5.2% in the competition system. These results suggest that AR-SiO2 is a useful adsorbent for the selective separation of Hg2+ over a range of transition and heavy metal ions.
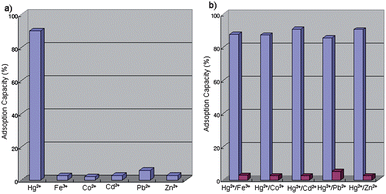 |
| Fig. 5 Adsorption capacities of AR-SiO2 for (a) single and (b) binary metal ions in H2O. | |
Coloration of TiO2 films with metal ions
We now address the metal cation sensing ability of AR-TiO2 films. For the cation sensing experiments, the AR-TiO2 films were dipped into cation solutions of interest, then dried in air, with any color change determined by “naked-eye” inspection and by measurement of solid UV-vis absorption spectra. In all cases, changes in film color were found to be complete within 1–2 s of immersion, with the resulting coloration being stable for in excess of one day, both for films immersed continuously in the cation solution and for films dried in air. Typical data are shown in Fig. 6, showing a photographic image of the films after immersion in 1.0 mM solutions of chloride salts of the seven cations (Co2+, Cd2+, Pb2+, Zn2+, Fe3+, Cu2+ and Hg2+). The yellow color of the AR-TiO2 film changed to a deep red color when the film was dipped in Hg2+ solution. On the other hand, no significant color changes were observed with the other metal ions. These results indicate that the AR-TiO2 film selectively detected only the Hg2+ ion. Since the preparation of the AR-TiO2 films prepared in this work is much simpler than the sol–gel grafting method, we propose that this AR-TiO2 film is highly useful as a chemosensor to detect Hg2+ ions.
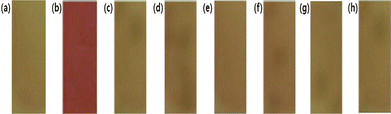 |
| Fig. 6 Color changes observed after the AR-TiO2 films were dipped in 1.0 mM aqueous solutions of the analytes: (a) the absence and the presence of (b) HgCl2, (c) CoCl2, (d) CdCl2, (e) PbCl2, (f) ZnCl2, (g) FeCl3 and (h) CuCl2. | |
We also observed the solid UV-vis spectra of the AR-TiO2 films after dipping into the various cation solutions. Fig. 7 shows the corresponding absorption spectra for selected films. It is apparent that the film coloration is only sensitive to the Hg2+ ion. Hg2+ exposure resulted in a deep red coloration of the film caused by a shift of the absorption maximum from 310 to 492 nm. This bathochromic shift is similar to that observed for AR-SiO2. On the other hand, no significant changes in the UV-vis spectra were observed for any other cations tested (including Co2+, Cd2+, Pb2+, Zn2+, Fe3+ and Cu2+) for concentrations up to 1.0 mM. Furthermore, the bathochromic shift observed with the Hg2+ ion was insensitive to the presence of other cations in the solution, indicating that other metal cations do not interfere with the ability of AR-TiO2 to detect Hg2+.
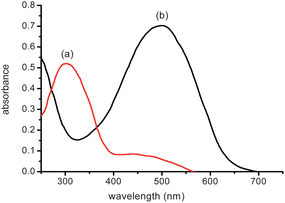 |
| Fig. 7 (A) Solid UV-vis spectra of AR-TiO2 films in the (a) absence and (b) the presence of HgCl2 (1.5 μM) aqueous solution. | |
The color change observed following the dipping of AR-TiO2 into Hg2+ solution (1.0 μM) was found to be fully reversible when AR-TiO2 was rinsed thoroughly with EDTA (10.0 μM). Reusability was evaluated by repeated dipping/rinsing cycles, with the AR-TiO2 absorption spectrum being recorded after each step. Typical data are shown in Fig. 8. The data show that AR-TiO2 exhibits excellent reusability, as almost no loss in AR-TiO2 sensitivity was observed after eight repeated dipping/rinsing cycles. In addition, the AR-TiO2 film before and after dipping of Hg2+ ion solution showed transparency with a deep red color (Fig. S14, ESI).†
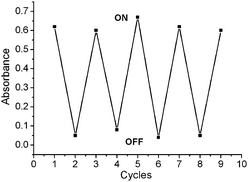 |
| Fig. 8 Plot for the absorbance of AR-SiO2 by alternated dipping in 1.0 μM aqueous solution of Hg2+ ion (“ON”) and 10.0 μM EDTA (“OFF”). The cyclic index is the number of alternating dipping/rinsing cycles, with the vertical axis showing the absorbance for the AR-SiO2 at 492 nm. | |
To develop the AR-TiO2 as a general Hg2+ ion sensor which is independent of any anions present, we also added the anions as I−, Br−, NO3− and ClO4−. With the anion addition, the color of the AR-TiO2 in HgCl2 solution still changed from yellow to deep red. This finding indicates that AR-TiO2 can be employed for the detection of Hg2+ independently of other anion(s) present.
Fig. S15 (ESI)† shows standard calibration data (Abs. vs. [Hg2+]) for AR-TiO2. A linear response is observed (between 28 nM and 1.0 μM) with a sensitivity of ∼28 nM. This sensitivity is much higher than those previously reported for spectrophotometric sensors anchored to mesoporous aluminosilicates.24 Furthermore, the sensitivity of the AR-TiO2 film for Hg2+ was much higher than that of AR-SiO2, because AR-TiO2 films are transparent and/or have higher immobilization of receptor onto TiO2 nanoparticles. These findings demonstrate that AR-TiO2 has strong potential as a portable chemosensor for Hg2+ ions.
AR-TiO2 film function as a pH buffer
We consider next the buffer action of the AR-TiO2 film. The AR-TiO2 film was immersed in aqueous solutions with pH values ranging between 1.0 and 11.0. Control experiments were conducted using 1.0 mM of Hg2+ solution controlling the pH range. The resulting film coloration is shown in Fig. 9. The absorbances of AR-TiO2 in the presence of Hg2+ ion were almost constant, with pH values ranging between 4 and 11. The absorbances of AR-TiO2 are independent between pH 4 and 11. We conclude that AR-TiO2 can reliably detect the Hg2+ ion over the wide pH range of pH 4–11.
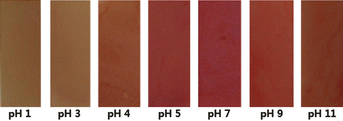 |
| Fig. 9 Color changes observed after AR-TiO2 films were dipped in Hg2+ aqueous solution (1.0 mM) at different pH values. | |
Conclusions
We have fabricated AR-SiO2 and AR-TiO2 using a functional azobenzene-coupled acyclic receptor. The AR-SiO2 recognized and separated Hg2+ with a high degree of selectivity among heavy metal ions in aqueous solution. Beyond its immediate applications in environmental cleanup, AR-SiO2 provides a unique opportunity to introduce molecular binding sites and to rationally design the surface properties of inorganic nanomaterials. Furthermore, AR-TiO2 films with 4 μm thickness on glass substrates were prepared as portable chemosensors for Hg2+ ion in aqueous solution. The AR-TiO2 films show excellent sensitivity and selectivity for Hg2+ ion. The AR-TiO2 film detected Hg2+ in pH = 7.4 with a sensitivity of 28 nM. The Hg2+ detection ability was unaffected by the presence of other cations or anions. The results imply that the AR-TiO2 film has strong potential as a portable colorimetric sensor for detection of Hg2+ in the environmental field. We believe the combination of well-defined inorganic nanomaterials and functionalized organic receptors can play a pivotal role in the development of a new generation of hierarchical structures and functionalized composites.
Acknowledgements
This work was supported by Korea Science and Engineering Foundation Grant (2009-0074100, 2008-2001014), and World Class University (WCU) Program supported from Ministry of Education, Science and Technology (R32-2008-000-20003-0), and Environmental-Fusion Project (191-091-004) supported from Ministry of Environment.
References
- G. K. Walkup and B. Imperiali, J. Am. Chem. Soc., 1996, 118, 3053 CrossRef.
- A. Miyawaki, J. Llopis, R. Helm, J. M. McCaffery, J. A. Adams, M. Ikura and R. Y. Tsien, Nature, 1997, 388, 882 CrossRef CAS.
- M. M. Henary and C. J. Fahrni, J. Phys. Chem. A, 2002, 106, 5210 CrossRef CAS.
- J. Homola, S. S. Yee and G. Gauglitz, Sens. Actuators, B, 1999, 54, 3 CrossRef.
- F. Turner, Science, 2000, 290, 1315 CrossRef CAS.
- P. Chen and C. He, J. Am. Chem. Soc., 2004, 126, 728 CrossRef CAS.
- S. J. Lee, J.–E. Lee, J. Seo, I. Y. Jeong, S. S. Lee and J. H. Jung, Adv. Funct. Mater., 2007, 17, 3441 CrossRef CAS.
- S. J. Lee, S. S. Lee, J. Y. Lee and J. H. Jung, Chem. Mater., 2006, 18, 4713 CrossRef CAS.
- E. Palomares, R. Vilar, A. Green and J. R. Durrant, Adv. Funct. Mater., 2004, 14, 111 CrossRef CAS.
- E. C. Lima, J. L. Brasil and A. H. D. P. Santos, Anal. Chim. Acta, 2003, 484, 233 CrossRef CAS.
- E. C. Lima, J. L. Brasil and J. C. P. dos Vaghetti, Talanta, 2003, 60, 103 CrossRef CAS.
- N. G. Becka, R. P. Franks and K. W. Bruland, Anal. Chim. Acta, 2002, 455, 11 CrossRef CAS.
- E. Palomares, R. Vilar and J. R. Durrant, Chem. Commun., 2004, 362 RSC.
- J. S. Wu, I.-C. Hwang, K. S. Kim and J. S. Kim, Org. Lett., 2007, 9, 907 CrossRef CAS.
- M. H. Lee, J. S. Wu, J. W. Lee and J. S. Kim, Org. Lett., 2007, 9, 2501 CrossRef CAS.
- E. M. Nolan and S. J. Lippard, Chem. Rev., 2008, 108, 3443 CrossRef CAS.
- S. O. Obare, R. E. Hollowell and C. J. Murphy, Langmuir, 2002, 18, 10407 CrossRef CAS.
- T. Balaji, S. A. El-Safty, H. Matsunaga, T. Hanaoka and F. Mizukami, Angew. Chem., Int. Ed., 2006, 45, 7202 CrossRef CAS.
- E. Palomares, M. V. Martínez-Díaz, T. Torres and E. Coronado, Adv. Funct. Mater., 2005, 15, 803 CrossRef CAS.
- R. Metivier, I. Leray, B. Lebeau and B. Valeur, J. Mater. Chem., 2005, 15, 2965 RSC.
- M. Boiocchi, M. Bonizzoni, L. Fabbrizzi, G. Piovani and A. Taglietti, Angew. Chem., Int. Ed., 2004, 43, 3847 CrossRef CAS.
- M. H. Lee, S. J. Lee, J. H. Jung and J. S. Kim, Tetrahedron, 2007, 63, 12087 CrossRef CAS.
- S. J. Lee, J. H. Jung, J. Seo, I. Yoon, K.-M. Park, L. F. Lindoy and S. S. Lee, Org. Lett., 2006, 8, 1641 CrossRef CAS.
- A. B. Descalzo, D. Jimenz, J. E. Haskouri, D. Beltran, P. Amoros, M. D. Marcos, R. Martinez-Manez and J. Soto, Chem. Commun., 2002, 562 RSC.
- E. Palomares, R. Vilar, A. Green and J. R. Durrant, Adv. Funct. Mater., 2004, 14, 111 CrossRef CAS.
- W. S. Han, H. Y. Lee, S. H. Jung, S. J. Lee and J. H. Jung, Chem. Soc. Rev., 2009, 38, 1904 RSC.
- H. J. Kim, S. J. Lee, S. Y. Park, J. H. Jung and J. S. Kim, Adv. Mater., 2008, 20, 3229 CrossRef CAS.
- H. Y. Lee, D. R. Bae, J. C. Park, H. Song, W. S. Han and J. H. Jung, Angew. Chem., Int. Ed., 2009, 48, 1239 CrossRef CAS.
- J. V. Ros-Lis, R. Casasús, M. Comes, C. Coll, M. D. Marcos, R. Martínez-Máñez, F. Sancenón, J. Soto, P. Amorós, J. E. Haskouri, N. Garró and K. Rurack, Chem.–Eur. J., 2008, 14, 8267 CrossRef CAS.
- D. Li, A. Wieckowska and I. Willner, Angew. Chem., Int. Ed., 2008, 47, 1 CrossRef.
Footnote |
† Electronic supplementary information (ESI) available: Measurement data. See DOI: 10.1039/b915975d |
|
This journal is © The Royal Society of Chemistry 2010 |
Click here to see how this site uses Cookies. View our privacy policy here.