DOI:
10.1039/B908210G
(Paper)
Analyst, 2010,
135, 62-70
Received
24th April 2009
, Accepted 15th October 2009
First published on
4th November 2009
Abstract
Cell separation and fractionation based on fluorescent and magnetic labeling procedures are common tools in contemporary research. These techniques rely on binding of fluorophores or magnetic particles conjugated to antibodies to target cells. Cell surface marker expression levels within cell populations vary with progression through the cell cycle. In an earlier work we showed the reproducible magnetic fractionation (single pass) of the Jurkat cell line based on the population distribution of CD45 surface marker expression. Here we present a study on magnetic fractionation of a stem and progenitor cell (SPC) population using the established acute myelogenous leukemia cell line KG-1a as a cell model. The cells express a CD34 cell surface marker associated with the hematopoietic progenitor cell activity and the progenitor cell lineage commitment. The CD34 expression level is approximately an order of magnitude lower than that of the CD45 marker, which required further improvements of the magnetic fractionation apparatus. The cells were immunomagnetically labeled using a sandwich of anti-CD34 antibody-phycoerythrin (PE) conjugate and anti-PE magnetic nanobead and fractionated into eight components using a continuous flow dipole magnetophoresis apparatus. The CD34 marker expression distribution between sorted fractions was measured by quantitative PE flow cytometry (using QuantiBRITE™ PE calibration beads), and it was shown to be correlated with the cell magnetophoretic mobility distribution. A flow outlet addressing scheme based on the concept of the transport lamina thickness was used to control cell distribution between the eight outlet ports. The fractional cell distributions showed good agreement with numerical simulations of the fractionation based on the cell magnetophoretic mobility distribution in the unsorted sample.
Introduction
Cell separation is becoming increasingly important for research and clinical applications because of the wealth of molecular data and therapeutic possibilities associated with a defined cell type or even a single cell.1–3 There is a considerable interest in exploring new technologies that go beyond current standards of fluorescent-activated cell sorters (FACS)4 and automated cell counters based on the Coulter principle. The current efforts are mostly focused on adapting microfabrication technology for building cell sorting devices, and on a search for exploitable cell sorting mechanisms. These include miniaturization and modification of the optical detection methods and mechanical actuation methods adapted from larger machines,5 complemented by investigations of other mechanisms that are important only at the microscale, such as dielectrophoresis,6 optical trapping,7 or gelling of heat-sensitive solutions.8 The magnetic separation methods span the range of macro- to micro-separations because of the scaling of magnetic capture cross-section with the size of the magnet and that of the separand.9,10 They are very effective in application to cell separation in microchannels because of the possibility of producing very high field gradients on the microscale.11,12 The operation of popular high-gradient magnetic separation (HGMS) columns is based on properties of stacked, sub-millimeter ferromagnetic microspheres that produce both high local field gradients and highly branched, 3D flow channels.13 Another appealing quality of magnetic forces is that they can provide both sensing and actuation functions, thus significantly simplifying cell sorting mechanics.14 Magnetic cell separation is an active area of research and development because of these qualities and the already well-developed technique of magnetic cell tagging using monoclonal antibodies conjugated to magnetic nanoparticles.15–18
We have set out to determine the current limitations of the resolving power and yield of magnetic cell separation by magnetophoresis in a rectangular, millimeter-scale channel.19 The process relies on an orthogonal superposition of convective and magnetic transport and does not require sensors or actuators. The selectivity of the separation is based on the specificity of the targeting antibody–magnetic nanoparticle conjugate. The throughput is determined by the volumetric flow rate of the cell suspension, but is limited by the field-induced velocity which affects the necessary length of the separation region.20 The potential applications include cell sorting based on the cell surface marker expression, without the complicated fluidics, sensing and actuation mechanics inherent to current optical sorting methods.21 The cell separation relies on a local equilibrium of the magnetic and viscous forces acting on a cell in a flowing suspension which are inherently less well-defined than the discrete stop-and-go algorithms of fluidic switching.22 This introduces a level of uncertainty in the separation that is perhaps less well-controlled than that associated with mechanical switching in microfluidic devices. Fortunately, there is already a large body of data on similar systems such as field-flow fractionation, split-flow thin (SPLITT) channel fractionation, and free-flow cell electrophoresis that can be used for guidance in the experimental design and data analysis.23–28 In particular, we have adapted the concept of a ‘transport lamina’ to control the separation process.20,28,29 For laminar flows, the transport lamina represents a well-defined fraction of flow in the channel across which a cell must be driven to exit at a particular outlet port.27 For multiple outlet ports, the flow in the channel is divided into successive increments to transport lamina defined by virtual stream surfaces that end at stagnation points between neighboring outlet ports.30,31 The transport lamina represents an abstract physical space in the flowing solution that is equivalent to a microfluidic channel except that it is bounded by virtual surfaces within the stream rather than by solid walls.32 This has distinct advantages for cell separation. For example, the cells are not exposed to high viscous shear as they are carried across the transport lamina (the laminar flow profile spans the full breadth of the flow channel).30,31,33 It is also possible to adjust the width of the increments to transport lamina dynamically, that is, without making changes to the physical dimensions of the channel but rather by changing the distribution of the outlet flow rates.29,34 The physical reality of the flow laminae has been beautifully illustrated albeit for patterning,35 mixing,36 and diffusion37 rather than separation purposes.
In the present study we use immunomagnetically labeled KG-1a cells as a hematopoietic progenitor cell surrogate and employ the SPLITT theory as applied to the dipole magnetic flow fractionator (DMF) to control the fluid volume elements that carry the cell fractions to different outlets. By decreasing the increments to transport lamina thickness, simply by reducing the fractional volumetric flow rates at the outlets used for collecting the fractionated sample, a finer fractionation of the sample may be obtained. The increments to transport lamina thickness in each experiment were kept equal for all outlets used for cell fractionation. The effect of the CD34 marker expression on the cell outlet address was measured quantitatively using QuantiBRITE™ fluorescence standards and flow cytometry analysis. The experimental findings based on flow cytometry and cell tracking velocimetry were compared with theoretical predictions based on magnetophoresis.
Materials and methods
Labeling buffer containing 1× phosphate buffered saline (PBS; Central Cell Services (CCS), Lerner Research Institute, Cleveland Clinic, Cleveland, OH), 2 mM ethylenediaminetetraacetic acid (EDTA; 99.4–100.06%, cat. no. E9884; Sigma Aldrich, Inc., St. Louis, MO), and 0.5% Bovine Serum Albumin (BSA; FractionV, approx. 99%; cat. no. A3059; Sigma Aldrich) was prepared at least one day prior the experimental procedures. The labeling buffer was sterile filtered (0.22 μm) and degassed for 30 min with constant stirring under an absolute pressure of 0.16 atm provided by a vacuum pump (model 25458-01, Gardner Denver Thomas, Inc., Welch Vacuum Technology, Niles, IL).
The primitive human acute myelogenous leukemia cell line KG-1a (ATCC Number CCL-246.1™; American Type Culture Collection, Manassas, VA) was used as a model for stem cells due to its high expression of the cell surface marker CD34 and its morphological and cytochemical similarity to primitive hematopoietic cells.38 The cells were cultured according to protocols provided by the ATCC in IMDM (ATCC), supplemented with 10% fetal bovine serum (FBS; CCS), 1% penicillin/streptomycin (50 units/ml/50 μg ml−1; CCS), and 292 μg ml−1L-glutamine (CCS) in 75 cm2 T-flasks (cat. # 430641; Corning Inc., Corning, NY) at 37 °C and 5% CO2 up to a cell density of 1 × 106 cells ml−1.
Cell size distribution and count measurement
The cell samples were evaluated using a Coulter Counter Z2 particle count and size analyzer equipped with a 70 μm aperture (Beckman Coulter, Inc., Fullerton, CA) and gated in a size range from 9 to 17 μm. A minimum of 2000 events were acquired per sample.
For the DMF cell fractionation experiments, cells were removed from the culture flask and washed twice in labeling buffer. After cell number evaluation, 3 × 106 cells were placed in 5 ml Falcon tubes (polystyrene, round-bottom tubes, cat. # 352052; BD Bioscience, Bedford, MA), pelleted by centrifugation (300
g, 4 °C, 5 min), followed by complete removal of the supernatant. Subsequently, the pellet was incubated with the primary antibody, anti-CD34-PE (class III, clone 563; cat. # 550761; BD Pharmingen, San Diego, CA), at a concentration of 20 μl/106 cells for 30 min in the dark and on ice. The sample was then washed once by resuspending in 5 ml of labeling buffer, followed by centrifugation and subsequent complete removal of the supernatant. After adding the secondary antibody, anti-PE MACS beads (cat. # 130-048-801, lot 5080205113; Miltenyi Biotec, Auburn, CA), at a concentration of 20 μl/106 cells, the sample was incubated for 15 min in the dark and on ice. Finally, the sample was washed once more, resuspended in 3 ml of labeling buffer, and after cell number evaluation, diluted to a concentration of 1 × 105 cells ml−1 prior to injection into the DMF.
The CTV principle has been presented before39,40 and a brief summary of the setup is given in the ESI.† The cell samples were labeled prior to fractionation and examined without further modification after the fractionation experiments. The samples were kept pelleted on ice and in the dark after fractionation and before measurement in the CTV apparatus. After completion of the CTV measurements, the samples were pelleted by centrifugation, fixed with 1% v/v of formaldehyde (methanol-free, 16% Ultra Pure; cat. # 18814; Polysciences, Inc., Warrington, PA) in labeling buffer, and subjected to flow cytometry analysis together with a vial of QuantiBRITE™ PE beads (homogenized in labeling buffer prior to flow analysis; BD Pharmingen). Flow cytometry analysis was conducted in the Flow Cytometry Core (Lerner Research Institute, Cleveland Clinic) using a BD LSR I sorter (BD Biosciences, San Jose, CA). At least 2300 events were acquired per outlet sample. The flow cytometry data were subsequently analyzed using WinMDI, a freeware provided by Joe Trotter of the Scripps Institute, La Jolla, CA. Gating of cell and bead populations, as well as estimation of antibodies bound per cell (ABC), was performed according to manufacturer's recommendations.
Dipole magnetic flow fractionator (DMF)
The DMF assembly consisted of a dipole magnet assembled from NdFeB bricks and specially shaped, custom-made pole pieces that minimize edge effects at the entrance and exit points of the magnetic gap. A channel frame incorporated the injector and outlet manifolds and supported the rectangular borosilicate glass channel (interior thickness: 0.75 mm, interior breadth: 8.85 mm, length: 152.4 mm; VitroCom, Mountain Lakes, NJ). The channel frame was designed by one of the authors (S. K.) and manufactured in the Lerner Research Institute Prototype Laboratory. The volumetric flow rates were controlled using syringe pumps for the inlet and outlet flows (Model ‘Pump 33’ and ‘55-4143’, Harvard Apparatus, Holliston, MA; Model ‘74900 series’, Cole-Parmer Instrument Company, Vernon Hills, IL).
The dipole magnetic field was mapped using a Hall-effect Gaussmeter (Model 6010; Probe #STD61-0404-05; F.W. Bell, Orlando, FL). The probe was supported by a linear x–y translation stage (pitch 1/80 inch/revolution; Model 420; Newport Research Corp., Irvine, CA) and moved over quarter-pitch intervals at the magnet half length, z = 0, along the y-direction at the midline of the interpolar gap (at x = 0). A total of 272 data points were acquired over a distance of 22.5 mm. A glass cover slip was used to align the probe with the front surface of the pole pieces. This alignment position was taken to correspond to y = 0 and the initial position for field measurement (see Fig. 1a). The measurements were performed in triplicate. The sample injector tubing (Hypo Tube S7S 316-RW 25 GA, ID 0.01 inch, OD 0.02 inch, length 35 mm, Part # HTXX-25R-12; Small Parts, Inc., Miramar, FL) was connected to a 4-way diagonal flow, 4-port switching valve (V-100D; Upchurch Scientific, Inc., Oak Harbor, WA) to provide a fluidic connection between the sample and a priming fluid without major interruption of the sample flow. The tip of the sample injector tubing was rounded using sand paper and placed into the inlet manifold. All outlets were connected to valves (‘T’-flow path, 3-port Hamilton valves, part # 86727; Alltech Associates, Inc., Deerfield, IL) to facilitate initial system setup, sample syringe collection, and cleaning procedures of the DMF system.
 |
| Fig. 1 (a) Principle of the dipole magnetic fractionator. The feed (cell or particle suspension) is continuously injected into a carrier fluid flow inside a rectangular glass channel. The sample flows downward (z-direction) in the channel and is deflected from its flow path by an interaction with the magnetic field (y-direction) generated by a dipole magnet (not to scale). The sample is fractionated based on the strength of interaction with the magnetic field and collected in 8 different outlet fractions. Also shown are magnetic field (B) lines inside the interpolar gap (simulated with FEMM 4.0.1 freeware57) and the distribution of B and the gradient of B along the y-axis at the mid-plane of the interpolar gap (x = 0). (b) The fractionation of KG-1a cells is controlled by the stream splitting surfaces, demarcated by dashed lines. The stream splitting surfaces indicate parts of the flow that are collected in separate channel outlets (identified by their outlet numbers). The linear extension of these streams (or the transport laminae increments, Δbt) along the magnetic transport direction (y-axis) determine the travel distance required for a cell to reach a particular flow outlet. Note the regular decrease in Δbt between outlets 2 and 6 for different outlet flow distribution setups: Δbt = 400 μm (Setup A), 350 μm (B), 300 μm (C), and 250 μm (D). | |
The channel was positioned precisely inside of the interpolar gap of the magnet using an x–y translation stage (0.49 inch travel; MVN80; Newport Research Corp.) while taking into account the position of the injector with respect to the channel and magnet dimensions. The tip of the injector tubing protruded 10 mm into the channel. The interpolar gap was 2.63 mm at the narrowest point (x-direction, Fig. 1a) and had a maximum magnetic field, B, of 2.2 T (at y = 13.5 mm measured from the pole piece surface). The highest gradient (dB/dy), however, was obtained within 4 mm of the magnetic pole piece surface (0.154 T/mm at y = 3.85 mm, Fig. 1a). Therefore, the channel was placed so that the sample injector was aligned with the magnetic pole piece surface (y = 0, dB/dy = 0.100 T/mm), thus subjecting the migrating species to a steady increase of field with increasing y.
The outlet manifold incorporated 8 outlets, of which outlets 2–8 were connected to syringes by FEP Teflon tubing (ID 1/32′′, OD 1/16′′, length 120 mm; Zeus Industrial Products, Inc., Orangeburg, SC) and a multistage syringe pump. Outlet 1 was left open to the atmosphere to avoid mismatch of internal pressure with the atmosphere that would arise from slight discrepancies between inlet and outlet flow rates. In order to achieve equal, but narrow increments to transport lamina thickness, Δbt, for outlets 2–6, various combinations of common syringes were used (i.e., Becton Dickinson plastic syringes, volumes 3 cm3, 5 cm3, 10 cm3, 20 cm3, and 30 cm3). A detailed schematic and flowchart of the DMF setup is presented in Fig. S1–S3 of the ESI.†
The fractionation principle in the DMF is dependent on a laminar flow pattern inside the channel, an equilibrium of all forces acting on the particles or cells (i.e., magnetic force, drag force, buoyancy force), and the magnetic field geometry. Furthermore, the use of multiple outlet ports and the use of different flow rates at each outlet dictate the positions of the splitting surfaces that separate the fluid into the fractions eluting at different outlets. The positions of the splitting surfaces are determined from ratios of fractional to total flow rate, as discussed earlier.31 An approximate expression for the two-dimensional fluid velocity profile in a thin channel of rectangular cross-section was proposed by Takahashi and Gill.41 Their expression for fluid velocity v may be written in the form |  | (1) |
in which w is the channel thickness, b is the channel breadth, ΔP is the pressure drop along the channel length L, and η is the fluid viscosity. X is the reduced distance from the channel center along the x-axis (X = 2x/w, so that X ranges from −1 to +1 across the channel thickness), Y is the reduced distance from the channel center along the y-axis (Y = 2y/b, and Y ranges from −1 to +1 across the channel breadth). If the splitting surfaces are assumed to be planar and perpendicular to the major channel walls, and a fraction Q(i) of the total channel flow rate Q is bounded by splitting surfaces at Y(i − 1) and Y(i), then |  | (2) |
Solving the integrals, we obtain
|  | (3) |
The position of a splitting surface Y(i) for a given Y(i − 1) and flow rate ratio Q(i)/Q is found by solving eqn (3) with the aid of a Regula-Falsi method using computer software (Maple™, Maplesoft, Waterloo, Ontario, Canada).
The fractional cell content at each specific outlet is determined by steady state magnetophoretic transport orthogonal to the convective transport (Fig. 1a) and the positions of the splitting surfaces across the breadth of the flow channel (Fig. 1b). The magnetophoretic cell transport was unchanged for all experiments as it was fixed by the particular set of the immunomagnetic labeling reagents, the cell labeling protocol (as described above), and the fixed total channel flow rate. The distribution of the splitting surfaces, on the other hand, could be easily changed by changing the outlet flow rate ratios, Q(i)/Q, as shown in eqn (3). Thus, the cell distribution between the flow channel outlets could be varied by varying the widths of transport lamina increments spanning the channel breadth (Fig. 1b). Note the unequal distribution of the stream splitting surfaces. Only the first six outlets (counting from the left) were used for cell fraction collection. The remaining two on the right were used for solution overflow management. The five increments to transport lamina width, corresponding to those outlet streams used for the collection of magnetic cell fractions, were kept to a constant breadth Δbt. This was accomplished by arranging equal flow rates at outlets 2–6. The fraction of the channel breadth occupied by fluid that exited at outlet 1 was much wider than Δbt, and this outlet was used to collect the non-magnetic fraction. This breadth was also kept constant for all experiments. Four different values of Δbt were used, referred to as Setup A (Δbt = 400 μm), Setup B (Δbt = 350 μm), Setup C (Δbt = 300 μm), and Setup D (Δbt = 250 μm), as shown in Fig. 1b (for a detailed summary of the theoretical values of Δbt refer to Table S1 in the ESI†). The breadth of the fluid stream exiting at outlet 1 was 1.9 mm. It contained a streamline originating at the sample injector tip. This particular set of Δbt values and split stream boundaries was selected by matching the volumetric flow rate through the channel, Q, to the cell magnetophoretic mobility distribution measured by CTV, using a computer model of the cell transport within the DMF separator. The model predicts the fractional recovery at outlets 1 through 8, as well as the magnetophoretic mobility distribution within each outlet fraction (Table 1). For a complete summary of the model the reader is referred to the supplemental material. The model was validated by calculating the relative errors in fraction mobilities, defined as the differences between measured and predicted values of the mean cell magnetophoretic mobility for each fraction.
Table 1 Summary of the fractional recovery
Setup |
A |
B |
C |
D |
# cells injected |
413 184 |
475 000 |
465 264 |
518 240 |
Outlet |
Recovery (%) |
1 |
12.3 |
8.4 |
5.6 |
12.3 |
2 |
7.5 |
9.3 |
3.7 |
2.7 |
3 |
8.1 |
8.4 |
3.7 |
2.6 |
4 |
6.5 |
5.0 |
3.3 |
2.0 |
5 |
4.2 |
2.7 |
2.6 |
1.9 |
6 |
1.7 |
3.5 |
1.0 |
0.5 |
7 |
6.6 |
2.3 |
6.4 |
4.3 |
8 |
0.7 |
0.4 |
1.7 |
1.7 |
Total
|
47.6
|
40.1
|
27.9
|
27.8
|
DMF system operation
The flow channel used in this study was characterized by the aspect ratio, b/w = 8.85/0.75 = 11.8, and channel cross-section area S = 6.64 mm2. The ratio of the cell sample feed injection flow rate to the total flow rate was Qf
:
Q = 1
:
10, for the total flow rate of Q = 30 ml h−1. All buffers and solutions were allowed to equilibrate to room temperature. Prior to the fractionation procedure the DMF channel and attached tubing were primed with a detergent solution (1% w/w; Joy Liquid Detergent; Procter & Gamble, Cincinnati, OH) and subsequently primed, bottom up, with degassed labeling buffer. The cell sample was protected from direct light throughout the sorting process to avoid photo-bleaching (this included the labeling, DMF, CTV, and FCM phases). The DMF system operation included an initial period of 10 min to allow for stable flow conditions to develop in the channel prior to sample injection for 80 min. Subsequently, the sample valve was switched to introduce cell-free fluid at the same flow rate, and the remainder of the sample was flushed from the channel over 30 min. After completion of the DMF experiments, the fluid volume in the sample collection syringes was determined by weight, and the cell concentrations determined by Coulter Counter.
Results and discussion
Cell fluorescence intensity and MM distributions in the initial sample
The labeled cell sample was analyzed for cell fluorescence intensity (PE) by flow cytometry and for cell magnetophoretic mobility (MM or m, below) by CTV. The labeled sample consisted of >90% PE-positive, CD34 marker expressing cells and >98% of magnetic cells, as compared to the unlabeled control (Fig. 2a). Note the higher fraction of magnetically labeled cells when compared to fluorescent positive cells. The difference can be attributed to an artifact of the analysis procedure as the CTV measurement was performed before the same sample was subjected to flow cytometry analysis and cell fluorescence losses were expected. The cell PE fluorescence intensity and magnetophoretic mobility showed log-normal distributions, as is typical for an immunocytological labeling technique.42 The magnetic cell fraction was determined as the fraction of cells having mobilities higher than a certain cut-off mobility. This cut-off mobility was defined as the 99-percentile mobility of the unlabeled cell mobility distribution (Fig. 2a).21 Thus, for a given residence time in the magnetic field during separation, the low mobility cells were expected to travel shorter distances across the flow stream lines than the high mobility cells. In particular, the non-magnetic cells would be expected to elute at outlet 1 and the magnetic cells in outlets 2 through 8.
 |
| Fig. 2 (a) Representative distribution of cell magnetophoretic mobility (MM) and cell PE fluorescence intensity (insert). The drop lines indicate cut-off MM (0.1 × 10−4 mm3/TAs) based on the 95th percentile mobility of the unlabeled control sample (the so-called non-magnetic fraction, not shown). The corresponding fraction of magnetically labeled cells was 99%, that of the fluorescently labeled cells was 97.4%. (b) Comparison of fractional recovery in outlets 1–8 for different values of transport lamina (Setup A–D, compare Fig. 1b). Outlets 1 and 7–8 are treated separately because of their different increments to transport lamina thicknesses, Δbt, as shown in Fig. 1b. The lines in the upper portion of the figure show model predicted recovery based on the MM distribution in the unsorted sample (feed, as shown in panel 2a). | |
Cell number distribution in the sorted fractions
The number of cells collected at the eight channel outlets followed the trend as expected from their magnetophoretic mobility distribution (Fig. 2b). The fractional cell recovery distribution followed the cell mobility distribution in a flow-dependent fashion, such that the cell recoveries were generally decreasing with increasing channel outlet number, and that these changes were more pronounced for wide transport lamina increments (Setup A, Δbt = 400 μm) than for narrow increments (for Setup D, Δbt = 250 μm), as shown in Fig. 2b. The wide transport lamina increments capture cells with broader mobility range than do the narrow transport lamina increments, which explains why these changes were more pronounced for Setups A and B than for Setups C and D. One notes that the measured recoveries are lower than the predicted recoveries, and so the sum of the fractional cell recoveries in all outlets is lower than 100%. Furthermore, the total recovery decreases monotonically with decreasing transport lamina increment, Δbt, from 47.6% for Setup A (Δbt = 400 μm), 40.1% for B (Δbt = 350 μm), 27.9% for C (Δbt = 300 μm), and 27.8% for D (Δbt = 250 μm). This is indicative of a systematic effect not predicted by the theory, such as cell loss due to sedimentation and non-specific adhesion in dead flow zones in the channel. Indeed, such effects are expected to be more pronounced for thin transport lamina increments and lower, associated flow rates in the connecting tubing (as in Setup D) than for wide increments (Setup A), as borne out by the experimental data in Fig. 2b. One also notes that the cell content in outlet 1 is higher than the non-magnetic cell fraction determined by the CTV measurement (5–10% as compared to <2% of the non-magnetic fraction; compare Fig. 2b and 2a, respectively). This indicates that a portion of the low-mobility magnetic cell fraction was also captured in this outlet.
Cell fluorescence intensity and MM distributions in the sorted fractions
The number of PE molecules per labeled cell was obtained using QuantiBRITE™ calibration curves (ESI,† Fig. S4). The cell PE intensity in each fraction was measured by flow cytometry. The CD34 expression on the KG-1a cells was higher than that reported by others, also based on QuantiBRITE™ analysis,43 interpreted by us as due to differences in cell culture conditions. A comparison of cell fluorescence intensity between sorted fractions yielded results that are consistent with the magnetophoretic cell transport as a separation mechanism. The mean cell PE fluorescence intensity increased monotonically with the increasing outlet number for the magnetic cell fractions, outlets 2 through 6, and was higher than that in the non-magnetic cell fraction, outlet 1, as expected (Fig. 3a). The highest PE intensity was measured in outlet 7, used for overflow control (insufficient cell numbers were collected at outlet 8 for flow cytometry analysis). This is consistent with the double label binding mechanism for which the presence of a PE moiety on a cell is necessary for magnetic particle binding. Increase in PE molecules bound per cell is therefore correlated with increase in cell magnetization, and hence, magnetophoretic mobility.44 Furthermore, the mean number of PE molecules in the initial cell sample fell between the upper and lower limits of the sorted fractions, as expected (Fig. 3a). We also noted another, more subtle, effect of the transport lamina increment on the PE distribution over the channel outlets, also consistent with the magnetophoretic cell transport mechanism. Specifically, the increase in mean PE molecules bound per cell for a given transport lamina increment with outlet number is steeper for wide transport lamina increments (Setups A and B) than for narrow increments (Setup D), Fig. 3a. As already discussed in the context of the cell number distribution, above, the wide transport lamina increments span a wider fraction of the channel breadth (Fig. 1b) and therefore are expected to collect cells over a wider range of mobility and, consequently, a wider range of cell PE intensity. Thus, although the spatial disposition of the channel outlet ports does not change, the cells are collected from a wider fraction of the flow channel, resulting in a stronger dependence of the mean cell PE on the fraction number than observed for narrow transport lamina increments, Fig. 3a. The effect is weak but points to the flow distribution affecting the resolving power of the cell fractionation (discussed in detail below) and it is consistent with the transport separation mechanism. The mean magnetophoretic mobility for each of the sorted fractions is shown in Fig. 3b. The mean mobilities do not increase with the fraction number as strongly as predicted by theory from the MM distribution in the initial (unsorted) sample (Fig. 3b); however, the differences between them were statistically significant (one-tail Student t-test with unequal sample size and variance, at the 0.05 confidence level, ∞ degrees of freedom, N from 187 to 1673 events, with the fewer events measured in higher outlet number fractions).
 |
| Fig. 3 (a) Mean number of PE molecules per cell for different cell fractions and different transport lamina distribution (Setups A–D). Note the increase in the mean number of PE/cell with increasing outlet number and with increasing Δbt (outlets 2–6). (b) Mean cell MM for different cell fractions and different transport lamina distribution (Setups A–D). The lines show calculated values based on the MM distribution in the unsorted sample in feed (as in Fig. 2a). | |
Correlation between cell fluorescence intensity and magnetophoretic mobility
The correlation between PE intensity and magnetophoretic mobility with increasing cell fraction number was tested directly by measuring the cell mobility for the unsorted sample and each sorted fraction. The measurements were then compared with the corresponding values of the cell PE intensities. The results are presented in terms of mean fractional cell PE intensity and mean fractional mobility normalized to their respective values in the unsorted sample, Fig. 4. The analysis was performed for all four different transport lamina increments (Setups A through D), and all results are included in the figure. The correlation was highly significant, as expected of the transport separation mechanism, (R2 of the linear regression = 0.9218, n = 26).
 |
| Fig. 4 Mean cell PE fluorescence intensity in sorted fraction normalized to that in the unsorted sample graphed against mean cell MM in the sorted fraction normalized to that in the unsorted sample (Setups A–D lumped together). Note the highly significant correlation between cell fluorescence intensity and its magnetophoretic mobility. | |
Relative resolving power of the system
The relative resolving power of a separation system for polydisperse materials may be described in terms of the fractionating power.45 This measure of relative resolution was introduced to describe the quality of separation of polydisperse materials by elution techniques such as field-flow fractionation or size exclusion chromatography. For these applications it was defined as a continuous function of elution time or of the selective property exploited for separation. However, the concept is equally applicable to continuous two-dimensional separations such as multiple-outlet split-flow thin (SPLITT) channel separations,46 two-dimensional field-flow fractionation,47–52 and the magnetic dipole flow sorter under consideration here. In these cases, the fractionating power is defined as a continuous function across the separation dimension, just prior to collection of separated material at discrete outlets. It is apparent that the inherent fractionating power of the separator is realized only for very high numbers of outlets. With the use of a limited number of outlets, there is inevitably a partial remixing of separated material in each outlet stream.
Fractionating power may be defined in terms of any selective property that is exploited for separation. If γ represents the selective property then the γ-based fractionating power is defined as
|  | (4) |
where
Rs is the resolution between species which differ by the small relative increment δ
γ/
γ in the selective property. For elution separations, the resolution is generally defined in terms of elution times:
|  | (5) |
in which δ
tr is the small difference in elution times for the two species, and
σt is the mean standard deviation in their elution times. For displacements along a separation axis, as is the case for the DMF, the resolution is defined in terms of displacement distances:
|  | (6) |
in which δ
y is the small difference in displacement distance along the separation (
y) axis, and
σy is the mean standard deviation in their displacement distances. Substituting
eqn (6) into
eqn (4), and taking mobility
m as the selective property, we obtain
|  | (7) |
in which
Sm is the mobility-based selectivity of the separation defined in terms of relative displacement:
|  | (8) |
It is simple to show that the mobility-based selectivity must be equal to unity. It follows that
|  | (9) |
The major contributions to
σy will arise out of the finite breadth of the feed stream into the system, and the variation of longitudinal fluid velocity across the separation
channel thickness leading to variation in residence time in the separation zone. These are independent contributions to band spreading and will add in terms of their contributions to variance. If the feed stream is relatively narrow, we might expect
σy to increase almost linearly with
y, in which case
Fm would be expected to be nearly constant across the
channel breadth. For a relatively wide feed stream we would expect
Fm to be relatively low at small
y but to increase across the
channel breadth. If it is assumed that the feed stream occupies an initial breadth of
bf then the equation for
Fm could be written in the form
|  | (10) |
in which it is assumed that the standard deviation in migration distance caused by the variation in residence time is some monotonic, increasing function
f of the migration distance,
y −
bf/2.
For collection of fractionated material over a breadth interval of bc, an additional contribution to the variance is made, so that
| 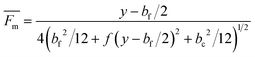 | (11) |
Note that

does not represent a true fractionating power, and we have included the bar to indicate this.

simply represents the ratio of
y/4
σy for the outlet stream. If it is now assumed that
σy ≪
y, then it follows from the unit selectivity that
|  | (12) |
Although this assumption must be considered to be approximate, it is useful as an estimate of the mobility distribution in the outlet collection stream. We can go further, and assume that the breadths of various fluid streams are proportional to their flow rates in the thin
channel, so that
|  | (13) |
in which
Qt is a
transport stream between the feed and the collection streams. This allows the estimation of resolution between neighboring outlet stream compositions. If we assume that neighboring outlet stream flow rates are equal, then
|  | (14) |
in which

represents the mean standard deviation in mobilities.
Eqns (10),
(11),
(13), and
(14) include the unknown function
f, but we can still draw some qualitative conclusions from these equations regarding separation performance.
For each of the experimental setups considered here, the Qc values are constant for outlets 2–6, so that Qt = 0, Qc, 2Qc, 3Qc, and 4Qc for the successive outlets. Eqn (14) indicates that for a given setup, Rs should decrease across outlet pairs 2–3, 3–4, 4–5, and 5–6. Also, as Qc is reduced (considering the order of Setups A, B, C, to D) with a finite feed flow rate Qf, eqn (14) predicts decreasing resolutions for neighboring outlets.
The resolution between outlets is expected to decrease with decreasing Δbt. This is confirmed by the numerical fractionation simulation based on the fractional mean magnetophoretic mobilities and the respective standard deviations. The mean resolution for outlets 2–6 is decreasing from 0.163 for Setup A, 0.161 for Setup B, 0.139 for Setup C, to 0.111 for Setup D. The individual resolutions between neighboring outlets are given in Tables S2 and S3 of the ESI.† The evaluation of fractionation power based on measured CTV values showed much lower values. Note here, that the CTV values were gated for label+ cells that have a mobility ≥1 × 10−5 mm2/TAs. The mean resolution between outlets 2–5 for Setups A, C, and D is 0.04, 0.05, and 0.05, respectively. The mean resolution for Setup B is evaluated only for outlets 2–4 and results in 0.16. The mean resolution values based on fluorescence intensity (geometric mean) for Setups A, B, C, and D are 0.48, 0.89, 0.56, and 0.41, respectively and support the theoretical trend.
Conclusions
A state-of-the-art magnetophoretic fractionation system was described and used to explore new territory in the field of magnetic cell separation. Separation is achieved according to the degree of cell magnetization rather than into two fractions of the ‘magnetic’ and ‘non-magnetic’ sub-populations that is the current laboratory practice. The system consisted of a precision glass, rectangular, high-aspect-ratio flow channel with a special-purpose, multi-outlet flow manifold and system of precision pumps controlling inlet flows and the flow distribution between the outlet ports. A specially-designed permanent magnet generating a magnetic force field directed orthogonal to the flow was used. The pole pieces were specially shaped so that a nearly constant magnetic force field was generated across the breadth of the flow channel (a Faraday field that is locally nearly isodynamic) ensuring a position-independent magnetic cell velocity component. The system, dubbed the dipole magnetic flow fractionator (DMF), was tested on a human cell line (KG-1a) expressing a cell surface marker characteristic of hematopoietic progenitor cells (CD34), labeled with a fluorescence-conjugated targeting antibody and a magnetic colloid. Commercially available reagents and cell media were used for compatibility with the quantitative flow cytometry, used as a reference.
Earlier DMF prototypes were tested for fractionation of immunomagnetically labeled, peripheral blood T-lymphocytes and an immortalized T cell line (Jurkat).30,31,53 Compared to T-lymphocytes, the fractionation of the KG-1a cell line presents a more challenging model because of the lower expression of the CD34 cell surface antigen on KG-1a cells as compared to the CD45 antigen expression of the T-lymphocytes.54–56 This results in lower KG-1a cell magnetophoretic mobilities.21 Consequently, for the given flow rates the magnetic deflection of a KG-1a cell was lower (at most 2 mm) than that of Jurkat cells (up to 4 mm). Accordingly, the DMF system used in this study was redesigned and rebuilt to allow the use of a thinner precision glass channel and smaller inlet and outlet flow manifolds. The reduced interpolar gap allowed application of a higher magnetic field gradient and magnetic force on the KG-1a cells.
In designing and analyzing the performance of the magnetophoretic sorter system, we were aided by the theory of split-flow thin (SPLITT) fractionation. In particular, we have based the flow distribution patterns on the concept of splitting surfaces that determine which portion of the flow in the channel is assigned to a given outlet port. The increments to transport lamina thickness functioned effectively as microchannels collecting fractions of cells differing in magnetophoretic mobility. By manipulating the increments to transport lamina thickness, the fractionated cells were collected from different regions of the channel breadth. The influence of this on the resolving power of the system was examined.
The precise control over the fluidics and magnetic force components acting on the cell allowed us to develop a quantitative description of the magnetic fractionator process typical of quantitative separation science dealing with polydisperse suspensions (such as chromatography). In particular, parameters such as the resolving power will be useful for quantitative comparison between different targeting antibodies, magnetic nanoparticles, microfluidic channel geometries, and cell types likely to be encountered in future applications. The chosen cell model system is pertinent to applications of (hematopoietic) progenitor cells to cellular therapy and, potentially, tissue engineering. Advances in magnetic cell sorting and fractionating devices are supported by the ongoing development of better quality, cheaper microfluidics systems, stronger permanent magnet materials, and improved nanoparticle synthesis and characterization.
Acknowledgements
Funding support was provided by the NIH (CA62349) and the NSF (BES-0124897). We thankfully acknowledge the help of data acquisition by the Flow Cytometry Core of the Cleveland Clinic, Lerner Research Institute.
References
- S. Nagrath, L. V. Sequist, S. Maheswaran, D. W. Bell, D. Irimia, L. Ulkus, M. R. Smith, E. L. Kwak, S. Digumarthy, A. Muzikansky, P. Ryan, U. J. Balis, R. G. Tompkins, D. A. Haber and M. Toner, Nature, 2007, 450, 1235–1239 CrossRef CAS
.
- D. T. Scadden, Nature, 2006, 441, 1075–1079 CrossRef CAS
.
- T. Lapidot, C. Sirard, J. Vormoor, B. Murdoch, T. Hoang, J. Caceres-Cortes, M. Minden, B. Paterson, M. A. Caligiuri and J. E. Dick, Nature, 1994, 367, 645–648 CrossRef CAS
.
- L. A. Herzenberg, D. Parks, B. Sahaf, O. Perez, M. Roederer and L. A. A. Herzenberg, Clin. Chem., 2002, 48, 1819–1827 CAS
.
- A. Y. Fu, H. P. Chou, C. Spence, F. H. Arnold and S. R. Quake, Anal. Chem., 2002, 74, 2451–2457 CrossRef CAS
.
- J. Vykoukal, D. M. Vykoukal, S. Freyberg, E. U. Alt and P. R. C. Gascoyne, Lab Chip, 2008, 8, 1386–1393 RSC
.
- T. D. Perroud, J. N. Kaiser, J. C. Sy, T. W. Lane, C. S. Branda, A. K. Singh and K. D. Patel, Anal. Chem., 2008, 80, 6365–6372 CrossRef CAS
.
- Y. Shirasaki, J. Tanaka, H. Makazu, K. Tashiro, S. Shoji, S. Tsukita and T. Funatsu, Anal. Chem., 2006, 78, 695–701 CrossRef CAS
.
-
U. Hafeli, in Scientific and Clinical Applications of Magnetic Carriers, ed. U. Hafeli, W. Schuett, J. Teller and M. Zborowski, Plenum Press, New York, 1997, pp. 1–10 Search PubMed
.
-
E. P. Furlani, Permanent Magnet and Electromechanical Devices, Academic Press, San Diego, CA, 2001 Search PubMed
.
- C. H. Ahn, M. G. Allen, W. Trimmer, Y.-N. Jun and S. Erramilli, J. Microelectromech. Syst., 1996, 5, 151–157 CrossRef CAS
.
- A. R. Urbach, J. C. Love, M. G. Prentiss and G. M. Whitesides, J. Am. Chem. Soc., 2003, 125, 12704–12705 CrossRef CAS
.
-
A. B. Kantor, I. Gibbons, S. Miltenyi and J. Schmitz, in Cell Separation Methods and Applications, ed. D. Recktenwald and A. Radbruch, Marcel Dekker, Inc., New York, 1998, pp. 153–173 Search PubMed
.
- M. A. M. Gijs, Microfluid. Nanofluid., 2004, 1, 22–40 CAS
.
- N. Pamme, Lab Chip, 2006, 6, 24–38 RSC
.
- Q. A. Pankhurst, J. Connolly, S. K. Jones and J. Dobson, J. Phys. D: Appl. Phys., 2003, 36, R167–R181 CrossRef CAS
.
- Q. Ramadan, V. D. Samper, D. P. Puiu and C. Yu, J. Microelectromech. Syst., 2006, 15, 624–638 CrossRef CAS
.
- B. B. Yellen, R. M. Er, H. S. So, J. R. Hewlin, H. Shan and G. U. Le, Lab Chip, 2007, 7, 1681–1688 RSC
.
- M. Zborowski, L. R. Moore, P. S. Williams and J. J. Chalmers, Sep. Sci. Technol., 2002, 37, 3611–3633 CAS
.
- P. S. Williams, M. Zborowski and J. J. Chalmers, Anal. Chem., 1999, 71, 3799–3807 CrossRef CAS
.
- Y. Jing, L. R. Moore, P. S. Williams, J. J. Chalmers, S. S. Farag, B. Bolwell and M. Zborowski, Biotechnol. Bioeng., 2007, 96, 1139–1154 CrossRef CAS
.
- A. Wolff, I. R. Perch-Nielsen, U. D. Larsen, P. Friis, G. Goranovic, C. R. Poulsen, J. P. Kutter and P. Telleman, Lab Chip, 2003, 3, 22–27 RSC
.
- J. C. Giddings, Sep. Sci. Technol., 1992, 27, 1489–1504 CrossRef CAS
.
- R. Hartig, M. Hausmann, J. Schmitt, D. B. Herrmann, M. Riedmiller and C. Cremer, Electrophoresis, 1992, 13, 674–676 CrossRef CAS
.
- C. Contado, F. Dondi, R. Beckett and J. C. Giddings, Anal. Chim. Acta, 1997, 345, 99–110 CrossRef CAS
.
- C. B. Fuh, E. M. Trujillo and J. C. Giddings, Sep. Sci. Technol., 1995, 30, 3861–3876 CrossRef CAS
.
- M. Hoyos, L. R. Moore, K. E. McCloskey, S. Margel, M. Zuberi, J. J. Chalmers and M. Zborowski, J. Chromatogr., A, 2000, 903, 99–116 CrossRef CAS
.
- P. S. Williams, M. Hoyos, P. Kurowski, D. Salhi, L. R. Moore and M. Zborowski, Anal. Chem., 2008, 80, 7105–7115 CrossRef CAS
.
- P. S. Williams, K. Decker, M. Nakamura, J. J. Chalmers, L. R. Moore and M. Zborowski, Anal. Chem., 2003, 75, 6687–6695 CrossRef CAS
.
- L. R. Moore, M. Zborowski, L. Sun and J. J. J. Chalmers, J. Biochem. Biophys. Methods, 1998, 37, 11–33 CrossRef CAS
.
- T. Schneider, L. R. Moore, Y. Jing, S.-J. Haam, P. S. Williams, A. J. Fleischman, S. Roy, J. J. Chalmers and M. Zborowski, J. Biochem. Biophys. Methods, 2006, 68, 1–21 CrossRef CAS
.
- N. Callens, M. Hoyos, P. Kurowski and C. S. Iorio, Anal. Chem., 2008, 80, 4866–4875 CrossRef CAS
.
- M. Mollet, R. Godoy-Silva, C. Berdugo and J. J. Chalmers, Biotechnol. Bioeng., 2007, 100, 260–272
.
- P. S. Williams, L. R. Moore, J. J. Chalmers and M. Zborowski, Anal. Chem., 2003, 75, 1365–1373 CrossRef CAS
.
- P. J. Kenis, R. F. Ismagilov and G. M. Whitesides, Science, 1999, 285, 83–85 CrossRef CAS
.
- B. Stoeber, D. Liepmann and S. J. Muller, Phys. Rev. E: Stat., Nonlinear, Soft Matter Phys., 2007, 75, 066314 CrossRef
.
- A. Hatch, A. E. Kamholz, K. R. Hawkins, M. S. Munson, E. A. Schilling, B. H. Weigl and P. Yager, Nat. Biotechnol., 2001, 19, 461–465 CrossRef CAS
.
- H. P. Koeffler, R. Billing, A. J. Lusis, R. Sparkes and D. W. Golde, Blood, 1980, 56, 265–273 CAS
.
- L. R. Moore, M. Zborowski, M. Nakamura, K. McCloskey, S. Gura, M. Zuberi, S. Margel and J. J. Chalmers, J. Biochem. Biophys. Methods, 2000, 44, 115–130 CrossRef CAS
.
- M. Nakamura, M. Zborowski, L. C. Lasky, S. Margel and J. J. Chalmers, Exp. Fluids, 2001, 30, 371–380 CrossRef
.
- T. Takahashi and W. N. Gill, Chem. Eng. Commun., 1980, 5, 367–385 CrossRef CAS
.
-
H. M. Shapiro, Practical Flow Cytometry, John Wiley & Sons, Inc., Hoboken, NJ, 4th edn, 2003 Search PubMed
.
-
R. Xu, K. Deluca and A. Liebmann-Vinson, Eur. Pat., 1881063, 2008 Search PubMed
.
- D. R. Leigh, S. Steinert, L. R. Moore, J. J. Chalmers and M. Zborowski, Cytometry, Part A, 2005, 66a, 103–108 CrossRef CAS
.
- J. C. Giddings, P. S. Williams and R. Beckett, Anal. Chem., 1987, 59, 28–37 CrossRef CAS
.
- J. C. Giddings, Sep. Sci. Technol., 1985, 20, 749–768 CrossRef CAS
.
- M. N. Myers and J. C. Giddings, Powder Technol., 1979, 23, 15–20 CrossRef CAS
.
- J. C. Giddings, J. Chromatogr., A, 1990, 504, 247–258 CrossRef CAS
.
- M. R. Schure, M. N. Myers, K. D. Caldwell, C. Byron, K. P. Chan and J. C. Giddings, Environ. Sci. Technol., 1985, 19, 686–689 CAS
.
- P. Vastamäki, M. Jussila and M.-L. Riekkola, Sep. Sci. Technol., 2001, 36, 2535–2545 CrossRef CAS
.
- P. Vastamäki, M. Jussila and M.-L. Riekkola, Analyst, 2003, 128, 1243–1248 RSC
.
- P. Vastamäki, M. Jussila and M.-L. Riekkola, Analyst, 2005, 130, 427–432 RSC
.
- M. Zborowski, L. R. Moore, S. Reddy, G. H. Chen, L. Sun and J. J. Chalmers, Asaio J., 1996, 42, M666–671 CAS
.
- A. Hendrickx and X. Bossuyt, Cytometry, 2001, 46, 336–339 CrossRef CAS
.
- M. J. Borowitz, J. Shuster, A. J. Carroll, M. Nash, A. T. Look, B. Camitta, D. Mahoney, S. J. Lauer and D. J. Pullen, Blood, 1997, 89, 3960–3966 CAS
.
- F. Lanza, S. Moretti, B. Castagnari, A. Latorraca, G. M. Rigolin, A. Bardi and G. Castoldi, Leuk. Lymphoma, 1995, 18(Suppl. 1), 25–30 CrossRef
.
-
D. C. Meeker, Finite Element Method Magnetics, Version 4.0.1 ( 03Dec2006 Build), http://femm.foster-miller.net Search PubMed
.
Footnotes |
† Electronic supplementary information (ESI) available: CTV principle details, predicting recoveries and outlet compositions from the equations of motion, schematic and flowchart of the DMF setup, data acquisition flowchart, detailed summary of the theoretical values of Δbt and individual resolutions between neighboring outlets. See DOI: 10.1039/b908210g |
‡ Current address: Department of Chemistry, University of Washington, Seattle, Washington 98195, USA. |
§ Current address: School of Physics, The University of Western Australia, Crawley, Perth, WA 6009, Australia. |
|
This journal is © The Royal Society of Chemistry 2010 |
Click here to see how this site uses Cookies. View our privacy policy here.