DOI:
10.1039/B911672A
(Paper)
Analyst, 2010,
135, 121-126
An electrochemical molecular switch for one-step, reusable detection of a single-base mutation of DNA
Received
16th June 2009
, Accepted 28th October 2009
First published on
10th November 2009
Abstract
A new strategy for one-step, reusable and sensitive detection of a single-base mutation based on an electrochemical molecular switch is developed in the present work. When the hybridization reaction takes place in the presence of target DNA, the Fc-labeled terminal of the open switch molecule can be captured by the probe through the predesigned complementary bases of both sequences. By this method, a signal-on sensor featuring both generalizability and simplicity towards reagentless detection of DNA with sensitivity and selectivity electrochemical system is built on. The approach had been demonstrated with the identification of a single-base mutation of α-thalassemia point mutation in Hb Constant Spring codon 142 (TAA → CAA). The wild-type and mutant-type of the synthetic 16 mer DNA sequences as the model targets were successfully discriminated. The results showed that the response signal was linear to the logarithm of the target concentration in the range from 0.01 to 100 pM with a detection limit of 0.01 pM. The regeneration experiment demonstrated that the sensor interface can be easily and successfully regenerated. All these revealed that the present system is a promising candidate for single-base mutation discrimination.
1.0 Introduction
In human molecular biology and genetics, the vast majority of mutations and sequence polymorphisms in DNA result from single-base substitutions and insertions or deletions of bases in a genome. Single-base mutation (SBM) is the most abundant form of genetic variation and occurs once every 100–300 bases.1 Many pathogenic and genetic diseases are associated with changes in the sequence of particular genes.2 Identification of these single-base mutations can realize the medical diagnosis of the diseases. Up to now, many techniques have been developed for SBM detection. Conventional methods for small genetic alternation detection include denaturing gradient gel electrophoresis, enzyme or chemical mismatch cleavage, and direct sequencing of polymerase chain reaction products.3–5 These methods are time consuming and of relatively high cost, which renders most of them unsuitable for automatic and rapid screening for polymorphisms.
Other approaches for SBM detection are primarily built on the optical character changes2,6–8 or electrochemical analysis.9–11 Typically, these methods employ linear single-strand DNA probes for capturing targets in hybridization reactions.12,13 One shortcoming of this approach is that it requires target labeling, which costs time, money, and can increase the potential for errors in the analysis due to additional steps. In recent years, DNA hairpin probes, namely molecular beacons have been used to detect the presence of unlabeled target sequences in homogeneous solution with high discrimination; they have also been shown to be remarkably effective at detecting single-base mutations.8,10,14 Many of these approaches for SBM detection are primarily based on fluorescence quenching/enhancing (or energy transfer) as well as electrochemical analysis.
The electrochemical analysis-based approaches have attracted increasing interest due to its cost efficiency, ease of operation and rapidness of implementation. Moreover, the incorporation of molecular beacons offers additional advantages. DNA hairpins have been found to exhibit extraordinary stability, better selectivity, and higher specificity than similar assays performed using single-stranded DNA.15–17 The molecular beacons of ferrocene (Fc) moieties have been shown to be extremely useful for electrochemical detection of DNA hybridization, and protein binding.18–20 In general, molecular beacons are attached to solid substrates, which maintain the proper conformation for inhibition or promotion of the charge transfer by hybridization with target DNA. However, Binding events at surfaces are limited by mass transport; furthermore, for a surface-immobilized molecular beacon, in most situations it is designed as a signal-off biosensor, which is not a good choice for exhibiting a limited signal gain or suffers from the problem of substantial background signals.18,21,22
In the present approach, we have revealed a new scheme for electrochemical detection of the target DNA sequence using Fc-labeled oligonucleotides as a molecular switch, which is a signal-on sensor featuring both generalizability and simplicity in design towards reagentless detection of DNA. The operation principle and the fabrication of this molecular switch-based DNA sensor are shown in Scheme 1. The gold electrode was modified with a self-assembled monolayer of the capture probe. Then, the electrode was dipped into the hybridization solution, in which the Fc-labeled hairpin-like switch molecules were opened by hybridizing with the target DNA. Finally, the Fc-labeled terminal of the molecular beacon was captured by the capture sequence that immobilized on the electrode. As a consequence, a much higher response current was obtained than that before hybridization because the electrochemically active molecule, the Fc label, was brought into closer proximity with the electrode surface. The present approach has been demonstrated with identification of a single-base mutation in Hb Constant Spring codon 142 (terminating codon TAA → CAA) that is one of the major types of α-thalassemia point mutations for clinical diagnosis.23 The wild-type (single-base mismatch target) and the mutant-type (perfect matched target) of the synthetic 16 mer DNA sequences as the model targets were successfully discriminated. A distinctly strong peak current was obtained if a mutant target DNA sequence was used for hybridization. The result revealed that a sensitive and simple detection for SBM can be achieved using the present strategy.
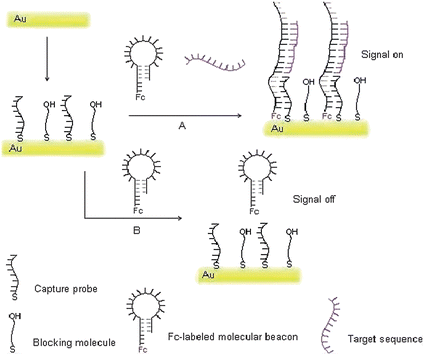 |
| Scheme 1 Schematic representation of the fabrication of the molecular switch for target DNA detection. A: the situation of the target DNA present. B: the situation of the target DNA absence. | |
2.0 Materials and methods
2.1 Chemicals
The oligonucleotides used in this study were obtained from Shanghai Generay Biotechnology Co. Ltd. (Shanghai, China) and were used as received with the sequences listed in Table 1. After the labeling of the amine terminated DNA with ferrocenecarboxylic acid (Fc–COOH), all oligonucleotide stock solutions were prepared with 0.15 M NaCl, 10 mM phosphate buffer solution (pH 7.4, hybridization buffer). Mercaptoethanol (MCE) was purchased from Sigma-Aldrich Inc. All other chemicals were of analytical grade and were used as received. Ultrapure water (electric resistance >18.3 MΩ) was obtained through a Nanopure Infinity ultrapure water system (Barnstead/thermolyne Corp, Dubuque, IA) and was used throughout the experiments.
Name |
Sequence (5′-3′) |
Tm |
The melting temperature of the capture probe/switch molecule.
The melting temperature of the switch molecule/mutant DNA sequence.
The melting temperature of the switch molecule/wild-type DNA sequence (in theory for 1 nM of both oligonucleotides).
|
Capture probe |
SH-(CH2)6-AGGCCGCAACG |
38 °Ca |
Molecular beacon |
GCAACGAAATACCGTCAAGCTGCGTTGCGGCCT-(CH2)3-NH2 |
38 °Ca |
Mutant Target |
CAGCTTGACGGTATTT |
42 °Cb |
Wild-type Target |
CAGCTTAACGGTATTT |
28 °Cc |
2.2 Apparatus
All electrochemical measurements were performed in a self-made measuring cell at ambient temperature using a CHI 760B electrochemical workstation (Shanghai Chenhua Instruments Co. Ltd., China). A conventional three-electrode system was employed: a gold disc electrode (polycrystalline gold rod, 99.99%, 2 mm diameter) as working electrode, a KCl saturated calomel electrode (SCE) as reference electrode, and platinum foil as auxiliary electrode. All potentials were referenced to the SCE electrode.
The electrochemical molecular beacon was produced by incorporation of a ferrocene label to the 3′-NH2-moiety of the oligonucleotide using the succinimide coupling (EDC-NHS) method.24 Briefly, 100 μL of 10 μM molecular beacon probe was mixed with 100 μL of 10 mM PBS (pH 7.4) containing 10 mM of ferrocenecarboxylic acid, 1 mM EDC, and 5 mM Sulfo-NHS, and incubated for 2 h at 37 °C. The conjugate was dialyzed against 10 mM PBS (1000 mL) for 12 h to remove excessive ferrocenecarboxylic acid.
2.4 Fabrication of DNA biosensor
The gold electrode was polished sequentially with 0.3 and 0.05 μm gamma alumina slurries on microcloth pads, was then sonicated in ultrapure water and ethanol respectively for 5 min to remove bound particles, and rinsed thoroughly with ultrapure water and dried in nitrogen stream. Then, the electrode was dipped in piranha solution (1
:
3 (v/v) mixture of 30% H2O2 and concentrated H2SO4. Warning: This solution reacts violently with organic materials and should be handled with great care.) for 30 min and was rinsed with ultrapure water and dried in a nitrogen stream. Afterwards, 20 μL of 1.0 μM capture probe was dropped onto the gold electrode and incubated overnight. After rinsing with water to remove the unbound oligonucleotides, 20 μL of 1.0 mM MCE was pipetted onto the resulting electrode and maintained for 30 min to block the blank sites. Finally, the electrode was rinsed with ultrapure water and ready for detection.
2.5 Measurement procedure
The capture probe-modified electrode was held upside down, covered with 30 μL hybridization solution containing the target DNA sequence and Fc-labeled molecular beacon, incubated for 90 min, followed by washing with ultrapure water. To evaluate the ability of the developed biosensor to identify a point mutation, a mutant target DNA sequence was used for hybridization. The electrochemical measurements were performed using differential pulse voltammetry (DPV) and cyclic voltammetry (CV) with a CHI 760B electrochemical workstation. A conventional three-electrode system comprising a KCl saturated calomel reference electrode (SCE), a platinum counter electrode and the working electrode was used for all electrochemical measurements in 5 mL of 10 mM phosphate buffer solution (pH 7.4) containing 0.1 M KClO4 at room temperature. The Faradic impedance measurement were performed in the presence of 5 mM K3[Fe(CN)6]/K4[Fe(CN)6] (1
:
1) mixture as a redox probe in 10 mM PBS (pH 7.4) containing 0.1 M KCl. The results were recorded in the frequency range from 1 Hz to 100 kHz at the bias potential of 0.24 V versus SCE, using an alternating voltage of 5 mV. All experiments were performed in triplicate measurements under the optimal condition.
After each assay, the electrode capturing the molecular switch can be easily regenerated by dipping into 1.0 M NaOH at 50 °C for 15 min. Then, the electrode was rinsed with ultrapure water and ready for another assay.
3.0 Results and discussion
3.1 Sensor fabrication and the probes design
Combining the advantages of the electrochemistry analysis and the molecular beacons for DNA detection,16–19 the molecular beacons of ferrocene (Fc) moieties have been used fabricating a signal-on sensor to detect DNA as well as to discriminate the perfect matched target and the single base mutant target. According to some of the literature, the method to immobilize molecular beacons to the electrode surface is not very satisfactory at enhancing the signal/noise ratio.18,21,22 Based on the above considerations, the present approach for detection of DNA has been fabricated using the molecular beacon as a switch in combination with the configuration change of DNA sequence by hybridization. Illustration of the strategy is shown in Scheme 1.
Obviously, the design of the capture probe and molecular beacon to ensure the smooth realization of the program is essential. In this study, A 16 mer of single base mutant in Hb Constant Spring codon 142 of α-thalassemia was taken as a target DNA sequence, and its' complementary sequence was adopted in the loop portion of the molecular beacon. There are 6 base-pairs in the stem portion of the molecular beacon, but another 5 free bases act as a tail sequence at one end of the stem labelled Fc. When the target sequence met with the Fc-labeled molecular beacon in hybridization solution, the original hairpin-like molecular switch would be turned on because of the hybridization proceeding. When the electrode modified with capture probe was dipped into the preceding hybridization solution, the Fc-labeled terminal of opened switch molecule is captured by the capture probe because that both sequences were complementary by 11 bases with a predesigned. Therefore, a much higher response current is obtained than that before hybridization because the electrochemically active molecule was brought in closer proximity to the electrode surface and ensured the efficient redox of the Fc. Worthy to be mentioned is that, either the switch molecule or the target DNA is absent, the molecular switch would not be opened, and the electrode could not capture it, of course no signal changed or enhanced. The probe, the beacon and the target oligonucleotide used in the experiment were listed in Table 1. The thermodynamic parameters of all oligonucleotides were calculated using bioinformatics software (http://www.bioin-fo.rpi.edu/applications/).25 The predicted secondary structure of the switch molecule was shown in Fig. 1. No significant folding structure was found for other oligonucleotides.
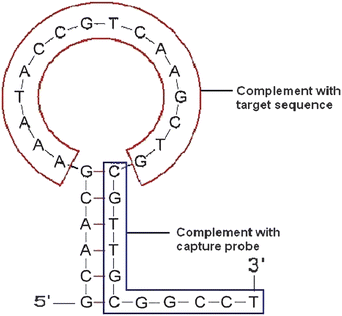 |
| Fig. 1 Secondary structure of the molecular beacon predict using bioinfomatics software.25 The 3′- terminal was Fc-labeled as a switch molecule in experiment. | |
3.2 Optimization of the experimental conditions
3.2.1 Effect of hybridization temperature.
Since the validity of the such-fabrication DNA sensor is dependent on following two factors: whether or not the target sequence hybridizes with the switch molecule and whether or not the hybridization molecule is captured by the probe on the electrode. In fact, these two factors are mainly affected by temperature control. The hybridization temperature of the system was investigated to ensure successful genotyping of SBM using the proposed strategy. A series of hybridization temperatures were evaluated. The maximum peak current and the better signal–noise ratio was observed at around 30 °C, which is between the melting temperature of the capture probe/switch molecule (Tm = 38 °C in theory for 1 nM switch molecule and 1 nM capture probe) and the melting temperature of the switch molecule/wild-type DNA sequence (Tm = 28 °C in theory for 1 nM DNA sequence and 1 nM switch molecule). When the hybridization temperature increased, the peak current presented obviously weak (as shown in Fig. 2A). If the temperature of the system is under the melting temperature of the wild-type sequence and switch molecule (Tm = 28 °C), or above the melting temperature of switch molecule and capture probe (Tm = 38 °C), then the molecule switch system would not give a good performance. Therefore, 30 °C was adopted as the optimal hybridization temperature for detection of the target DNA in all of the following experiments.
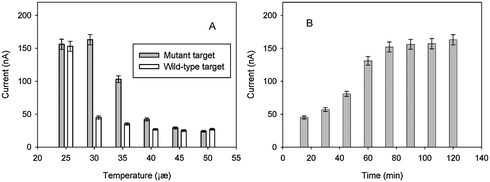 |
| Fig. 2 Optimization of experimental conditions: (A) Effect of hybridization temperature (the hybridization time was 90 min). (B) Effect of hybridization time for mutant target at 30 °C. The current presented in figures were measured from DPV, and the target DNA (mutant or wild-type) concentration is 1.0 nM for each test. | |
3.2.2 Effect of hybridization reaction time.
In order to obtain high sensitivity, the reaction time of hybridization was also investigated. After hybridizing, the temperature was allowed to maintain at 30 °C, every 15 min, one electrode was taken out and rinsed with water, then was used for electrochemical detection. The results are shown in Fig. 2B. As can be seen, the current intensity increased with the augmentation of the reaction duration owing to more switch molecule hybridizing with the target sequence and then combining with the capture probe on the electrode. The maximum current intensity was observed when the hybridizing reaction was maintained for about 90 min. However, when the reaction duration increased continuously, the current intensity did not increase.
3.2.3 Optimization of AC frequency.
To avoid the instability of ferrocenium (the oxidized form of the ferrocene), 1.0M NaClO4 solution was used as the supporting electrolyte for investigating the electrochemical behavior of the working electrode.16Fig. 3 shows the cyclic voltammograms for detection of 1.0 nM mutant-type target DNA at different scan rate. A marked signal of the redox response was clearly seen after the switch molecules were captured to the gold electrode surface. The roughly symmetrical shape of CV curve was also observed with an apparent formal potential of 0.25 V (estimated from E1/2 = (Ered + Eox)/2, where Ered and Eox were the reduction potential and the oxidation potential, respectively). The dependence of cyclic voltammograms on the scan rates was investigated, the peak current increased linearly with the potential scan rate, reflecting a surface-confined redox reaction only.
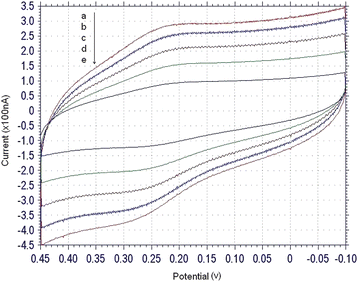 |
| Fig. 3 Electrochemical characterization: cyclic voltammograms, scan rate (v/s) = 0.1(a), 0.08 (b), 0.06 (c), 0.04 (d), 0.02 (e). The concentration of target sequences was 1.0 nM, and the electrolyte was 1.0 M NaClO4 solution. | |
3.3 Electrochemical response characteristics
Fig. 4 shows the typical characteristics of electrochemical response of the molecular switch system under the optimum experimental conditions. The availability of the DNA sensor was proved by the controlled trial experiment. As shown in Fig. 4, in the absence of target DNA, the current signal is very weak (column a in Fig. 4) because the tail of switch molecules does not associate with the surface-immobilized strands since the complementary fragment is too short to promote effective annealing. When the hybridization reaction takes place in the presence of target DNA, the Fc-labeled terminal of opened switch molecule can be captured by the capture probe because there are enough complementary bases to both sequences with predesigned. The signal is very strong (column b in Fig. 4). At the same time, the situations in switch molecule blank and un-labeled switch molecule were also investigated in controlled trial experiments (columns c and d); wild-type target (column e) was used as a replacement for mutant targets and the results were compared with each other. The signal is quite weak and which if fully consistent with the expected results. It is worth mentioning that the signal strength of the mutant target and wild-type target mixture is stronger but almost the same compared with the single mutant target (column f and b). The quantity of DNA in the mixture sample (the concentration is 2 nM) is double that of the single mutant target or the single wild-type target, however, the signal have not augmented accordingly. This result indicated that false positives or mis-hybridization could not swamp the real signal and the system is provided with a good performance.
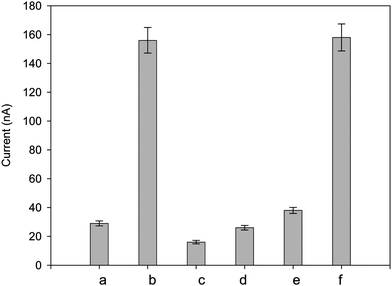 |
| Fig. 4 Characterization electrochemical response of differential pulse voltammetry: background (a), mutant target (b), switch molecule blank (c), using un-labeled switch molecule (d), wild-type target (e), the mixture of mutant target and wild-type target (f). The target DNA (mutant or wild-type) concentration is 1.0 nM for each test except a (target blank). | |
3.4 Impedance measurement
The impedance of each step for detection of the target DNA was also recorded as shown in Fig. 5. The same electrode was used for the electrochemical Faradic impedance measurement in each step. The impedance spectra were plotted in the form of plane diagrams (Nyquist plots). The results obtained from the impedance measurement further confirmed the success in the design of the proposed sensing system. Relatively lower impedance for the bare gold surface could be observed in Nyquist plots (a), while the immobilization of the capture sequences significantly increases the impedance of the electrode (b). When the target DNA hybridized with the capture sequences, a moderate decrease of impedance was observed (c). This might attribute to the fact that duplex structure of oligonucleotide facilitated the conductivity of electron toward the electrode surface. After regeneration, the impedance of the electrode recovered to the value after immobilizing the capture sequences (d).
3.5 Analytical performance
The developed DNA sensor demonstrated a signal-on architecture in response to the target. In the absence of the target DNA, only insignificant signals were observed. After reaction with various concentrations of target DNA under the optimum experimental conditions, the differential pulse voltammograms provided a nice resolution of the response. Using the described strategy, a series of different concentrations of target DNA were measured. The calibration curve was plotted in Fig. 6. The results showed that the current intensity was linear to the logarithm of the target concentration in the range from 0.01 to 100 pM, the square of linear correlation coefficient is 0.9777 with a detection limit 0.01 pM (from the three times of standard deviation corresponding to the blank sample measurement).
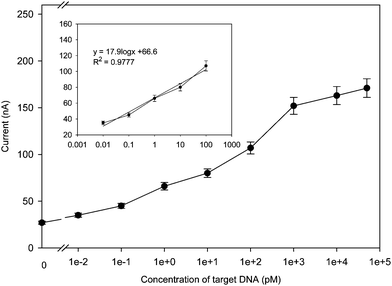 |
| Fig. 6 Calibration curve and linear relationship (inset) between the current response and concentration of mutant target under the optimized condition, the curves are expressed as the current signal against the logarithm of target DNA concentration. | |
Good reusability of a proposed sensor is critically important for the practical applications such as clinical diagnoses and biological monitoring. Because this molecule switch system only included a surface-tethered monolayer of oligonucleotides without intricate conformation, the sensor interface could be readily regenerated. In this study, 1 M NaOH were employed as the denaturing reagents. It was found that the DNA sensor could be reused over seven times without significant loss of sensitivity via immersed into NaOH solution for 10 min at 50 °C. Presumably, the duplex structure might be collapsed in high alkaline solution due to the break of hydrogen bonds. The sensor response to 1.0 nM mutant target still was able to maintain 90% the value of the original measurement after seven experiments, indicating that the presented sensor could be easily and successfully regenerated.
4.0 Conclusion
In the present approach, we have demonstrated a novel scheme for one-step, reusable and sensitive detection of the target DNA sequence using Fc-labeled oligonucleotide as an electrochemically molecular switch. According to the predesign, when the electrode was dipped into the hybridization solution, in which the Fc-labeled hairpin-like molecular switch was opened by hybridizing with the target DNA. So, the Fc-labeled terminal of the molecular beacon was captured and a signal-on electrochemical system was built on. The approach had been demonstrated with the identification of a single-base mutation of α-thalassemia point mutation in Hb Constant Spring codon 142 (terminating codon TAA → CAA). The wild-type (the single base mismatch target) and mutant type (the perfect matched target) of the synthetic 16 mer DNA sequences as the model targets were successfully discriminated. So much so as to the mutant target and wild-type target mixture sample, the signal strength is almost the same when compared with the single mutant target indicating that false positives or mis-hybridization could not swamp the real signal. After reaction with various concentrations of target DNA under the optimum experimental conditions, the calibration curve was plotted. The results showed that the current intensity was linear to the logarithm of the target concentration in the range from 0.01 to 100 pM with a detection limit 0.01 pM. The experiment found that the DNA sensor could be reused via immersed into NaOH solution to be easily and successfully regenerated. All of these features revealed that the system is a promising candidate for single-base mutation discrimination, owing the advantages of both generalizability and simplicity toward reagentless detection of DNA with sensitivity and selectivity.
Acknowledgements
This study was financially supported by the National Natural Science Foundation of China (Grants No. 20375012, 90817101, 20775023) and “973” National Basic Research Program of China (No. 2007CB310500).
References
- R. Sachidanandam, D. Weissman, S. C. Schmidt, J. M. Kakol, L. D. Stein, G. Marth, S. Sherry, J. C. Mullikin, B. J. Mortimore and D. L. Willey, Nature, 2001, 409, 928 CrossRef.
- M. B. Wabuyele, H. Farquar, W. Stryjewski, R. P. Hammer, S. A. Soper, Y. W. Cheng and F. J. Barany, J. Am. Chem. Soc., 2003, 125, 6937 CrossRef CAS.
- K. J. Livak, J. Marmaro and A. J. Todd, Nat. Genet., 1995, 9, 341 CrossRef CAS.
- D. Schmalzing, A. Belenky, M. A. Novotny, L. Koutny, O. Salas-Solano, S. El-Difrawy, A. Aram, P. Matsudaira and D. Ehrlich, Nucleic Acids Res., 2000, 28, e43 CrossRef CAS.
- M. M. Mhlanga and L. Malmberg, Methods, 2001, 25, 463 CrossRef CAS.
- J. S. Li, X. Chu, Y. L. Liu, J. H. Jiang, Z. M. He, Z. W. Zhang, G. L. Shen and R. Q. Yu, Nucleic Acids Res., 2005, 33, 19 CAS e168.
- H. Ichinose, M. Kitaoka, N. Okamura, T. Maruyama, N. Kamiya and M. Goto, Anal. Chem., 2005, 77(21), 7047 CrossRef CAS.
- A. G. Frutos, S. Pal, M. Quesada and J. Lahiri, J. Am. Chem. Soc., 2002, 124, 2396 CrossRef CAS.
- S. Kumamoto, M. Watanabe, N. Kawakami, M. Nakamura and K. Yamana, Bioconjugate Chem., 2008, 19(1), 65 CrossRef CAS.
- Z. S. Wu, J. H. Jiang, G. L. Shen and R. Q. Yu, Hum. Mutat., 2007, 28(6), 630 CrossRef CAS.
- Y. T. Long, C. Z. Li, T. C. Sutherland, H. B. Kraatz and J. S. Lee, Anal. Chem., 2004, 76, 4059 CrossRef CAS.
- M. C. Pirrung, Angew. Chem., Int. Ed., 2002, 41, 1276 CrossRef.
- F. Patolsky, A. Lichtenstein and I. Willner, J. Am. Chem. Soc., 2001, 123, 5194 CrossRef CAS.
- G. Bonnet, S. Tyagi, A. Libchaber and F. Kramer, Proc. Natl. Acad. Sci. U. S. A., 1999, 96, 6171 CrossRef CAS.
- I. Willner, Science, 2002, 298, 2407 CrossRef CAS.
- C. H. Fan, K. W. Plaxco and A. J. Heeger, Proc. Natl. Acad. Sci. U. S. A., 2003, 100, 9134 CrossRef CAS.
- P. V. Riccelli, F. Merante, K. T. Leung, S. Bortolin, R. L. Zastawny, R. Janeczko and A. S. Benight, Nucleic Acids Res., 2001, 29, 996 CrossRef CAS.
- C. E. Immoos, S. J. Lee and M. W. Grinstaff, J. Am. Chem. Soc., 2004, 126, 10814 CrossRef CAS.
- C. J. Yu, Y. Wan, H. Yowanto, J. Li, C. Tao, M. D. James, C. L. Tan, G. F. Blackburn and T. J. Meade, J. Am. Chem. Soc., 2001, 123, 11155 CrossRef CAS.
- F. L. Floch, H. A. Ho and M. Leclerc, Anal. Chem., 2006, 78, 4727 CrossRef CAS.
- F. J. Steemers, J. A. Ferguson and D. R. Walt, Nat. Biotechnol., 2000, 18, 91 CrossRef CAS.
- T. Heinlein, J. P. Knemeyer, O. Piestert and M. Sauer, J. Phys. Chem. B, 2003, 107, 7957 CrossRef CAS.
- B. C. Ye, Z. F. Zhang and Z. S. Lei, J. Biotechnol., 2005, 115, 1 CrossRef CAS.
- R. P. Fahlman and D. Sen, J. Am. Chem. Soc., 2002, 124, 4610 CrossRef CAS.
- N. R. Markham and M. Zuker, Nucleic Acids Res., 2005, 33, W577 CrossRef CAS.
|
This journal is © The Royal Society of Chemistry 2010 |