DOI:
10.1039/B905145G
(Paper)
Metallomics, 2009,
1, 479-488
Incorporation of excess gadolinium into human bone from medical contrast agents
Received
13th March 2009
, Accepted 25th August 2009
First published on 16th September 2009
Abstract
We find anomalously high gadolinium (Gd) concentrations in the femoral head bones of patients exposed to chelated Gd, commonly used as a contrast agent for medical imaging. Gd is introduced in chelated form to protect patients from exposure to toxic free Gd3+, a calcium antagonist which disrupts cellular processes. Recent studies suggest Gd chelates break down in vivo, and Gd accumulation in tissue is linked to medical conditions such as nephrogenic systemic fibrosis (NSF), acute kidney failure, and in some cases death. We measure Gd and other rare earth element (REE) concentrations in 35 femoral heads by solution based ICP-MS. Gd concentrations in patients with documented exposure to Gd-based contrast agents (n = 13: Gd DTPA-BMA (Omniscan) n = 6; Gd HP-DO3A (Prohance) n = 5; unknown type n = 4) are significantly higher (p < 0.001) than the control group (n = 17). We use our control group to establish the ‘natural’ background level of Gd in human bone (cortical 95% CI: 0.023, 0.041 nmol/g; trabecular 95% CI: 0.054, 0.107 nmol/g). A control group outlier reveals the occurrence of individuals with high concentrations of all REEs, including Gd. Because of this, we calculate Gd anomalies from the concentrations of adjacent REEs and normalize to the control group mean to isolate Gd input from contrast agents. Normalized Gd anomalies, (Gd/Gd*)N, for exposed patients range up to >800 times the ‘natural’ level (95% CI: 124, 460). Our data confirm that Gd, introduced in chelated form, incorporates into bone and is retained for more than 8 years. No difference was observed in bone Gd concentrations and anomalies between patients dosed with Gd DTPA-BMA (Omniscan; n = 6) and Gd HP-DO3A (Prohance; n = 5). Osteoporotic fracture patients exposed to Gd have significantly lower Gd concentrations than osteoarthritis patients (p < 0.001). This indicates different mechanisms of metal incorporation and/or retention in osteoporotic bone tissues, and may signal an increased risk of endogenous Gd release for patients with increased rates of bone resorption (e.g. osteoporosis patients and menopausal, pregnant, and lactating women) who are exposed to Gd-based contrast agents.
Introduction
The use of contrast agents has expanded the role of imaging in medical diagnostic studies,1 and hence the size of the population exposed to chelated Gd. In fact, since 2005 more than 20 million Gd-based contrast enhancement procedures are performed each year.2 Gd (z = 64) is a paramagnetic rare earth element (REE) commonly used as a contrast enhancement agent for clinical and diagnostic medical imaging.3–5 Gd was the first contrast agent introduced for diagnostic MRI studies because of its paramagnetic nature with an efficient (long) T1 relaxing mechanism.6 Free gadolinium (Gd3+) is extremely toxic,7,8 disrupts cellular processes,9,10 inhibits stretch-activated ion channels,11 and is one of the most efficient known calcium antagonists.9,12,13 Chelation of Gd in the MRI contrast media is intended to prevent toxic cationic Gd (Gd3+) from directly interacting with cells and tissues, and to allow efficient and rapid removal of the contrast agent from the body following imaging studies.6,14,15 Severe adverse health effects may occur with the use of Gd-based contrast agents if Gd dissociates from chelate structures in the blood stream. Gd3+ release from the chelating agent is known to occur via transmetallation reactions in which metals in blood and extracellular fluids (e.g. Cu, Zn, Fe, Ca) replace Gd within the chelate structure.2,16–18 Anions such as PO42−; CO32−; OH− compete with the chelates for the Gd cation.2,11 Transmetallation reactions also release Gd3+ from Gd DTPA-BMA during transport across the cellular membrane, concentrating ionic Gd3+ within cells.19
Previous studies have shown that excretion of the majority of Gd-chelates occurs within 90 minutes of injection, from which the authors concluded that Gd is rapidly removed from the body.20–22 Other studies document toxicity resulting from exposure to Gd-based contrast agents, linking them to symptoms such as nephrogenic systemic fibrosis (NSF),1,17,23,24 anaphylactic shock,25 acute kidney failure,21,26 and in some cases death in patients who had previously compromised kidney and immune function.27 These studies indicate that some of the Gd chelate agent may degrade quickly after injection, allowing release and incorporation of free Gd3+ into the body, e.g. into the skin tissue of nephrogenic systemic fibrosis patients.28–30 Gadolinium concentrations averaging 2.8 and 7.5 nmol/g were incorporated into bone tissues of patients exposed to Gd DTPA-BMA (Omniscan) and Gd HP-DO3A (Prohance) three days prior to hip replacement surgery.31
Human bone mineral (a carbonated hydroxyapatite) serves as a reservoir for the majority of the body’s Ca (99%), P (80%), Mg (50%), and other trace metals.32–34 Metals incorporate into bone by two major routes: (1) “active” incorporation during osteoblast-mediated bone mineralization, and (2) “passive” ionic exchange into the crystal lattice of individual bone mineral crystals (2–3 nm × 20–40 nm platelets).35–37 The [calcium] × [phosphate] ion product in extracellular fluid is supersaturated with respect to bone mineral.33 During “active” incorporation, osteoblastic membrane transport from the extracellular fluid regulates the delivery of these ions to the mineralizing collagen-rich organic matrix of developing bone, along with lesser amounts of other metal cations present in the fluid (Mg, Sr, Pb, Gd, other REE, etc.). Ionic exchange with the bone mineral lattice is dependent on the relative concentrations of trace metal cations, ionic fit in lattice vacancies, and individual solubility products of their phosphate and carbonate salts.11 Metals are also released from bone during bone resorption. Multinucleated osteoclasts secrete HCl into membrane-sealed resorption pits on the bone surface, lowering the pH to about 4 and completely dissolving the bone mineral under these specialized cells. This maintains calcium homeostasis in the blood, as well as removing bone targeted for replacement during normal remodeling.33,34,38,39 Bone turnover in normal adults is in the range of 5–15% of total bone mass per year, but rates for resorption of the trabecular (spongy) bone in postmenopausal women are 19–26%.40 Therefore, Gd incorporated into bone, whether as free Gd3+ or as a chelate, may potentially be endogenously released back into the bloodstream by bone resorption and remodeling processes, as has been shown for other trace metals.34 For example, stable isotopic compositions of blood Pb have shown that up to 70% of plasma Pb is released endogenously from bone tissue,41 with increased plasma Pb concentrations in pregnant and lactating women, who experience increased bone resorption.42,43
We investigate the relation between Gd concentrations in trabecular and cortical bone tissues and medical exposure to Gd chelate contrast agents. Femoral heads removed during hip replacement surgeries are analyzed for Gd and other REEs to identify anomalous Gd concentrations. The effects of bone pathology on metal incorporation and retention are investigated by comparing Gd concentration in the bone of osteoporotic fracture patients to those of osteoarthritis patients.
Experimental
Sample selection
Bone samples consist of femoral heads obtained from patients who underwent total hip replacement surgery at the University of Rochester Medical Center, prior to specimen disposal (RSRB approval #0019010). Clinical histories associated with the samples are limited to the occurrence or lack of occurrence of Gd-based contrast agent exposure, some brand information, the reason for hip replacement surgery, and routine personal data, e.g. gender, age. The control group includes 18 osteoarthritis patients with no exposure to Gd-based contrast imaging studies. The Gd exposure group includes 13 patients with medical records documenting exposure to Gd based contrast imaging studies, with maximum time elapsed since exposure of 8 years prior to surgery. Contrast agent type is known for 11 of these patients: the linear, non-ionic, Gd-chelate2 Gd DTPA-BMA (Omniscan), n = 6; and the macrocyclic non-ionic, Gd-chelate2 Gd HP-DO3A (Prohance), n = 5.
Bone samples from 4 additional patients have anomalous Gd concentrations but incomplete associated medical records with no Gd exposure histories. These 4 patients are not included in comparisons of control and exposure group. Based on our inference that they have been exposed to Gd contrast agents (see results), we include these 4 patients in our comparison of osteoarthritis and fracture patients exposed to Gd. Table 1 summarizes the groups.
Table 1 Patient groups according to medical histories of exposure to Gd based contrast agents. OA = osteoarthritis; F = fracture. The absence of fracture patients (F) in the control group reflects the difficulty of retrieving complete medical records for emergency surgery patients
Control group |
Gd exposure group |
Incomplete medical histories |
n = 18 |
n = 13 |
n = 4 |
OA = 18 |
OA = 7 |
OA = 2 |
F = 0 |
F = 6 |
F = 2 |
Sample preparation
Sample preparation was conducted in a class 100 trace metal free clean laboratory facility. Femoral heads were cross-sectioned to a thickness of approximately 10 mm using a low speed diamond tip Isomet saw. Samples were dried for 48 hours (or until dry) at 80 °C in a vacuum oven. Trabecular and cortical bone samples were extracted using a manually operated precision Dremel tool with graphite carbide tip to provide approximately 300 mg of each bone tissue type per patient. Care was taken to avoid anomalous sections of bone, such as areas near fracture zones, areas of necrosis, zones of hemorrhage, or arthritic nodules. Fifty milligram (50 mg) aliquots of cortical and trabecular tissues from each patient were dried and then crushed with a ceramic mortar and pestle. After crushing, sample weights were recorded to ±0.001 mg, and dried bone material was digested in Teflon vessels using concentrated (15.9 mol L−1) ultra-pure nitric acid (HNO3) at 90 °C for approximately 5 hours or until digestion was complete. The remaining solution was baked for approximately 3 hours (or until dry) at 90 °C to remove excess nitric acid. Residual dried material was then redigested with ultra pure HNO3. Internal standards consisting of known quantities of indium (In) and bismuth (Bi), used to correct for instrumental drift, were added to the digested bone material. The solutions were then diluted using water purified to 18.2 MΩ cm resistance (by a Milli-Q water purification system, Millipore, Bedford, Mass., USA) to result in a final concentration of 2% nitric acid (by volume), and 1 ng/g of each of the internal standards (In and Bi). All standards used during ICP-MS analysis, including aliquots of the certified NIST 1486 SRM and procedural blanks, were prepared in an analogous fashion.
Quantification of NIST 1486 SRM bone powder
Accurate determination of trace element concentrations by ICP-MS requires the use of a directly matrix-matched standard,44 with a similar major chemical composition and mineralogical form to the sample. Although the National Institute for Standards and Technology (NIST, USA) does currently certify two modern bone standard reference materials (SRM’s), NIST SRM 1400 and NIST SRM 1486, for specific major and minor elements, no current standard allows for the quantification of many biologically relevant trace and rare earth elements, including those of interest in this study (Sm, Gd, Tb).
We use standard addition to quantify Sm, Gd, and Tb concentrations in NIST 1486 SRM, which consists of powdered bone meal that approximates the matrix of the sample unknowns. Standard addition quantification eliminates potential effects of variable dilution of the matrix and any associated matrix matching effects. The standard addition procedures used are as follows:
(1) Aliquots of NIST 1486 SRM were prepared using preparation techniques identical to those used for bone samples.
(2) Known quantities of pure element standard solution were added to six aliquots, while one was left with unaltered NIST 1486 SRM to produce an array of samples with final concentrations of 0, 0.1, 0.5, 1, 5, and 10 ng/g for each analyte, with duplicate aliquots at 0.1 ng/g concentration.
(3) Each sample, with a known concentration of NIST 1486 SRM material and known quantities of added element standard, was then analyzed by ICP-MS, giving the intensity of each analyte in counts per second. Five replicate analyses of each sample were performed and values averaged to result in a mean intensity for each analyte.
(4) For each element, mean intensity (counts per second) of each sample was plotted against the amount of known standard added. The data were then regressed to determine the initial concentration of each analyte in NIST 1486 SRM. The regression for Gd is shown as an example in Fig. 1.
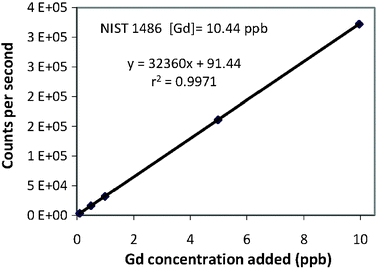 |
| Fig. 1 Standard addition linear regression for Gd concentration in NIST 1486 SRM. | |
Table 2 shows the calculated concentrations of Pb, Sm, Gd, and Tb in bone powder (NIST 1486 SRM), as well as the previously certified concentration of Pb. Results obtained by this study for Pb are in agreement with the certified value (<1σ) as reported by NIST, USA.45
Table 2 Concentrations of Sm, Gd, and Tb in bone powder (NIST 1486 SRM) as determined by standard addition, and comparison of calculated Pb with previously certified Pb value.45 Note that the Pb concentration determined in this study by standard addition is <1σ from the certified value
(a) Calculated element concentrations (this study) |
Element |
Pb (ng/g) |
Sm (ng/g) |
Gd (ng/g) |
Tb (ng/g) |
Conc. |
1329 |
10.57 |
10.44 |
6.12 |
St. dev. |
12.2 |
0.11 |
0.11 |
0.07 |
(b) Previously certified Pb concentration (NIST, 2007) |
Element |
Pb (ng/g) |
|
|
|
Conc. |
1335 |
|
|
|
St. dev. |
14 |
|
|
|
Analytical procedures and uncertainty
REE concentrations were measured using a Thermo X7 ICP-MS. Solution based ICP-MS analyses were conducted with modifications to the EPA 6020A methodologies,46,47 the approved ICP-MS analytical procedure for inorganic trace elements in soils and sediments. To clean sample lines and reduce memory effects, sample lines were washed with 2% nitric acid solution for 120 seconds between analyses. Procedural blanks were analyzed within each block of 8 samples, to monitor and correct for instrumental and procedural backgrounds.
Calibration standards used to determine REE concentrations in bone samples included aliquots of NIST 1486 SRM spiked with known quantities of REEs in a linear range from 0.1 to 10 ng/g. Standards were prepared from 1000 mg/L single element standards obtained from SCP Science, USA. Concentrations of REEs in NIST 1486 were quantified by standard addition as described above. Unaltered aliquots of NIST 1486 SRM were analyzed as unknowns to determine external precision as less than 5%. Isobaric corrections were performed on-line using ICP-MS software. Three duplicate analyses (n = 3) were performed for all analytes for each sample solution. Limits of detection (LOD) according to Long and Winefordner48 are as follows: Gd = 0.000158 nmol/g; Sm = 0.0000124 nmol/g; Tb = 0.000554 nmol/g. Limits of quantification according to Long and Winefordner48 are as follows: Gd = 0.000189 nmol/g; Sm = 0.000022 nmol/g; Tb = 0.000556 nmol/g. Method detection limits were calculated according to the two-step approach using the t99SLLMV method49 at 99% CI (t = 3.71) as Gd = 0.00236 nmol/g, Sm = 0.00458 nmol/g, Tb = 0.00100 nmol/g. MDL values incorporate matrix effects and interferences associated with the complex bone matrix, and provide a more robust and accurate limit of detection for these analyses.
Calculation of Gd anomaly
We calculate the amount of excess Gd incorporated into bone from medical exposure as a Gd anomaly (eqn (1); Table 3), which is defined as the ratio of the measured Gd concentration in cortical bone tissue samples relative to the concentration expected based on the concentrations of adjacent REEs. Gd/Gd* compares measured Gd in bone to the expected value Gd* for normal dietary and environmental inputs based on other REEs, similar to the method used for rocks, soils and water.50 Because the rare earth element group (REEs) behaves in a coherent fashion, relative abundances remain nearly constant in natural systems (e.g. rocks, soils, water). The constants in eqn (1) normalize elemental abundance to upper continental crust (UCC) (i.e. most natural soils). | Gd anomaly = Gd/Gd* = [Gd] M/[0.33[Sm] M + 0.67[Tb] M] | (1) |
Mean Gd anomaly for the control group is 1.45, similar to that observed for NIST 1486 SRM (Gd/Gd* = 1.38). This indicates that the concentration of Gd relative to Sm and Tb in normal human bone is almost 1.5 times higher than its relative concentration in upper continental crust. Exposure group Gd anomalies were divided by 1.45 to estimate excess Gd exposure beyond natural environmental inputs. Eqn (2) isolates the amount of Gd in bone tissue that results from medical dosing of contrast agents. A (Gd/Gd*)N value of 1 reflects observed ‘normal’ Gd incorporation. | Normalized Gd anomaly = (Gd/Gd*)N = (Gd/Gd*)/1.45 | (2) |
Results
Gd concentrations (nmol/g) in trabecular and cortical bone tissues of the control group and exposure group are shown in Table 3, along with statistical summaries. The amount of excess Gd incorporated into cortical bone from medical exposure was estimated by calculating the Gd anomaly (Gd/Gd*) (eqn (1); Table 3), and normalizing to the control group mean (Gd/Gd*)N (eqn (2); Table 3).
Table 3 Gd concentrations and anomalies for (a) control group (n = 18); (b) Gd exposure group (n = 13); and (c) statistical comparison of control and exposure groups. Also shown (d) are concentrations and anomalies for 4 additional patients with inferred Gd exposure. Gd anomalies are calculated according to eqn (1), using Gd concentrations measured in cortical tissues, and normalized to the control group mean (Gd/Gda )N (eqn (2)). OA = osteoarthritis; F = osteoporotic fracture. Mean, median, and 95% confidence interval (CI) of the mean are listed for each data subset
Patient ID # |
Pathology |
Cortical |
Trabecular |
Sm (nmol/g) |
Gd (nmol/g) |
Tb (nmol/g) |
Gd/Gd*a (eqn (1)) |
(Gd/Gd*)Na (eqn (2)) |
Gd (nmol/g) |
Patient ID #11 is an outlier for all REE concentrations and is excluded from calculations of mean, median, and 95% CI of mean.
|
(a) Control group |
2 |
OA |
0.03 |
0.03 |
0.02 |
1.3 |
0.9 |
0.07 |
5 |
OA |
0.03 |
0.02 |
0.02 |
0.9 |
0.6 |
0.05 |
6 |
OA |
0.02 |
0.05 |
0.03 |
2.0 |
1.4 |
0.15 |
9 |
OA |
0.05 |
0.05 |
0.02 |
1.6 |
1.1 |
0.10 |
10 |
OA |
0.02 |
0.02 |
0.02 |
1.0 |
0.7 |
0.05 |
11a |
OA |
2.58 |
1.86 |
0.25 |
1.8 |
1.3 |
6.54 |
12 |
OA |
0.02 |
0.02 |
0.02 |
0.9 |
0.6 |
0.06 |
13 |
OA |
0.01 |
0.01 |
0.02 |
0.7 |
0.5 |
0.04 |
15 |
OA |
0.01 |
0.02 |
0.02 |
1.3 |
0.9 |
0.04 |
17 |
OA |
0.03 |
0.06 |
0.02 |
2.3 |
1.6 |
0.19 |
18 |
OA |
0.02 |
0.02 |
0.03 |
0.8 |
0.5 |
0.04 |
20 |
OA |
0.02 |
0.02 |
0.02 |
0.9 |
0.6 |
0.05 |
21 |
OA |
0.02 |
0.03 |
0.02 |
1.3 |
0.9 |
0.18 |
22 |
OA |
0.01 |
0.04 |
0.02 |
2.8 |
1.9 |
0.06 |
23 |
OA |
0.01 |
0.01 |
0.01 |
1.4 |
0.9 |
0.02 |
26 |
OA |
0.03 |
0.04 |
0.03 |
1.5 |
1.0 |
0.12 |
29 |
OA |
0.02 |
0.07 |
0.02 |
3.3 |
2.3 |
0.10 |
49 |
OA |
0.02 |
0.02 |
0.03 |
0.8 |
0.6 |
0.04 |
Control group statistics |
Meana |
0.02 |
0.03 |
0.02 |
1.45 |
1.00 |
0.08 |
Mediana |
0.02 |
0.02 |
0.02 |
1.28 |
0.88 |
0.06 |
95 % CIa |
0.016, 0.027 |
0.023, 0.041 |
0.020, 0.024 |
1.07, 1.83 |
0.74, 1.26 |
0.054, 0.107 |
(b) Gd exposure group |
16 |
OA |
0.01 |
20.8 |
0.02 |
1198 |
826 |
39.5 |
25 |
OA |
0.02 |
9.84 |
0.03 |
427 |
295 |
15.3 |
33 |
OA |
0.01 |
6.24 |
0.03 |
262 |
181 |
24.3 |
35 |
OA |
0.02 |
31.0 |
0.03 |
1207 |
832 |
28.8 |
36 |
OA |
0.02 |
14.3 |
0.02 |
743 |
512 |
15.8 |
68 |
OA |
0.02 |
10.1 |
0.03 |
397 |
274 |
20.2 |
85 |
OA |
0.02 |
11.4 |
0.03 |
461 |
318 |
34.8 |
1 |
F |
0.07 |
0.07 |
0.03 |
1.6 |
1.1 |
0.11 |
75 |
F |
0.02 |
1.50 |
0.02 |
81 |
56 |
2.58 |
79 |
F |
0.07 |
6.40 |
0.03 |
144 |
99 |
4.91 |
81 |
F |
0.03 |
3.19 |
0.02 |
136 |
93 |
3.33 |
87 |
F |
0.01 |
5.69 |
0.02 |
401 |
277 |
17.1 |
91 |
F |
0.04 |
1.18 |
0.02 |
43 |
29 |
1.80 |
Exposure group statistics |
Mean |
0.03 |
9.36 |
0.02 |
417 |
280 |
16.05 |
Median |
0.02 |
6.40 |
0.03 |
293 |
197 |
15.78 |
95% CI |
0.016, 0.040 |
4.09, 14.63 |
0.021, 0.028 |
180, 666 |
124, 460 |
8.09, 24.0 |
(c) 2 sample t-test comparison of control and exposure groups (normalized data (log(x)) |
p-value |
|
0.77 |
<0.001 |
0.81 |
<0.001 |
<0.001 |
<0.001 |
(d) Inferred Gd exposure |
27 |
OA |
0.02 |
12.9 |
0.02 |
601 |
414 |
22.58 |
28 |
OA |
0.02 |
14.4 |
0.03 |
587 |
405 |
28.04 |
80 |
F |
0.04 |
0.90 |
0.03 |
30 |
21 |
1.30 |
84 |
F |
0.04 |
1.06 |
0.02 |
40 |
27 |
1.94 |
Comparison of control and Gd exposure groups
Fig. 2 shows the concentrations of all REEs for the control group (n = 18) and exposure group (n = 13), normalized to average upper continental crust (UCC), which approximates local Rochester, NY soil composition. The approximately flat REE pattern seen for control group samples demonstrates the ‘normal’ pattern of REE uptake relative to natural abundance in soils, food, and water. Gd concentrations in 17 of the 18 control group bone samples fall within a narrow range, with cortical tissue concentrations ranging from 0.013 to 0.070 nmol/g. One outlier (patient ID#11) has a cortical Gd concentration of 1.86 nmol/g (Table 3). For the control group (excluding the outlier, n = 17), the mean cortical tissue Gd concentration is 0.03 nmol/g (with a 95% CI of 0.023, 0.041 nmol/g), and the median is 0.02 nmol/g (Table 3). Trabecular tissue concentrations range from 0.044 to 0.190 nmol/g, excluding the outlier (ID #11) of 6.54 nmol/g (Table 3). For the control group (n = 17), the mean trabecular tissue Gd concentration is 0.08 nmol/g (with a 95% CI of 0.054, 0.107 nmol/g), and the median is 0.06 nmol/g (Table 3). As shown in Fig. 2, patient ID #11 is an outlier for all REEs, not only Gd. The patient’s Gd anomaly is comparable to those of other control group patients (Gd/Gd* = 1.8). Average Gd anomaly (Gd/Gd*) for the control group is 1.45 (95% CI: 1.07, 1.83).
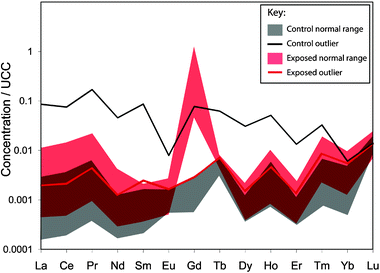 |
| Fig. 2 Rare earth element concentrations in cortical tissues for the control group (n = 18) and exposure group (n = 13) normalized to the upper continental crust (UCC) REE concentrations. The normal ranges for control and exposure groups are shown in grey and red, respectively (note that the dark red is overlap between control and exposure ranges). Outliers are shown separately. The occurrence of a control group outlier whose Gd concentration falls within the normal range for the exposure group reveals the necessity of calculating a Gd anomaly relative to abundance of neighboring REEs (eqn (1)). The outlier (patient ID #11) is anomalous for all REEs, and has a Gd anomaly similar to other control group patients. | |
Of the 13 patients in the exposure group, 12 have anomalously high bone concentrations of Gd with respect to the concentrations of other REEs (Fig. 2). Gd concentrations in the exposure group ranged up to 31 nmol/g in cortical bone with a mean of 9.36 nmol/g (95% CI 4.09, 14.63 nmol/g) and a median of 6.40 nmol/g. In trabecular tissues Gd concentrations ranged up to 39.51 nmol/g with a mean of 16.05 nmol/g (95% CI 8.09, 24.00 nmol/g) and median of 15.78 nmol/g (Table 3). One outlier (patient ID #1) has Gd concentrations and Gd anomaly comparable to the control group (e.g. 0.071 nmol/g cortical; Gd/Gd* = 1.3). Excluding the outlier, Gd anomalies (Gd/Gd*) in the exposure group range from 43 to 1207, with a mean of 417 (95% CI: 180, 666) (Table 3). Gd anomalies normalized to the control group mean, (Gd/Gd*)N, range from 30 to 832, with a mean of 280 (95% CI: 124, 460). Concentrations and anomalies have no correlation to time elapsed between Gd exposure and surgery, which ranges up to 8 years.
Gd concentrations for both cortical and trabecular tissues, and Gd anomalies, are significantly higher (p < 0.001) in Gd exposure patients (n = 13, including the outlier) than in control group patients (n = 17) (Table 3). Concentrations of REEs other than Gd were indistinguishable from the control group (Sm: p = 0.602; Tb: p = 0.559). The relative distributions of Gd concentration in the control and exposure groups are shown graphically in Fig. 3.
 |
| Fig. 3 Statistical distributions of normalized Gd anomaly (Gd/Gd*N) for cortical bone samples of control and Gd exposure patient groups (p < 0.001), and for both osteoarthritis and osteoporotic fracture patients exposed to Gd, which are also significantly different (p < 0.001; Table 4). | |
Comparison of Gd contrast agent brands
Of the 11 exposed patients for whom Gd contrast agent brand information is available, 6 were given Gd DTPA-BMA (Omniscan), and 5 were given Gd HP-DO3A (Prohance). No significant difference in bone Gd concentration or Gd anomaly was observed according to brand of contrast agent (cortical p = 0.94; trabecular p = 0.99; cortical (Gd/Gd*)N p = 0.98). Dosage and frequency information were insufficient to attempt correlation with bone Gd concentration.
Patients with incomplete medical histories
Four patients with incomplete medical histories have bone Gd concentrations and anomalies similar to those of the exposure group. While maximum (Gd/Gd*)N in the control group is 2.3, the 4 unknowns have (Gd/Gd*)N ranging from 21 to 414. These values occur at >50 sigma deviations from the control mean, and are very unlikely to result from anything other than exposure to Gd-based contrast agents.
Comparison of osteoarthritis and fracture patients exposed to Gd
In comparing Gd incorporation into bone tissues of osteoarthritis and fracture patients, we exclude a statistical outlier with no Gd anomaly (patient ID #1), and include the 4 patients with inferred Gd exposure (Table 4). Fracture patients (n = 7) have significantly lower Gd concentrations, in both cortical and trabecular tissues, and Gd anomalies than osteoarthritis patients (n = 9) (p < 0.001; Table 4), with no overlap in 95% confidence intervals. Fig. 3 illustrates the different distributions of normalized Gd anomalies (Gd/Gd*)N for the two bone pathology groups.
Table 4 Statistical summaries of concentrations and anomalies (cortical) for patients exposed to Gd according to bone pathology: (a) osteoarthritis patients (n = 9); and (b) osteoporotic fracture patients (n = 7). Concentrations and anomalies are significantly lower in osteoporotic fracture patients (p < 0.001)
|
Gd Cortical (nmol/g) |
Gd Trabecular (nmol/g) |
Gd/Gd* (eqn (1)) |
(Gd/Gd*)N (eqn (2)) |
(a) Osteoarthritis patients exposed to Gd (n = 9) |
Mean |
14.33 |
25.49 |
654 |
451 |
Median |
12.89 |
24.34 |
587 |
405 |
95% CI |
8.54, 20.1 |
19.2, 31.8 |
392, 915 |
271, 631 |
(b) Fracture patients exposed to Gd (n = 7) |
Mean |
2.84 |
4.71 |
125 |
86 |
Median |
1.50 |
2.58 |
81 |
56 |
95% CI |
0.695, 4.99 |
0, 9.89 |
5, 245 |
3, 169 |
(c) Statistical comparison: 2 sample t-test (normalized data (log(x)) |
p-value |
<0.001 |
<0.001 |
<0.001 |
<0.001 |
Discussion
Gd incorporation into bone tissues
Anomalously high Gd concentrations occur in both cortical and trabecular bone tissues of patients exposed to Gd-based chelated contrast enhancement agents (Gd-DPTA-BMA and Gd HP-DO3A). These results confirm the assertion by other authors that in vivo clinical exposure to Gd chelates results in Gd incorporation into body tissues.1,15,23 Other researchers have categorically demonstrated the potential toxicology, severe adverse health effects, and pathology associated with exposure to free ionic Gd3+.8,9,51,52 A previous study has shown that high clinical doses of chelated Gd, given immediately before hip replacement surgery, result in Gd concentrations measurable in the bone tissue averaging 2.8 (±2.5) to 7.5 (±4.9) nmol/g depending on the type of contrast agent administered.31 Our results for patients exposed to Gd-based contrast agents agree with this study, although our Gd values range up to three times higher, and include patients with up to >8 years time elapsed between exposure and surgical removal of the analyzed bone. We observe no correlation between elapsed time and bone Gd concentration. In addition, there is no significant difference in bone Gd concentration between patients exposed to Gd-DPTA-BMA (Omniscan; n = 6) and Gd HP-DO3A (Prohance; n = 5) in this study.
In the environment, Gd exists in trace concentrations of approximately 3 to 6 μmol/g or less in most water, soil, and rock.53 However, existing data on naturally occurring Gd concentrations in human bone are sparse. Our results are consistent with one study on fossilized bone with very low Gd concentrations (0.011 to 1.00 nmol/g).54 ‘Normal’ bone Gd concentrations, as represented by our control group, occur in a narrow range (95% CI: 0.023, 0.041 nmol/g in cortical tissue; n = 17). Our control group also includes an individual with concentrations of all REEs two orders of magnitude greater than those of other control group patients (Gd = cortical: 1.86 nmol/g; trabecular: 6.54 nmol/g). This occurrence indicates that it is necessary to measure all REE elements (at least Sm and Tb) to assess the magnitude of the Gd anomaly and determine medical exposure to Gd-based contrast agents. For example, without observing other REEs and calculating Gd anomaly, the control group outlier could be mistaken for an exposed patient.
Our study documents the marked difference between ‘normal’ bone Gd concentrations and those of Gd-chelate exposed patients (up to >800 times greater in the exposure group). Gd also remains elevated in both trabecular and cortical bone tissue for greater than 8 years, indicating retention of Gd in the body far longer than previously observed. Several previous studies assert that the in vivo use of Gd-based contrast agents is safe because of the rapid elimination of the chelated agent, with a half-life of only 30 to 60 minutes,21,22,31 without significant release of free Gd3+.5,55–57 Conversely, other recent publications indicate that chelated Gd breaks down in vivo, resulting in the release of Gd3+ to the blood stream and incorporation into the body.8,15,58,59Gd3+ ion release from Gd-DPTA-BMA (Omniscan) has also been shown to occur via competitive transmetallation reactions on the cell membrane, mediated by ionic components (specifically phosphate) in incubation media analogous to extracellular fluid.19
To further address the question of Gd clearance, we have estimated the total skeletal Gd load in patients exposed to Gd contrast imaging procedures. Assuming 3670 g of dry, fat-free bone in a 70 kg male skeleton,60 and using the lower Gd value range for cortical rather than trabecular bone (Table 4) the total skeletal Gd ranges from 10 nmol (Fracture group) to 53 nmol (OA group). At a typical contrast agent dose of 21 mmol Gd-chelate for a 70 kg patient (0.3 mmol/kg), the Gd retained in the skeleton represents only 0.05–0.25% of the total imaging dose. Thus, while more than 99.7% of the total injected Gd appears to have been cleared, there is significant long-term Gd retention by the skeleton.
The process of bone dissolution by osteoclasts and new bone formation by osteoblasts steadily remodels the skeleton in adults. Annual rates of bone remodeling are typically 5–15% for the skeleton as a whole, but can exceed 25% for dynamically loaded trabecular bone in the hip.40 The Ca content of bone mineral is tightly regulated (26.4% of bone dry wt, 40% of ash wt) by the osteoblast and the stoichiometric requirements of the hydroxyapatite-type crystal lattice [Ca10(PO4)6(OH)2]. However, the incorporation of other elements (Mg, Sr, Pb, Gd, REE, etc.) is proportional to their concentrations in the extracellular fluid or blood plasma,61 as well as their efficiency of transport by the osteoblast, their ionic radius and steric fit in vacancies in the crystal lattice, and the solubility products of their phosphate and carbonate complexes. Our data includes measurements of total Gd from complete nitric acid digestions of bone mineral without speciation methods. This analytical method does not allow conclusive identification of the Gd species incorporated into bone (chelate vs. other ionic complex vs. free Gd3+). Nonetheless our data clearly indicate that a small fraction of Gd does not clear the body following the application of Gd-based contrast agents and is incorporated and retained in bone for several years. We suspect that ionic Gd3+ is released from Gd-chelates by transmetallation reactions and subsequently incorporated into the carbonated calcium hydroxyapatite mineral phase of bone. In this proposed process Gd, a known Ca analog, may rapidly incorporate into bone mineral by substituting for Ca, given its similar ionic radius (Gd3+ 107.8 pm; Ca2+ 114 pm) and the high affinity of rare earth elements to hydroxyapatite minerals.62 Gd incorporation by this process would result in the potential for toxic metal exposure to the cells within bone tissue (osteoblasts, osteoclasts, endothelial cells, hematopoietic bone marrow, etc.) e.g. Gd disrupts cellular processes and is a highly efficient calcium antagonist.9,12
The incorporation of Gd chelates or other Gd complexes into bone remains a possibility in the absence of suitable speciation methods. Whether Gd is incorporated into bone in chelated form or other Gd complexes, our data indicate that bone tissue effectively stores and sequesters Gd for at least 8 years. While this sequestration may protect vital organs, toxic metal release can occur during bone resorption and remodeling processes. During the resorption process osteoclasts secrete HCl acid to dissolve existing bone mineral, effectively lowering the conditions in the resorption compartment to an approximate pH of 4.33,39 Because the thermodynamic stability of Gd chelates is significantly decreased at lower pH63 (i.e. there is increased potential for transmetallic exchange),63 ‘normal’ osteoclast-mediated bone resorption presents an avenue for the release of ionic Gd3+, whether initially incorporated in the form of a Gd-chelate, other Gd complexes, or ionic Gd3+.
Regardless, our data signals the need to consider both the potential effects of Gd incorporation on bone tissue itself, as well as the possibility of long term or time-delayed adverse health impacts associated with the use of Gd-based contrast agents. Gd incorporation into bone could negatively impact bone health similar to other toxic metals (e.g. Pb, Cd) which alter bone cellular processes, compromise bone health by altering bone cell signaling and molecular pathways,64 inhibit fracture healing,64 and alter the metabolism of osteoblasts and osteoclasts responsible for remodeling bone tissue.65,66
Impact of bone pathology on Gd incorporation
Our dataset, which includes both osteoarthritis and osteoporotic fracture patients medically exposed to Gd-based contrast agents, provides a unique opportunity to investigate the effects of bone pathology on metal incorporation and retention in bone. Bone samples from fracture patients exposed to Gd have statistically lower Gd concentrations and Gd anomalies (p < 0.001; Table 4; Fig. 3) than those of similarly exposed osteoarthritis patients. We suggest two possible explanations for the difference between osteoporotic fracture patients and osteoarthritis patients: (1) fracture patients affected by osteoporosis have lower rates of osteoblastic new bone formation,40 and may therefore incorporate less Gd into remodeling bone at the time of exposure; and (2) bone tissue in osteoporosis undergoes increased bone mineral loss that releases trace metals, including Gd, sequestered in bone tissue. For example, Pb is released from the bone of post-menopausal women, who undergo increased bone mineral loss.34,67 Accordingly, the bone remodeling occurring for many years following Gd exposure could constitute a health hazard particularly for individuals with compromised renal function and/or increased bone resorption. Bone remodeling and metal release may occur at slower rates in normal bone and/or osteoarthritic bone than in osteoporotic bone. Many unknown variables impact the potential toxicity including: Gd-uptake rates in normal, osteoporotic and osteoarthritic bone, residence times of free Gd or Gd chelates, rate of reincorporation into new bone during remodeling, and post-dosage clearance mechanisms. Patients with higher rates of bone turnover (e.g. osteoporosis patients, pregnant, lactating and postmenopausal women, and developing fetuses) may have an increased health risk associated with the potential rapid release of toxic elements stored in bone tissue.
Conclusions
(1) Bone tissues of patients exposed to Gd-chelate contrast agents have anomalously high Gd concentrations, indicating that Gd is incorporated into bone mineral as a result of contrast imaging and does not completely clear the body after exposure to the contrast agents. While the exact chemical species of Gd was not conclusively determined in this study, these results indicate that Gd chelates may not be stable in vivo and may expose patients to potentially toxic free Gd3+. Clearly further research is required to determine the chemical form of Gd incorporated into bone to fully understand the consequences and potential toxicity of Gd-based contrast enhancement agents.
Previous studies have measured Gd at concentrations from 2.8 to 7.5 nmol/g in bones surgically removed 3 days after exposure to high clinical doses of a Gd-chelate. Our results for patients exposed to Gd chelates show similar but higher abundance in bone. ICP-MS sample analysis enables us to establish ‘normal’ Gd levels in the control group by accurately measuring low concentrations of Gd incorporated into bone from natural sources (e.g. food, soils, water) at a mean of 0.03 nmol/g in cortical tissue (95% CI: 0.023, 0.041 nmol/g) and 0.08 nmol/g in trabecular tissue (95% CI: 0.054, 0.107 nmol/g). We also record the occurrence of an individual with high concentrations of all rare earth elements (REEs), including Gd at a concentration similar to that of a patient exposed to a Gd contrast agent. This proves the necessity of calculating a Gd anomaly from the abundances of adjacent REEs; we normalize Gd anomaly to our control group mean to isolate the amount of Gd in bone attributable to exposure to Gd chelate contrast agents. Gd anomalies in exposed patients range up to 832 with a mean of 280 (95% CI: 124, 460).
(2) Gd is retained in bone tissues of exposed patients for longer than 8 years post-exposure.
(3) Gd concentrations and anomalies are significantly lower in the bone tissues of osteoporotic fracture patients exposed to Gd chelates than similarly exposed osteoarthritis patients (p < 0.001) indicating a difference in the mechanism of metal incorporation according to pathological disease. This could indicate that either: (a) increased rates of bone resorption associated with osteoporosis allow removal and clearance of Gd more rapidly than osteoarthritic bone; or (b) osteoporotic bone incorporates less Gd at the time of exposure because of decreased rates of bone formation and/or metal incorporation. The former would indicate increased risk of endogenous release of Gd into the bloodstream for all patients affected by increased bone resorption (e.g. osteoporosis patients, pregnant, lactating and menopausal women, developing fetuses).
(4) Gd incorporation may affect bone quality and health similar to other toxic trace metals. The impact of elevated Gd concentration on bone cellular activity requires further investigation.
(5) Given the known toxicity and potential adverse health effects resulting from exposure to free Gd3+, it is essential to investigate and determine the mechanism of incorporation and long-term retention of Gd in bone from Gd-chelate contrast agents.
Acknowledgements
The authors wish to thank the anonymous reviewers whose input substantially improved the manuscript.
References
- C. Thakral, J. Alhariri and J. L. Abraham, Long-term Retention of Gadolinium in Tissues from Nephrogenic System Fibrosis Patient after Multiple Gadolinium-enhanced MRI Scans: A Case Report and Implications, Contrast Media Mol. Imaging, 2007, 2, 199–205 Search PubMed.
- J. M. Idee, et al., Clinical and biological consequences of transmetallation induced by contrast agents for magnetic resonance imaging: a review, Fundam. Clin. Pharmacol., 2006, 20, 563–576 CrossRef CAS.
- V. M. Runge and J. R. Parker, Worldwide clinical safety assessment of gadoteridol injection: an update, Eur. Radiol., 1997, 7, S243–S245 CrossRef.
- H. Sato, S. Takahashi and Y. Kubota, Effects of gadolinium on the retention and translocation of Pu-239-hydroxide, Health Phys., 2001, 80, 164–169 CAS.
- H. J. Weinmann, R. C. Brasch, W. R. Press and G. E. Wesbey, Characteristics of gadolinium-DTPA complex–a potential NMR contrast agent, Am. J. Roentgenol., 1984, 142, 619–624 Search PubMed.
- V. M. Runge, et al., MR imaging section profile optimization – improved contrast and detection of lesions, Radiology, 1988, 167, 831–834.
- V. M. Runge, GD-DPTA-AN IV contrast agent for clinical MRI, Nucl. Med. Biol., 1988, 15, 37–44 CAS.
- D. R. Broome, et al., Gadodiamide-associated nephrogenic systemic fibrosis: Why radiologists should be concerned, American Journal of Roentgenology, 2007, 188, 586–592 Search PubMed.
- B. A. Biagi and J. J. Enyeart, Gadolinium blocks low-threshold and high-threshold calcium currents in pituitary-cells, Am. J. Physiol.: Cell Physiol., 1990, 259, C515–C520 CAS.
- F. G. Shellock and A. Spinazzi, MRI Safety Update 2008: Part I, MRI Contrast Agents and Nephrogenic Systemic Fibrosis, Am. J. Roentgenol., 2008, 191, 1129–1139 Search PubMed.
- R. A. Caldwell, H. F. Clemo and C. M. Baumgarten, Using gadolinium to identify stretch-activated channels: technical considerations, Am. J. Physiol.: Cell Physiol., 1998, 275, C619–C621 CAS.
- X. C. Yang and F. Sachs, Block of stretch-activated ion channels in xenopus oocytes by gadolinium and calcium-ions, Science, 1989, 243, 1068–1071 CrossRef CAS.
- J. E. Molgo, J. Delpozo, E. Banos and D. Angautpetit, Changes of quantal transmitter release caused by gadolinium ions at the frog neuromuscular-junction, Br. J. Pharmacol., 1991, 104, 133–138 CAS.
- M. F. Tweedle, The ProHance story: the making of a novel MRI contrast agent, Eur. Radiol., 1997, 7, S225–S230 CrossRef.
- J. L. Abraham and C. Thakral, Tissue distribution and kinetics of gadolinium and nephrogenic systemic fibrosis, Eur. J. Radiol., 2008, 66, 200–207 CrossRef.
- J. S. Mann, Stability of gadolinium complexes invitro and invivo, J. Comput. Assist. Tomo., 1993, 17, S19–S23 Search PubMed.
- J. P. Weigle and D. R. Broome, Nephrogenic systemic fibrosis: chronic imaging findings and review of the medical literature, Skeletal Radiol., 2008, 37, 457–464 CrossRef.
- S. Laurent, L. V. Elst and R. N. Muller, Comparative study of the physicochemical properties of six clinical low molecular weight gadolinium contrast agents, Contrast Media Mol. Imaging, 2006, 1, 128–137 Search PubMed.
- C. Cabella, et al., Cellular labeling with Gd(III) chelates: only high thermodynamic stabilities prevent the cells acting as ‘sponges’ of Gd3+ ions, Contrast Media Mol. Imaging, 2006, 1, 23–29 Search PubMed.
- G. R. Moran, R. E. Thornhill, J. Sykes and F. S. Prato, Myocardial viability imaging using Gd-DTPA: Physiological modeling of infarcted myocardium and impact on injection strategy and imaging time, Magn. Reson. Med., 2002, 48, 791–800 CrossRef CAS.
- O. J. Badero, L. Schlanger and D. Rizk, Gadolinium nephrotoxicity: case report of a rare entity and review of the literature, Clin. Nephrol., 2008, 70, 518–522 Search PubMed.
- M. E. Bartolini, et al., An investigation of the toxicity of gadolinium based MRI contrast agents using neutron activation analysis, Magn. Reson. Imaging, 2003, 21, 541–544 CrossRef.
- T. Grobner, Gadolinium-a specific trigger for the development of nephrogenic fibrosing dermopathy and nephrogenic systemic fibrosis?, Nephrol., Dial., Transplant., 2006, 21, 1104–1108 CAS.
- P. Marckmann, L. Skov, K. Rossen, J. G. Heaf and H. S. Thomsen, Case-control study of gadodiamide-related nephrogenic systemic fibrosis, Nephrol., Dial., Transplant., 2007, 22, 3174–3178 CrossRef CAS.
- J. M. Idee and C. Corot, Anaphylactic shock after first exposure to a macrocyclic gadolinium chelate: A few comments, J. Allergy Clin. Immunol., 2008, 122, 215–216 CrossRef CAS.
- I. Ergun, et al., The safety of gadolinium in patients with stage 3 and 4 renal failure, Nephrol., Dial., Transplant., 2006, 21, 697–700 CrossRef.
- J. Kay, Nephrogenic systemic fibrosis: a gadolinium-associated fibrosing disorder in patients with renal dysfunction, Ann. Rheum. Dis., 2008, 67, iii66–iii69 CrossRef.
- R. E. Kalb, et al., Gadolinium-induced nephrogenic systemic fibrosis in a patient with an acute and transient kidney injury, Br. J. Dermatol., 2008, 158, 607–610 CrossRef.
- P. Marckmann, et al., Nephrogenic systemic fibrosis: Suspected causative role of gadodiamide used for contrast-enhanced magnetic resonance imaging, J. Am. Soc. Nephrol., 2006, 17, 2359–2362 CrossRef.
- C. Thakral and J. L. Abraham, Automated scanning electron microscopy and X-ray microanalysis for in situ quantification of Gadolinium deposits in skin, J. Electron Microsc., 2007, 56, 181–187 CrossRef.
- W. A. Gibby, K. A. Gibby and W. A. Gibby, Comparison of Gd DTPA-BMA (Omniscan) versus Gd HP-DO3A (ProHance) Retention in Human Bone Tissue by Inductively Coupled Plasma Atomic Emission Spectroscopy, Invest. Radiol., 2004, 39, 138–142 CrossRef.
- H. C. Skinner, W. Biominerals, Mineral. Mag., 2005, 69, 621–641 CrossRef CAS.
- J. D. Pasteris, B. Wopenka and E. Valsami-Jones, Bone and tooth mineralization: Why apatite?, Elements, 2008, 4, 97–104 CrossRef CAS.
- M. Berglund, A. Akesson, P. Bjellerup and M. Vahter, Metal-Bone Interact., 2000, 219–225 Search PubMed.
- S. G. Dahl, et al., Incorporation and distribution of strontium in bone, Bone, 2001, 28, 446–453 CrossRef CAS.
- A. L. Boskey, Biomineralization: An overview, Connect. Tissue Res., 2003, 44(Suppl. 1), 5–9 CrossRef CAS.
- A. L. Boskey, Mineral analysis provides insights into the mechanism of biomineralization, Calcif. Tissue Int., 2003, 72, 533–536 CAS.
- B. Gulson, et al., Skeletal lead release during bone resorption: Effect of bisphosphonate treatment in a pilot study, Environ. Health Perspect., 2002, 110, 1017–1023.
- Z. P. Li, K. M. Kong and W. L. Qi, Osteoclast and its roles in calcium metabolism and bone development and remodeling, Biochem. Biophys. Res. Commun., 2006, 343, 345–350 CrossRef CAS.
- E. F. Eriksen, et al., Cancellous bone remodeling in type-I (postmenopausal) osteoporosis-quantitative assessment of rates of formation, resorption and bone loss at tissue and cellular-levels, J. Bone Miner. Res., 1990, 5, 311–319.
- B. L. Gulson, et al., Contribution of tissue lead to blood lead in adult female subjects based on stable lead-isotope methods, J. Lab. Clin. Med., 1995, 125, 703–712.
- S. J. Rothenberg, et al., Changes in serial blood lead levels during pregnancy, Environ. Health Perspect., 1994, 102, 876–880 CrossRef.
- B. L. Gulson, et al., Pregnancy increases mobilization of lead from maternal skeleton, J. Lab. Clin. Med., 1997, 130, 51–62 CrossRef CAS.
- V. Chavagnac, et al., Towards the development of a fossil bone geochemical standard: An inter-laboratory study, Anal. Chim. Acta, 2007, 599, 177–190 CrossRef CAS.
- NIST. Bone Meal Standard Reference Material Certificate 1486. in Standard Reference Materials (ed. N.I. Technology, o.S.a.) (2007).
- USEPA. US EPA SW-846 Method 6020A. Analysis of soils and sediments by inductively coupled plasma- mass spectrometry. (ed. U.S. Agency, E.P.) (1991).
- USEPA. EPA 200.8 Determination of trace elements in waters and waste waters by inductively copuled plasma- mass spectrometry. (ed. U.S. Environmental Protection Agency, O.o.R.a.D.) (1994).
- G. J. Long and J. D. Winefordner, Limit of detection, a closer look at the IUPAC definition, Anal. Chem., 1983, 55, 712A–724A CrossRef CAS.
- USEPA. Title 40 of the US Code of Federal Regulations, Part 136, Appendix B. (ed. Administration, O.o.t.F.R.N.A.a.R.) (Washington, DC, 1993).
- M. Bau and P. Dulski, Anthropogenic origin of positive gadolinium anomalies in river waters, Earth Planet. Sci. Lett., 1996, 143, 245–255 CrossRef CAS.
- S. M. Rocklage, The relationship between thermodynamics and the toxicity of gadolinium complexes, Abstr. Pap. Am. Chem. Soc., 1991, 201, 457.
- S. K. Morcos, Nephrogenic systemic fibrosis following the administration of extracellular gadolinium based contrast agents: is the stability of the contrast agent molecule an important factor in the pathogenesis of this condition?, Br. J. Radiol., 2007, 80, 73–76 Search PubMed.
-
Rare earth element concentrations, speciation, and fractionation along groundwater flow paths; the Carrizo Sand (Texas) and Upper Floridan aquifers, ed. J. Tang and K. H. Johannesson, Springer, Dordrecht, Netherlands, 2005, pp. 223–251 Search PubMed.
- C. N. Trueman, A. K. Behrensmeyer, R. Potts and N. Tuross, High-resolution records of location and stratigraphic provenance from the rare earth element composition of fossil bones, Geochim. Cosmochim. Acta, 2006, 70, 4343–4355 CrossRef CAS.
- B. Hamm, et al., Phase-I clinical-evaluation of GD-EOB-DTPA as a hepatobiliary Mr contrast agent-safety, Pharmacokinetics and MR-imaging, Radiology, 1995, 195, 785–792 Search PubMed.
- V. M. Runge, et al., A clinical comparison of the safety and efficacy of MultiHance (gadobenate dimeglumine) and Omniscan (gadodiamide) in magnetic resonance imaging in patients with central nervous system pathology, Invest. Radiol., 2001, 36, 65–71 CrossRef.
- O. Dohr, R. Hofmeister, M. Treher and H. Schweinfurth, Preclinical safety evaluation of Gd-EOB-DTPA (Primovist), Invest. Radiol., 2007, 42, 830–841 CrossRef.
- J. L. Abraham, C. Thakral, L. Skov, K. Rossen and P. Marckmann, Dermal inorganic gadolinium concentrations: evidence for in vivo transmetallation and long-term persistence in nephrogenic systemic fibrosis, Br. J. Dermatol., 2008, 158, 273–280 CAS.
- D. R. Broome, Nephrogenic systemic fibrosis associated with gadolinium based contrast agents: A summary of the medical literature reporting, Eur. J. Radiol., 2008, 66, 230–234 CrossRef.
- M. Trotter and B. B. Hixon, Changes in weight, density and percentage ash weight of skeleton from early fetal life through old AGE, Am. J. Phys. Anthropol., 1974, 41, 507–507.
- M. J. Glimcher, Bone: Nature of the calcium phosphate crystals and cellular, structural and physical chemical mechanisms in their formation, Rev. Mineral. Geochem., 2006, 64, 223–282 CrossRef CAS.
- USGS. Rare Earth Elements-Critical Resources for High Technology. in Fact Sheet (ed. USGS) 1-4 (2002).
- M. F. Tweedle, Physicochemical properties of gadoteridol and other magnetic-resonance contrast agents, Invest. Radiol., 1992, 27, S7–S6 CrossRef.
- J. J. Carmouche, et al., Lead exposure inhibits fracture healing and is associated with increased chondrogenesis, delay in cartilage mineralization and a decrease in osteoprogenitor frequency, Environ. Health Perspect., 2005, 113, 749–755.
- J. E. Puzas, M. J. Sickel and M. E. Felter, Osteoblasts and chondrocytes are important target-cells for the toxic effects of lead, Neurotoxicology, 1992, 13, 783–788 CAS.
- T. L. Dowd, J. F. Rosen, L. Mints and C. M. Gundberg, The effect of Pb2+ on the structure and hydroxyapatite binding properties of osteocalcin, Biochim. Biophys. Acta, Mol. Basis Dis., 2001, 1535, 153–163 CrossRef CAS.
- K. R. Mahaffey, J. L. Annest, J. Roberts and R. S. Murphy, National estimates of blood lead levels-united-states 1976–1980-Association with selected demographic and socio-economic factors, New Engl. J. Med., 1982, 307, 573–579 CAS.
|
This journal is © The Royal Society of Chemistry 2009 |
Click here to see how this site uses Cookies. View our privacy policy here.