DOI:
10.1039/B910557C
(Paper)
Analyst, 2010,
135, 116-120
A sensitive electrochemical approach for monitoring the effects of nano-Al2O3 on LDH activity by differential pulse voltammetry†
Received
28th May 2009
, Accepted 28th October 2009
First published on
9th November 2009
Abstract
In this paper, a sensitive electrochemical approach for monitoring the effect of nano-Al2O3 on lactate dehydrogenase (LDH) activity is established. It is based on the determination of reduction current of NAD+ involved in enzyme promoting catalytic reaction of “
” by differential pulse voltammetry (DPV). Various influencing factors including nano-type, nano-size, and adsorbed pollutant organics have been investigated. The experimental results show that the proposed electrochemical method is useful in monitoring and evaluating the toxic effects of nanoparticles, which might be suitable to the environmental pollutant's toxicity analysis.
1. Introduction
With the development of industry and agriculture, nanomaterials have been widely applied in various fields.1–3 However, the potential toxic effects of them have received great concerns in recent years.4–8 Nano-Al2O3 is one of the functional materials possessing superior structural, mechanical, and optical properties.9 It has been widespread applied in the manufacturing of ceramic-, optical-, semiconductor-, and surface protection materials, as well as catalyst carrier or catalyst. The evidence is increasing that nano-Al2O3 may also result in similarly unique nanotoxicological effects,10 and its toxic effect is changed with nano-size, shape, and the surface chemistry of various nano-Al2O3 nanomaterials.11–14 Due to the distinct properties of nanomaterials, there is a lack of a recognized method for evaluating their health and safety concerns, resulting in the controversial results for similar experiments carried out under different conditions. Hence, the development of effective analytical methods for evaluating the toxic effects and detecting the harmful effects of nanomaterials is important.
Biomarkers have been widely demonstrated to be able to evaluate and monitor the changes and toxic effects of environmental pollutants.15 Lactate dehydrogenase (LDH) is one of the most important enzymes, found in the liver, lungs, heart, and various other tissues, and plays a significant role in energy metabolism. LDH activity can be used to indicate several pathological conditions, such as gastric cancer,16 breast cancer,17 lung damage,18 and thrombotic thrombocytopenic purpura,19etc. Thus, LDH activity also has been recognized as a useful biomarker and applied in the fields of biology, medicine, and environment.20,21
Up to now, the main analytical methods used in detecting the nanotoxicological effects are: colorimetric assay, microscopy, single cell microgel electrophoresis, cell counting, fluorescent probe, and Fourier transformed infrared spectrometry, etc.22–27 These methods usually have some limitations in sensitivities. The electrochemical technique possesses some distinct advantages: rapidity, high sensitivity, cheap instrumentation and a simple operation procedure. On the other hand, hanging mercury drop electrode (HMDE) exhibits the prominent merit of regenerating the electrode surface easily which may avoid the serious adsorption of pollutants on the electrode surface efficiently. It could satisfy many requirements to analyze different biological systems and be widely applied to detect enzyme activity.28–31 In this paper, LDH activity is used as a biomarker and an electrochemical method for monitoring the toxic effect of nano-Al2O3 on LDH activity is established.
2. Experimental
2.1 Materials and instrumentations
Most chemicals were obtained from Shanghai Chemical Reagent Factory and used as received unless otherwise noted. All chemicals were of analytical reagent grades. Bovine heart LDH (10 mg mL−1) was obtained from Sigma Co. (St. Louis, MO), stored in a refrigerator of 4 °C, and diluted 100 times when used. β-NAD+ and NADH (purity 90%) were purchased from Shanghai Bio Life Science & Technology Co., Ltd (China). Pyruvic acid (Pyr, 98.50%) was of biological-reagent grade, purchased from Shanghai Chemical Reagent Factory. NADH (0.05 mol L−1) and Pyr (0.2 mol L−1) solutions were prepared with double-distilled water, and stored in a refrigerator of 4 °C. Nano-Al2O3 was purchased from Sigma. Nano-AlN is synthesized according to ref. 32. The sizes of nano-Al2O3 were in 20–50, 300–500, above 500 nm respectively and nano-AlN in 200–400 nm. The 0.1 mol L−1 pH 7.5 Tris-HCl buffer solutions were prepared as that described in ref. 33. The supporting electrolyte is 0.15 mol L−1 KCl. All dilute solutions were prepared by diluting this solution with double-distilled water.
A three-electrode system was used, which consisted of a HMDE (Jingsu Electroanalytical Instrument Factory, China), a platinum foil counter electrode, and a saturated calomel reference electrode. All electrochemical experiments were performed with a CHI660B electrochemical system (CH instruments Inc., Shanghai, China). DPV parameters were the following: scan rate 20 mV s−1, pulse amplitude 50 mV, pulse width 50 ms. pH measurements were carried out with a PHS-2F digital ion meter (Shanghai Precision and Scientific Instrument Company, China). The solutions were stirred with a 79-1 magnetic stirrer (Guohua Instrument Factory, China) and all the experiments were carried out with 501 superthermostatic bath maintaining 25 ± 1 °C all through the experiment (Shanghai Laboratory Instrument Company, China). The dispersions of all nanoparticles in aqueous solutions are good.
2.2 Test for the effect of nano-Al2O3 on lactate dehydrogenase activity in LDH reaction system
Twenty-five millilitres of 0.10 mol L−1 Tris-HCl buffer solution and a quantitative amount of nano-Al2O3 were transferred into the electrolytic cell. The solution was degassed for 10 min by bubbling of nitrogen gas through it and kept in the ambient of nitrogen. After injecting LDH, the solution was stirred for 5 min (with or without nanoparticles). Then 100 μL of 0.2 mol L−1 Pyr and 100 μL of 0.05 mol L−1 NADH were added into the 25 mL electrolyte cell, and their final concentrations were 8 × 10−4 mol L−1 and 2 × 10−4 mol L−1, respectively. The reduction currents of NAD+ (ip,NAD+) and Pyr (ip,Pyr) were recorded in DPV mode at regular time intervals.
2.3 Examination of the adsorption of nano-Al2O3 on the HMDE
Twenty-five millilitres of 0.10 mol L−1 Tris-HCl buffer solution was transferred into the electrolytic cell, degassed for 10 min by bubbling of nitrogen gas, then stirred quickly for 2 min and sonicated for 10 min. And the cell was sealed so that a positive pressure of nitrogen could be maintained over the surface of the samples. After adding certain amount of Pyr, the reduction peak current was recorded in DPV mode, and then certain amount of nano-Al2O3 was added in cell. After stirring for 10 min and with an equilibration time of 1 min, DPV peak current of Pyr was recorded again. A series of similar tests for different concentrations of Pyr and in the presence of nano-Al2O3 were carried out. Similar experiments for NAD+ in the presence and absence of nano-Al2O3 were also performed.
2.4 Determination of Michaelis constant Km and maximum velocity vmax for NADH
Twenty-five millilitres of 0.1 mol L−1 Tris-HCl buffer solution was treated as above. After adding 30 μL LDH (diluted 100 times), the solution was stirred for 5 min (with or without 0.25 mmol L−1 nanoparticles), and then 100 μL of 0.2 mol L−1 Pyr (0.8 mmol L−1) and NADH (the concentration was varied) were added. The reduction currents of NAD+ (ip, NAD+) were recorded in DPV mode. The Michaelis constant Km and maximum velocity vmax for NADH were calculated by using the following formula:34
3. Results and discussion
3.1 The effects of nano-Al2O3 on LDH activity in the LDH reaction system
In the LDH, NADH, Pyr, and nano-Al2O3 reaction system, only the substrates Pyr and NAD+ yield electrochemical responses at HMDE.35,36 When NADH was added, the peak current of NAD+ at −0.89 V (vs. SCE, pH 7.5) was increased continually while the peak current of Pyr decreased constantly along with the time (Fig. 1). The peak current of NAD+ increased until it arrived at the plateau. Within the first 3 min of the enzyme reaction progress, there were positive linear relationships between the peak currents ip,NAD+ and the time. ip,NAD+ at 3 min was recorded and used to indicate LDH activity and to represent the initial velocity v, since ip,NAD+ can indicate LDH activity simply and conveniently. Thus, the effect of nano-Al2O3 on LDH activity in the LDH reaction system can be easily inspected by adding certain amounts of nano-Al2O3 (50 nm). Insert in Fig. 1 is the changes of ip,NAD+ after adding different levels of nano-Al2O3 (50 nm), indicating that the LDH activity decreases with the elevation of nano-Al2O3 (50 nm).
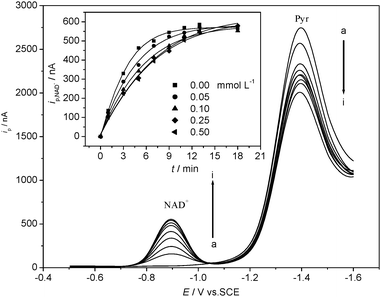 |
| Fig. 1 DPV responses of LDH reaction system changed with time in presence of 1× 10−3 mol L−1 nano-Al2O3 (50 nm). a → i: t = 0, 1, 3, 5, 7, 9, 11, 13, 18 min. T = 25 ± 1 °C, 0.10 mol L−1 Tris-HCl buffer solution (pH 7.5) + 0.15 mol L−1 KCl, 8.0 × 10−4 mol L−1 Pyr, 2.0 × 10−4 mol L−1 NADH and 30 μL LDH. Insert: the changes of ip,NAD+ after adding different levels of nano-Al2O3 (50 nm). | |
The adsorption effect of nano-Al2O3 on the HMDE was investigated. There are five species in enzymatic reaction system (LDH, NADH, Lac, Pyr, and NAD+). The adsorption of NAD+, Pyr, NADH, Lac, and LDH on HMDE have been reported to be weak in a short interaction time within 15 min.37,38 Thus, only the adsorption effect of nano-Al2O3 on the HMDE was considered. Experimental results indicate that in the absence and presence of nano-Al2O3, the peak currents of Pyr and NAD+ almost have no change in both cases (Fig. S1 in ESI†). In pH 7.5 buffer solution, both HMDE and Al2O3 possess negative charge,39 and the experimental time is also short (3 min). Thus, the adsorption of nano-Al2O3 on HMDE is weak, and nano-Al2O3 will not block the HMDE surface, and the changes of DPV responses mainly reflect the influences of nano-Al2O3 on LDH activity.
3.2 Effects of nano-Al2O3 on LDH activity with different types and sizes
As shown in Fig. 2, nano-Al2O3 inhibits the activity of LDH obviously, whereas nano-AlN shows weak inhibitory effects. It was found that under the same conditions, for the three kinds of Al species (Al(III) (data cited from ref. 38, 40), Al2O3, and AlN), low level Al(III) has activation effect on the LDH. When the Al(III) level is above 0.5 mmol L−1, the effect increased; When their concentration is 1 mmol L−1, nano-Al2O3 (50 nm) has the most serious impact on LDH activity and shows the order: nano-Al2O3 (50 nm) > nano-AlN > Al(III).
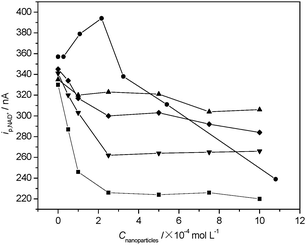 |
| Fig. 2 The effect of different nanoparticles and size on the reaction rates of LDH reaction system. ■ nano-Al2O3-50 nm; ▼ nano-Al2O3-300 nm; ◆ nano-Al2O3-1000 nm; ▲ nano-AlN-200–350 nm; ● Al(III). Other experimental conditions are the same as in Fig. 1. | |
Nano-size is a key influencing factor on its toxicity.41–44 In the present investigation, the experimental results indicate that the effects of nano-Al2O3 on LDH activity increased with the decrease of its size, as shown in Fig. 2 and 3 (the Lineweaver–Burk plots). This indicates that Al2O3 at nanometre level may have more toxicological effects. The Lineweaver–Burk plots and the values of Km and vmax in the absence and presence of 2.5 × 10−4 mol L−1 nanoparticles show that the inhibitions caused by nanoparticles are all of mixed type. Furthermore, it is interesting to note that the inhibition of ZnS (6–8 nm) and TiO2 (25–30 nm), which have small size, are competitive and non-competitive character but nano-Al2O3-300 nm and AlN (200–350 nm), which have larger sizes, are non-competitive and uncompetitive type. This result might imply that the size of particle has influence on the inhibition mechanism. Table 1 indicates that the inhibiting effect of nano-Al2O3 is close to that of TiO2,38a ZnS,38a and SWCNTs,45 but is weaker compared with that of Al(III),38b and nano-Al13.40 The effect trend is greater for smaller diameter Al2O3. In this case, the toxic mechanism may be due to some sort of steric access issue with the nanoparticles' small size effect. Some literature contributions to tackle this issue.46 Previous studies suggest that the mechanism of nanoparticles toxicity may relate to their photosensitivity and to production of reactive oxygen species under specific wavelength high-intensity light.46b,46c Nanoparticles are very small with large surface area, so that they can penetrate cell membranes and barriers.38a,46 Thus, the detailed information is unclear and deserves further studies.
Table 1 The effects of nano-Al2O3 and AlN on vmax and Km of LDH and comparisons with other known inhibitors of LDHa
|
v
max (μmol (L min)−1) |
K
m/μmol L−1 |
It should be pointed out that this comparison only gives a reference since their experimental conditions are different.
This paper. 0.10 mol L−1 Tris-HCl buffer solution (pH 7.5) + 0.15 mol L−1 KCl, 8.0 × 10−4 mol L−1 Pyr,2.0 × 10−4 mol L−1 NADH, 30 μL LDH (1 : 100).
Ref. 38a. 0.10 mol L−1 Tris-HCl buffer solution (pH 7.5) + 0.15 mol L−1 KCl, 8.0 × 10−4 mol L−1 Pyr, 2.0 × 10−4 mol L−1 NADH, 30 μL LDH (1 : 100).
Ref. 38b. 0.10 mol L−1 Tris-HCl buffer solution (pH 7.5) + 0.15 mol L−1 KCl, 8.0 × 10−4 mol L−1 Pyr, 2.0 × 10−4 mol L−1 NADH, 30 μL LDH (1 : 100).
Ref. 40,4b. 0.10 mol L−1 Tris-HCl buffer solution (pH 7.5) + 0.15 mol L−1 KCl, 8.0 × 10−4 mol L−1 Pyr, 2.0 × 10−4 mol L−1 NADH, 30 μL LDH (1 : 100).
Ref. 45. 50 mmol L−1 Tris-HCl buffer solution (pH 7.5), 5 × 10−4 mol L−1 Pyr, 5 × 10−5 mol L−1 NADH, 2 μg ml−1 LDH, GCE: glassy carbon electrode.
|
Al2O3 (mmol L−1)b |
|
|
0 |
89 |
134 |
0.25 (50 nm) |
58 |
106 |
0.25 (300 nm) |
61 |
109 |
0.25 (1000 nm) |
76 |
138 |
AlN/mmol L−1b |
|
|
0.25 (200–350 nm) |
80 |
124 |
TiO2(A) (mmol L−1)c |
|
|
0.25 (25–30 nm) |
55.2 |
177 |
TiO2(R) (mmol L−1)c |
|
|
0.25 (25–30 nm) |
66.7 |
156 |
ZnS/mmol L−1c |
|
|
0.25 (6–8 nm) |
37.7 |
140 |
Al(III) (mmol L−1)d |
|
|
0 |
38.8 |
85.7 |
0.04 |
28.8 |
107 |
Nano-Al13(mg L−1)e |
|
|
0 |
36 |
85 |
60 (200–500 nm) |
25 |
60 |
120 (200–500 nm) |
23 |
61 |
SWCNTs/mg L−1f |
|
|
0 (bare GCE) |
110 |
100 |
35 (SWCNTs modified GCE) (1.3 nm) |
185 |
75 |
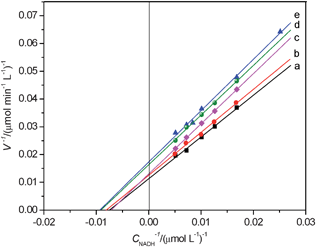 |
| Fig. 3 Double-reciprocal plot of v−1vs. CNADH−1. a. Cnanoparticles = 0 mol L−1; b. CAlN(200–350 nm) = 0.25 mmol L−1; c. CAl2O3 (1000nm) = 0.25 mmol L−1; d. CAl2O3 (300nm) = 0.25 mmol L−1; e. CAl2O3 (50 nm) = 0.25 mmol L−1. Other experimental conditions are the same as in Fig. 1. | |
3.3 Effects of nanoparticles loading phenol on LDH activity
It was suggested that the toxicity of nanoparticles is not only from their own harmful nature but also from the toxic substances adsorbed by them. Studies have evidenced that nanoparticles can act as vehicles transporting toxic chemicals into the human respiratory system,47–49 and the nanoparticles may be more dangerous in the presence of pollutants. Thus, the research on this subject is very valuable. In the present study, we stirred the mixture of nano-Al2O3 (50 nm, 1000 nm) and phenol for 30 min for full adsorption, respectively. As shown in Fig. 4, the inhibitory effects of phenol were enhanced after adsorbing by two sizes of Al2O3, demonstrating clearly the enhanced effect of nanoparticles on the pollutants' toxicity. This indicates that they may have a synergistic effect.
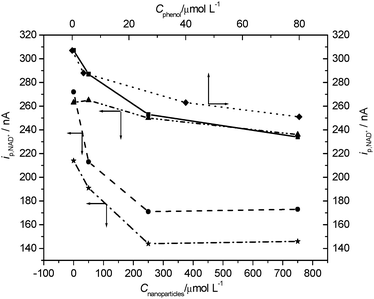 |
| Fig. 4 The effects of phenol and nano-Al2O3 on LDH (expressed as ip,NAD+ at 3 min). ■ Effects of nano-Al2O3 (1000 nm);▲effects of nano-Al2O3 (1000 nm) loading 40 μmol L−1 phenol; ● effects of nano-Al2O3 (50 nm); ★ effects of nano-Al2O3 (50 nm) loading 40 μmol L−1 phenol; ◆ effects of phenol concentration. Other experimental conditions are the same as in Fig. 1. | |
4. Conclusions
It has been demonstrated that the electrochemical technique can be a useful tool for evaluating the toxic effects of the nanoparticles through monitoring LDH activity simply by observing the DPV reduction peak current of NAD+ at the HMDE. Considering the significance of the LDH in the area of medicine, biology, and biomarkers for environment, this method can be conveniently applied to evaluate the toxic effects of nanomaterials on environment. It could be anticipated to satisfy many requirements for analyzing different biological systems and be applied to study the nano-toxicity just simply through detecting enzyme activity by constructing small and portable biosensors in SECM detections.50,51
Acknowledgements
This project is supported by the NSFC (20777030 & NFFTBS-J0630425), State Key Laboratory of Electrochemistry of China in Changchun Applied Chemistry Institute (2008008), and Analytical Center of Nanjing University.
References
- A. Saxena, B. Singh, A. K. Srivastava, M. V. S. Suryanarayana, K. Ganesan, R. Vijayaraghavan and K. K. Dwivedi, Microporous Mesoporous Mater., 2008, 115, 364 CrossRef CAS.
- M. Yoshida and J. Lahann, ACS Nano, 2008, 2, 1101 CrossRef CAS.
-
(a) W. H. Casey, Chem. Rev., 2006, 106, 1 CrossRef CAS;
(b) Z. S. Qian, H. Feng, W. J. Yang, Q. Miao, L. N. He and S. P. Bi, Chem. Commun., 2008, 3930 RSC;
(c) Z. S. Qian, H. Feng, W. J. Yang and S. P. Bi, J. Am. Chem. Soc., 2008, 130, 14402 CrossRef CAS.
-
(a) O. Deschaume, K. L. Shafran and C. C. Perry, Langmuir, 2006, 22, 10078 CrossRef CAS;
(b) J. J. Cheng, D. Q. Huang, J. Zhang, W. J. Yang, N. Wang, Y. B. Sun, K. Y. Wang, X. Y. Mo and S. P. Bi, Analyst, 2009, 134, 1392 RSC;
(c)
C. Exley, Aluminium and Alzheimer's-The Science that Describes the Link, Elsevier. Amsterdam, 2001 Search PubMed.
- A. Nel, T. Xia, L. Madler and N. Li, Science, 2006, 311, 622 CrossRef CAS.
- A. D. Maynard, R. J. Aitken, T. Butz, V. Colvin, K. Donaldson, G. Oberdörster, M. A. Philbert, J. Ryan, A. Seaton, V. Stone, S. S. Tinkle, L. Tran, N. J. Walker and D. B. Warheit, Nature, 2006, 444, 267 CrossRef CAS.
- Y. Li, G. Y. Peng, H. J. He, Y. K. Qiao, Q. Chang and X. Yang, Asian J. Ecotoxicology (Chinese), 2006, 1, 357 Search PubMed.
- T. Xia, M. Kovochich, M. Liong, J. I. Zink and A. E. Nel, ACS Nano, 2008, 2, 85 CrossRef CAS.
- A. K. Gianotto, J. W. Rawlinson, K. C. Cossel, J. E. Olson, A. D. Appelhans and G. S. Groenewold, J. Am. Chem. Soc., 2004, 126, 8275 CrossRef CAS.
-
(a) L. Yang and D. J. Watts, Toxicol. Lett., 2005, 158, 122 CrossRef CAS;
(b) N. Wang, J. J. Cheng, Y. Jin, K. A. Yao and S. P. Bi, Asian J. Ecotoxicology (Chinese), 2007, 2, 252 Search PubMed.
- K. A. D. Guzman, M. R. Taylor and J. F. Banfield, Environ. Sci. Technol., 2006, 40, 1401 CrossRef.
- T. Xia, M. Kovochich, J. Brant, M. Hotze, J. Sempf, T. Oberley, C. Sioutas, J. I. Yeh, M. R. Wiesner and A. E. Nel, Nano Lett., 2006, 6, 1794 CrossRef CAS.
- D. Y. Lyon, L. K. Adams, J. C. Falkner and P. J. J. Alvarez, Environ. Sci. Technol., 2006, 40, 4360 CrossRef CAS.
- N. W. S. Kam, T. C. Jessop, P. A. Wender and H. J. Dai, J. Am. Chem. Soc., 2004, 126, 6850 CrossRef CAS.
-
L. Lagadic, T. Caquet, J. C. Amiard and F. Ramade, Use of Biomarkers for Environmental Quality Assessment, CRC Press, 2000, pp. 2 Search PubMed.
- Y. Kolev, H. Uetake, Y. Takagi and K. Sugihara, Ann. Surg. Oncol., 2008, 15, 2336 Search PubMed.
- R. Coleman, J. Brown and R. Cook, Cancer Treat. Rev., 2008, 34, S78.
- M. Drent, N. A. M. Cobben, R. F. Henderson, E. F. M. Wouters and M. Van Dieijen-Visser, Eur. Respir. J., 1996, 9, 1736 CrossRef CAS.
- J. A. Cohen, M. E. Brecher and N. Bandarenko, J. Clin. Apheresis, 1998, 13, 16 CrossRef CAS.
- A. D. Ellington and J. J. Bull, Science, 2005, 310, 454 CrossRef CAS.
- J. E. Basner and S. D. Schwartz, J. Am. Chem. Soc., 2005, 127, 13822 CrossRef CAS.
- S. K. Manna, S. Sarkar, J. Barr, K. Wise, E. V. Barrera, O. Jejelowo, A. C. Rice-Ficht and G. T. Ramesh, Nano Lett., 2005, 5, 1676 CrossRef CAS.
- C. M. Sayes, J. D. Fortner, W. Guo, D. Lyon, A. M. Boyd, K. D. Ausman, Y. J. Tao, B. Sitharaman, L. J. Wilson, J. B. Hughes, J. L. West and V. L. Colvin, Nano Lett., 2004, 4, 1881 CrossRef CAS.
- A. Hoshino, K. Fujioka, T. Oku, M. Suga, Y. F. Sasaki, T. Ohta, M. Yasuhara, K. Suzuki and K. Yamamoto, Nano Lett., 2004, 4, 2163 CrossRef CAS.
- C. Kirchner, T. Liedl, S. Kudera, T. Pellegrino, A. M. Javier, H. E. Gaub, S. Stolzle, N. Fertig and W. J. Parak, Nano Lett., 2005, 5, 331 CrossRef CAS.
- L. H. Ding, J. Stilwell, T. T. Zhang, O. Elboudwarej, H. J. Jiang, J. P. Selegue, P. A. Cooke, J. W. Gray and F. Q. F. Chen, Nano Lett., 2005, 5, 2448 CrossRef CAS.
- J. R. Gurr, A. S. S. Wang, C. H. Chen and K. Y. Jan, Toxicology, 2005, 213, 66 CrossRef CAS.
- S. Serban and N. El Murr, Electrochim. Acta, 2006, 51, 5143 CrossRef CAS.
- A. S. Santos, A. C. Pereira, N. Duran and L. T. Kubota, Electrochim. Acta, 2006, 52, 215 CrossRef CAS.
- L. Yang, Z. B. Yang, M. Zhang, H. Y. Ni, M. Ji, Y. Z. Tang, X. D. Yang, X. F. Long and S. P. Bi, Electroanal., 2004, 16, 1051 CrossRef CAS.
- K. A. Yao, N. Wa, Y. Q. Shi, H. Y. Ni, Z. B. Yang, C. Sun, Y. Z. Tang and S. P. Bi, Chin. J. Inorg. Chem. (Chinese), 2006, 22, 2202 Search PubMed.
- Y. W. Ma, K. F. Huo, Q. Wu, Y. N. Lu, Y. M. Hu, Z. Hu and Y. Chen, J. Mater. Chem., 2006, 16, 2834 RSC.
-
N. A. Lange, Lange's Chemistry Handbook. 15th ed. McGrawHill: New York, 1999 Search PubMed.
-
Q. S. Yuan, Modern Enzymology, Shanghai: East China University of Science and Technology Press, 2001, pp. 31 Search PubMed.
- C. O. Schmakel, K. S. V. Santhanam and P. J. Elving, J. Am. Chem. Soc., 1975, 97, 5083 CrossRef CAS.
- W. T. Bresnahan and P. J. Elving, J. Am. Chem. Soc., 1981, 103, 2379 CrossRef CAS.
- X. X. Gao and X. Q. Wang, Chin.
J. Anal. Chem. (Chinese), 1998, 26, 757 Search PubMed.
-
(a) K. A. Yao, N. Wang, B. Zhao, J. J. Cheng, H. Y. Ni, Z. B. Yang, C. Sun and S. P. Bi, Chin. J. Inorg. Chem. (Chinese), 2007, 23, 981 Search PubMed;
(b) K. A. Yao, N. Wang, J. Y. Zhuang, Z. B. Yang, H. Y. Ni, Q. Xu, C. Sun and S. P. Bi, Talanta, 2007, 73, 529 CrossRef CAS.
-
(a) P. Vitanov, A. Harizanova, T. Ivanova and T. Dimitrova, Thin Solid Films, 2009, 517, 6327 CrossRef CAS;
(b) J. Schaep, C. Vandecasteele, B. Peeters, J. Luyten, C. Dotremont and D. Roels, J. Membr. Sci., 1999, 163, 229 CrossRef CAS;
(c) H. X. Lu, J. Hu, C. P. Chen, H. W. Sun, X. Hu and D. L. Yang, Ceram. Int., 2005, 31, 481 CrossRef CAS.
- N. Wang, D. Q. Huang, J. Zhang, J. J. Cheng, T. Yu, H. Q. Zhang and S. P. Bi, J. Phys. Chem. C, 2008, 112, 18034 CrossRef CAS.
- Q. Rahman, M. Lohani, E. Dopp, H. Pemsel, L. Jonas, D. G. Weiss and D. Schiffmann, Environ. Health Perspect., 2002, 110, 797 CAS.
- L. C. Renwick, K. Donaldson and A. Clouter, Toxicol. Appl. Pharmacol., 2001, 172, 119 CrossRef CAS.
- H. L. Zhang, Q. Wang, L. P. Song, J. Y. Yue and X. G. Leng, J. Biomedical Eng. (Chinese), 2007, 24, 1295 Search PubMed.
- A. A. Vertegel, R. W. Siegel and J. S. Dordick, Langmuir, 2004, 20, 6800 CrossRef CAS.
- Z. H. Gan, Q. Zhao, Z. N. Gu and Q. K. Zhuang, Anal. Chim. Acta, 2004, 511, 239 CrossRef CAS.
-
(a) H. H. Wang, R. L. Wick and B. S. Xing, Environ. Pollut., 2009, 157, 1171 CrossRef CAS;
(b) M. Green and E. Howman, Chem. Commun., 2005, 121 RSC;
(c) W. Jiang, H. Mashayekhi and B. S. Xing, Environ. Pollut., 2009, 157, 1619 CrossRef CAS;
(d) Y. Y. Guo and X. Q. Zhu, Foreign Medical Sci. (Section of Hygiene), 2008, 35, 268 Search PubMed.
- K. Yang, L. Z. Zhu and B. S. Xing, Environ. Sci. Technol., 2006, 40, 1855 CrossRef CAS.
- R. H. Hurt, M. Monthioux and A. Kane, Carbon, 2006, 44, 1028 CrossRef CAS.
- Z. Q. Qian, K. Siegmann, A. Keller, U. Matter, L. Scherrer and H. C. Siegmann, Atmos. Environ., 2000, 34, 443 CrossRef.
- S. P. Bi, B. Liu, F. R. F. Fan and A. J. Bard, J. Am. Chem. Soc., 2005, 127, 3690 CrossRef CAS.
- R. M. Wightman, Science, 2006, 311, 1570 CrossRef CAS.
Footnote |
† Electronic supplementary information (ESI) available: Investigation of the adsorption of nano-Al2O3 on the HMDE. See DOI: 10.1039/b910557c |
|
This journal is © The Royal Society of Chemistry 2010 |