DOI:
10.1039/B906273D
(Minireview)
Energy Environ. Sci., 2009,
2, 956-961
Received
30th March 2009
, Accepted 1st May 2009
First published on 11th May 2009
Abstract
Due to their unusual sets of properties, ionic liquids have many important applications in devices and processes for the production, storage and efficient use of energy and other resources.
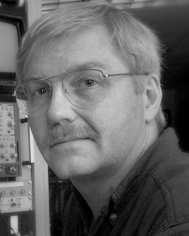 James F. Wishart | James F. Wishart received a BS in Chemistry from the Massachusetts Institute of Technology in 1979 and a PhD in Inorganic Chemistry from Stanford University in 1985 under the direction of Prof. Henry Taube. After a postdoctoral appointment at Rutgers University he joined the Brookhaven National Laboratory Chemistry Department in 1987. His research interests include ionic liquids, radiation chemistry, electron transfer, and new technology and techniques for pulse radiolysis. |
Broader context
During the early phases of the rapid growth of interest in ionic liquids, their “green” and environmentally-friendly characteristics were praised perhaps a little too enthusiastically. Ionic liquids, like any materials, are not intrinsically green; their environmental benefits accrue through the ways they are employed. As our understanding of ionic liquids and their novel combinations of tunable properties has grown, it is possible to see many opportunities for ionic liquids to contribute to the sustainable production, storage and efficient use of energy as components of advanced devices and processes. This brief review provides a basic introduction to ionic liquids and their interesting properties, and enumerates how these properties are being applied to improve the ways in which we generate, store, and consume energy.
|
Introduction
Ionic liquids (ILs) are defined (somewhat arbitrarily) as molten salts whose fusion temperatures are at or below 100 °C. Although their history extends back almost a century,1 they have received a burgeoning amount of attention in the present decade. While it may have been tempting a few years ago to view the interest in the field as a passing fad, the literature on ionic liquids continues to grow rapidly and to diversify into new areas. Ionic liquids are here to stay.1
The attraction of ionic liquids (ILs) lies in their remarkable set of properties when compared to conventional solvents. As salts consisting of distinct anions and cations, ILs are inherently binary (or higher order) systems. The anions and cations can be independently selected to tune the IL's physicochemical properties (melting point, conductivity, viscosity, density, refractive index, etc.) while at the same time introducing specific features for a given application (hydrophobicity vs. hydrophilicity, controlling solute solubility, adding functional groups for catalysis/reactivity purposes, chirality, etc.) Examples of typical IL anions and cations are shown in Fig. 1, however these are only a sample of the infinite variety available. Generally speaking, various tricks, such as using bulky organic ions, fluorous substitution, and low-symmetry structures, are employed to prevent efficient ion lattice packing that would result in strong coulombic interactions and high-melting solids instead of room-temperature liquids.
Although the coulombic interactions in ILs are weakened by design, in most cases they remain strong enough to make the IL's vapour pressure negligible at room temperature. Herein lies one of the major distinctions of ILs – the low vapour pressure makes them combustion resistant, evaporation-proof, and suitable for vacuum applications, and the lack of a boiling point (below the decomposition temperature) means that many ILs have very wide temperature ranges in the liquid state. In some ILs the fluid range extends all the way down to the glass transition temperature (typically −110 to −50 °C). On the other hand, certain ILs can be distilled if they are heated in high vacuum,2 or, as in the case of protic ionic liquids, they are in equilibrium with volatile neutral molecules via a proton transfer reaction.3
Ionic liquids are intrinsically conductive since they typically contain on the order of 2–5 M electrolyte, however the viscosities of ionic liquids tend to be higher than conventional solvents, ranging from 20–30000 cP at room temperature. Nevertheless, low-viscosity ionic liquids often have room-temperature conductivities of 1–10 mS cm−1, comparable to or larger than that of 0.1 M aqueous KCl solution. The reciprocal relationship between the viscosities and conductivities of ionic liquids, and causes of non-ideality, have been carefully studied by Angell and coworkers,4 as well as other groups.5
A large fraction of the reported ionic liquids are amphiphilic due to the hydrocarbon side chains that festoon the cations or anions. Molecular dynamics simulations6 have shown that hydrophobic domains can be formed through hydrocarbon side-chain aggregation, and if the chains are long enough the domains can merge into a continuous structure within the IL interwoven with the polar domain. This results in the second major distinction between ILs and molecular solvents – their ability to dissolve and solubilize a wide range of polar and nonpolar materials that would not be simultaneously soluble in a conventional molecular solvent. Solutes can range from inorganic salts to hydrocarbons, synthetic and bio-polymers, lipids, sugars, proteins and even functional enzymes. ILs can dissolve wood and lignocellulosic materials,7 and they even show promise as less hazardous fixatives for embalming tissues.8
Due to the electrostatic constraints of this ionic fluid, the polar and nonpolar regions are forced to remain in intimate contact and the liquid is heterogeneous on the molecular scale. Concepts of polarity that are useful for understanding the nature of conventional solvents are ill-defined in ionic liquids.9 Empirical polarity measurements often give conflicting results, since probe molecules sample local environments to which they have the highest affinity, recalling the Indian fable of the blind men and the elephant. Long-range structural order can persist in ionic liquids as well, for example interfacial layering at charged surfaces.10 These features can give rise to new reactivity patterns, the preparation of hybrid materials, and the fabrication of advanced devices.
The introduction above provides only a brief overview of the features of ionic liquids that motivate their energy-related applications. Their utility manifests itself in wide-ranging aspects of energy production, storage and utilization.
Ionic liquids will play many roles in the production of energy, for example in the capture and conversion of solar energy into electricity, the conversion of raw biomass or fossil resources into cleaner fuels, and enabling advanced nuclear energy technologies.
Solar photoconversion
Due to their conductivity, low volatility, and thermal stability, ionic liquids have been extensively studied as electrolytes for dye-sensitized solar cells (DSSCs).11 DSSCs use a metal complex or organic dye absorbed onto a semiconductor, such as TiO2, which has been applied to an electrode. Capture of a photon by the dye causes the excited state to inject an electron into the TiO2. The oxidized dye is reduced by a charge carrier within the electrolyte, typically iodide anion. The electron and the oxidized species (“hole”) recombine at the counter electrode, completing the circuit.
Although ILs are conductive, mass transport of the hole carrier to the counter electrode can be a limiting factor for IL DSSC performance.12Iodide is the most utilized hole carrier because I0 can dimerize to form I2 and pick up an iodide anion to form I3−. A Grotthus-type exchange mechanism transferring I2 between I3− and I− has been invoked to explain the superior charge transport in iodide-based DSSCs.13 A large body of literature traces the improvement of IL-based DSSCs. The present state of the art employs mixtures of IL cations and anions (in addition to I− and I3−) to lower viscosity and thus optimize hole transport. The energy efficiencies of IL-based DSSCs are close to those of the best conventional solvent-based systems, with substantial improvements in durability.14
Solar thermal conversion
Another emerging technology for conversion of solar energy involves using arrays of reflectors to focus sunlight onto vessels or pipes containing a heat transfer fluid. The heated fluid is then conducted through a system that converts the heat into electricity, either through thermoelectric conversion or by powering a generator. The heated fluid can be stored in an insulated vessel for electric generation on demand. Fluids for these systems must endure a wide range of temperatures, from night-time lows in winter to daytime operating temperatures in excess of 200 °C. Many ILs are liquid and stable over that entire range, and several studies15 have supported their suitability for this application. In addition to large-scale electricity installations, the technology is being investigated to provide direct heat and air conditioning to hospitals and resorts in Mediterranean countries.16
Biofuel production
Biofuels such as ethanol, butanol and biodiesel represent a potentially sustainable, carbon-neutral transportation fuel source if they can be obtained from non-food biomass grown and harvested in an ecologically stable manner. One of the biggest challenges is that the bulk of the energy available from such biomass (corn stalks, switchgrass, softwood, etc.) is in the form of lignocellulose, a tight, covalent- and hydrogen bond-linked matrix of the carbohydrate polymers cellulose and hemicellulose and the phenolic polymer lignin. Plants owe their sturdiness to the durability of lignocellulose and its “recalcitrance” to chemical and biological attack. Breaking down lignocellulose into its components so that they can be converted to biofuels requires harsh chemical treatments or specialized organisms (e.g., fungi).
Happily, ionic liquids that have anions with good hydrogen bond accepting properties, such as Cl−, carboxylates, and oxoanions, can dissolve cellulose (and lignocellulose) by disrupting their networks of intramolecular hydrogen bonds.17 It is therefore possible to dissolve wood and other plant material.7,18 The dissolved cellulose (or lignocellulose) can be recovered by addition of water to the ionic liquid. The water breaks up the cellulose–anion hydrogen bonds and precipitates the cellulose in a structurally disrupted form that is easier for enzymes to cleave into fermentable sugars to make ethanol, for example.19 Several groups are working on the adaptation of ionic liquid pre-treatment of biomass for the production of ethanol and butanol. Ionic liquids could possibly be used to extract butanol out of dilute aqueous fermentation broths.20
Ionic liquids have been used to solubilize other natural biopolymers in addition to cellulose, such as silk21 and wool keratin,22 and to make hybrid biopolymer materials with exotic items such as magnetic nanoparticles23 and carbon nanotubes.24 ILs have enabled the exploitation of cheap, renewable biopolymers in many applications that used to be the province of fossil fuel-derived plastics.
Biodiesel is another alternative fuel that has received a lot of attention because of its compatibility with the present transportation infrastructure. Several groups have used ionic liquids to convert triglycerides to glycerol and biodiesel (fatty acid methyl esters) through transesterification by immobilized lipase25 or homogeneous catalysis.26
More efficient production of cleaner fossil fuels
Although alternative energy sources will increase in capacity over time, fossil fuels will remain a major source of energy for the foreseeable future. Ionic liquids can make their production and use cleaner. Desulfurization of petroleum feedstocks is vitally important for the prevention of air pollution viaSOx aerosols and the environmental destruction caused by acid rain, as well as for the protection of antipollution catalysts and fuel cells. Standard methods such as hydrodesulfurization require elevated temperatures and pressures, along with hydrogen gas.
Ionic liquids have been shown to be effective at extracting sulfur compounds from fuels under ambient conditions, including compounds that are difficult to remove through hydrogenation such as dibenzothiophene.27 Ionic liquid extraction has been coupled with oxidation by H2O2 to boost efficiency,28 in some cases catalyzed by polyoxometallates.29 Ionic liquids containing Lewis acidic metal complex anions also show high performance.30
Ionic liquids are also effective for extracting aromatic hydrocarbons from aromatic/aliphatic mixtures, and an engineering analysis has shown significant cost savings and process benefits could be obtained by ionic liquid-based removal of aromatics from naphtha cracker feed streams.31 Regardless of the ability of ILs to extract organic materials out of hydrocarbons, they show limited solubility in hydrocarbons for the same Coulombic reasons that make their vapour pressure very low. Consequently, they are not washed away, even over many process cycles, a bonus for their cost efficiency.
A cleaner, advanced nuclear fuel cycle
Nuclear power provides a significant fraction of the world's energy, and it is a major carbon-free source of continuous, base-supply electrical power generation. Although the proportion of renewable alternative energy production will continue to grow, international recognition32 of the urgent need to reduce CO2 emissions calls for the growth of nuclear power generation in the near term to avoid an even larger increase in greenhouse gases from fossil fuel-based generating stations. Still, serious technical and political problems persist in the handling and ultimate disposal of spent nuclear fuel and waste. Current approaches are not sufficient for future needs, and work is underway to design an advanced fuel cycle33 that recycles spent nuclear fuel to significantly minimize waste, extracts more energy from existing uranium supplies, and burns up plutonium and other transuranic elements so that they are no longer weapons proliferation risks nor hazards to future generations.
Such a sea change in technology requires the development of new, detailed spent fuel treatment and separations schemes that present many challenges for chemists. Ionic liquids can play key roles in advanced fuel separations systems because of their useful properties, including low volatility and combustibility, amphiphilicity, tuneable separations affinities including functionalization, high conductivity, wide liquidus range and high thermal stability. In addition, ionic liquids containing boron can dramatically reduce the risk of criticality accidents in the processing of fissile materials.34 Several groups are investigating the liquid/liquid extraction behaviour of ionic liquids for actinides and fission products (lanthanides, Cs, Sr, Tc, etc.).35 Binnemans recently published a review on lanthanides and actinides in ILs.36 ILs have been shown to be effective solvents for extraction, but in some cases the operative mechanism is ion exchange instead of true neutral extraction.37 Several studies have revealed the effect of IL anion and cation hydrophobicity on the balance between the mechanisms.38 In addition to liquid/liquid extraction, various elements can be selectively electrodeposited in metallic form, even if highly reactive, due to the high conductivity and wide electrochemical windows of selected ILs.39 The use of ionic liquids to electrodeposit a wide variety of metals and alloys has industrial and commercial applications far beyond the nuclear field.40,41
Instability under the radiation burden of spent nuclear fuel is a common problem for nuclear separations systems based on conventional solvents. Therefore it is important to understand the chemistry produced by irradiation of ionic liquids and its potential effects on separations processes. A review of this issue has recently been published.42 Ionic liquid radiolysis product studies have shown that ILs are generally resistant to radiation damage, and product distributions have been characterized.43EPR identification of radiation-induced radical species relates initial transient species to the ultimate product distributions.44,45 Unlike conventional hydrocarbon solvents that direct damage into the extractant molecules, thus reducing their efficiency, ionic liquids can be designed to direct damage away from the extractant, thereby increasing the durability of the separations system.42,44 There are numerous reasons to believe that the future of ionic liquids in advanced nuclear fuel recycling is bright.
Batteries and supercapacitors
The strongest motivation for the development of ionic liquids in the early years of the field was in their use as battery electrolytes, where their conductivity, wide electrochemical windows and liquidus ranges, and low volatility made them attractive for high performance applications such as thermally-activated batteries.46 The electrochemical properties of ionic liquids are intensively studied worldwide and several reviews47–50 and books41 have been published on the subject, which includes many uses of ILs that fall under other sections of this minireview. The field is so large that it is not possible to do it justice within this space; interested parties are urged to consult the reviews.
Ionic liquids have been studied in nearly every battery system imaginable, but their use in lithium batteries has received the greatest attention.47–49,51 Because of their chemical and thermal stability, ILs are a good choice for development of batteries with lithium metal anodes, although there are issues with film formation at the IL/Li interface that require further investigation.
Supercapacitors store energy through the formation of electrochemical double layers formed by the adsorption of ionic species on electrode surfaces.47,49,52 They are designed to have very large electrode surface areas and they can store much more charge than conventional dielectric capacitors. They can be charged and discharged rapidly, and thus have higher power densities than batteries, although their energy density is lower. Supercapacitors have many uses in mobile electrical systems and power distribution systems (regenerative braking, accelerating electric vehicles, load buffering, uninterruptible power supplies) that can markedly improve energy conservation. Supercapacitors can also enhance the performance characteristics of batteries and fuel cells. Because of the ionic nature, conductivity and durability of ILs, supercapacitors using them are actively being developed.47,49,52,53
Ionic liquids and efficient energy utilization
Ionic liquids impact energy utilization most directly through their application to fuel cells, but there are many other ways that they can make positive contributions indirectly through improved materials, devices and processes that conserve energy or other resources during their manufacture or operation. The example of metal electrodeposition has already been mentioned above.
As low-volatility conductive media with good thermal stability, ionic liquids are finding applications as fuel cell electrolytes and as proton conductors in proton exchange membranes, often in combination with polymer gels.48,54 Typically, “protic” ionic liquids, formed through the reaction of a strong acid with a base, are used as the proton conductors. However, fuel cells typically operate at temperatures over 100 °C. Under those conditions, reverse proton transfer to produce volatile neutral acid and base molecules leads to slow loss of the ionic liquid through evaporation of its molecular precursors. This problem can be avoided through the use of very strong acids or bases.55
Advanced devices and processes
Ionic liquids can be combined with electroactive polymers to make electrochromic windows or displays.56 Their durability, chemical inertness and low volatility promise gains in reliability and useful lifetime. In addition to changing color, the charging or discharging of conductive polymers can be coupled to IL ion migration and electrostriction to produce physical displacements, creating electromechanical actuators or “artificial muscles”.48,49,57 Turning signal transduction in the opposite direction, ILs can be used to make sensors for the electrochemical detection of gases, ions (selective electrodes), biomolecules, and electroactive species.58 Similarly, the principles used to design IL-based actuators could be applied to portable energy harvesting systems59
Catalysis and industrial applications
The use of ionic liquids in catalytic systems has been studied extensively1,60 Because many ILs are practically insoluble in hydrocarbons and supercritical CO2,61 particular attention has been paid to their use in phase-transfer catalysis, particularly when the catalyst can be “immobilized” in the IL (to prevent its loss and product contamination) by attaching a charged functionality.
The solubility of gases in ionic liquids and their general lack of volatility make them useful for gas capture or separation applications, for example using supported ionic liquid polymer membranes for separation of CO2, SO2, etc.,62 H2purification63 and storage of large gas volumes under mild conditions.64
Conclusion
Well over a dozen edited books, at least 14,000 articles, and hundreds of patents concerning ionic liquids have been written. This short minireview highlights the major ways that ILs can impact energy technologies from generation to consumption. Most of the examples mentioned are still in the research and development stage, but commercial application of ILs continues to move forward in many areas. It is hoped that this brief introduction to ionic liquids will inspire new ideas for IL uses among the readers of this review.
Acknowledgements
This work was supported by the U. S. Department of Energy, Office of Basic Energy Sciences, Division of Chemical Sciences under contract # DE-AC02-98CH10886.
References
- N. V. Plechkova and K. R. Seddon, Chem. Soc. Rev., 2008, 37, 123–150 RSC.
- M. J. Earle, J. M. S. S. Esperanca, M. A. Gilea, J. N. C. Lopes, L. P. N. Rebelo, J. W. Magee, K. R. Seddon and J. A. Widegren, Nature, 2006, 439, 831–834 CrossRef CAS.
- D. R. MacFarlane, J. M. Pringle, K. M. Johansson, S. A. Forsyth and M. Forsyth, Chem. Commun., 2006, 1905–1917 RSC.
- W. Xu, E. I. Cooper and C. A. Angell, J. Phys. Chem. B, 2003, 107, 6170–6178 CrossRef CAS.
- D. R. MacFarlane, M. Forsyth, E. I. Izgorodina, A. P. Abbott, G. Annat and K. Fraser, Phys. Chem. Chem. Phys., 2009 10.1039/b900201d.
- J. N. A. C. Lopes and A. A. H. Padua, J. Phys. Chem. B, 2006, 110, 3330–3335 CrossRef.
- D. A. Fort, R. C. Remsing, R. P. Swatloski, P. Moyna, G. Moyna and R. D. Rogers, Green Chem., 2007, 9, 63–69 RSC.
- P. Majewski, A. Pernak, M. Grzymislawski, K. Iwanik and J. Pernak, Acta Histochem., 2003, 105, 135–142 CrossRef CAS.
- H. Weingartner, Angew. Chem., Int. Ed., 2008, 47, 654–670 CrossRef.
- M. Mezger, H. Schroder, H. Reichert, S. Schramm, J. S. Okasinski, S. Schoder, V. Honkimaki, M. Deutsch, B. M. Ocko, J. Ralston, M. Rohwerder, M. Stratmann and H. Dosch, Science, 2008, 322, 424–428 CrossRef CAS.
- M. Gratzel, J. Photochem. Photobiol., C, 2003, 4, 145–153 CrossRef CAS.
- M. Gorlov and L. Kloo, Dalton Trans., 2008, 2655–2666 RSC; P. Wachter, M. Zistler, C. Schreiner, M. Berginc, U. O. Krasovec, D. Gerhard, P. Wasserscheid, A. Hinsch and H. J. Gores, J. Photochem. Photobiol., A, 2008, 197, 25–33 CrossRef CAS.
- R. Kawano and M. Watanabe, Chem. Commun., 2005, 2107–2109 RSC; N. Yamanaka, R. Kawano, W. Kubo, T. Kitamura, Y. Wada, M. Watanabe and S. Yanagida, Chem. Commun., 2005, 740–742 RSC.
- Y. Bai, Y. M. Cao, J. Zhang, M. Wang, R. Z. Li, P. Wang, S. M. Zakeeruddin and M. Gratzel, Nat. Mater., 2008, 7, 626–630 CrossRef CAS; D. Shi, N. Pootrakulchote, R. Z. Li, J. Guo, Y. Wang, S. M. Zakeeruddin, M. Gratzel and P. Wang, J. Phys. Chem. C, 2008, 112, 17046–17050 CrossRef CAS.
- L. Moens, D. M. Blake, D. L. Rudnicki and M. J. Hale, J. Sol. Energy Eng. Trans. ASME, 2003, 125, 112–116 Search PubMed; R. G. Reddy, Z. J. Zhang, M. F. Arenas and D. M. Blake, High Temp. Mater. Process., 2003, 22, 87–94 Search PubMed; M. E. Van Valkenburg, R. L. Vaughn, M. Williams and J. S. Wilkes, Thermochim. Acta, 2005, 425, 181–188 CrossRef CAS; I. Perissi, U. Bardi, S. Caporali, A. Fossati and A. Lavacchi, Sol. Energy Mater. Sol. Cells, 2008, 92, 510–517 CrossRef CAS.
- T. El Asmar, Desalination, 2008, 220, 600–611 CrossRef CAS.
- R. C. Remsing, G. Hernandez, R. P. Swatloski, W. W. Massefski, R. D. Rogers and G. Moyna, J. Phys. Chem. B, 2008, 112, 11071–11078 CrossRef CAS.
- I. Kilpelainen, H. Xie, A. King, M. Granstrom, S. Heikkinen and D. S. Argyropoulos, J. Agric. Food Chem., 2007, 55, 9142–9148 CrossRef; S. D. Zhu, Y. X. Wu, Q. M. Chen, Z. N. Yu, C. W. Wang, S. W. Jin, Y. G. Ding and G. Wu, Green Chem., 2006, 8, 325–327 RSC.
- A. P. Dadi, C. A. Schall and S. Varanasi, Appl. Biochem. Biotechnol., 2007, 137, 407–421 CrossRef.
- A. Chapeaux, L. D. Simoni, T. S. Ronan, M. A. Stadtherr and J. F. Brennecke, Green Chem., 2008, 10, 1301–1306 RSC.
- R. A. Mantz, D. M. Fox, J. M. Green, P. A. Fylstra, H. C. De Long and P. C. Trulove, Z. Naturfors. Sect. A, 2007, 62, 275–280 Search PubMed.
- H. B. Xie, S. H. Li and S. B. Zhang, Green Chem., 2005, 7, 606–608 RSC.
- N. Sun, R. P. Swatloski, M. L. Maxim, M. Rahman, A. G. Harland, A. Haque, S. K. Spear, D. T. Daly and R. D. Rogers, J. Mater. Chem., 2008, 18, 283–290 RSC.
- H. Zhang, Z. G. Wang, Z. N. Zhang, J. Wu, J. Zhang and H. S. He, Adv. Mater., 2007, 19, 698–704 CrossRef CAS.
- M. Gamba, A. A. M. Lapis and J. Dupont, Adv. Synth. Catal., 2008, 350, 160–164 CrossRef CAS; S. H. Ha, M. N. Lan, S. H. Lee, S. M. Hwang and Y. M. Koo, Enzyme Microb. Technol., 2007, 41, 480–483 CrossRef CAS.
- Q. Wu, H. Chen, M. H. Han, D. Z. Wang and J. F. Wang, Ind. Eng. Chem. Res., 2007, 46, 7955–7960 CrossRef CAS; B. A. D. Neto, M. B. Alves, A. A. M. Lapis, F. M. Nachtigall, M. N. Eberlin, J. Dupont and P. A. Z. Suarez, J. Catal., 2007, 249, 154–161 CrossRef.
- J. Esser, P. Wasserscheid and A. Jess, Green Chem., 2004, 6, 316–322 RSC; J. D. Holbrey, I. Lopez-Martin, G. Rothenberg, K. R. Seddon, G. Silvero and X. Zheng, Green Chem., 2008, 10, 87–92 RSC.
- L. Lu, S. F. Cheng, J. B. Gao, G. H. Gao and M. Y. He, Energy Fuels, 2007, 21, 383–384 CrossRef CAS; D. S. Zhao, R. Liu, J. L. Wang and B. Y. Liu, Energy Fuels, 2008, 22, 1100–1103 CrossRef CAS.
- W. S. Zhu, H. M. Li, X. Hang, Y. S. Yan, H. D. Lu and J. X. Xia, Energy Fuels, 2007, 21, 2514–2516 CrossRef CAS.
- C. P. Huang, B. H. Chen, J. Zhang, Z. C. Liu and Y. X. Li, Energy Fuels, 2004, 18, 1862–1864 CrossRef CAS; R. Schmidt, Energy Fuels, 2008, 22, 1774–1778 CrossRef CAS; H. S. Gao, J. M. Xing, Y. G. Li, W. L. Li, Q. F. Liu and H. Z. Liu, Sep. Sci. Technol., 2009, 44, 971–982 CrossRef CAS.
- G. W. Meindersma and A. B. de Haan, Chem. Eng. Res. Design, 2008, 86, 745–752 Search PubMed.
-
Climate Change 2007 Synthesis Report, Intergovernmental Panel on Climate Change, Geneva, 2007 Search PubMed.
-
K. L. Nash, in Separations for the Nuclear Fuel Cycle in the 21st Century, ed. G. J. Lumetta, K. L. Nash, S. B. Clark and J. I. Friese, Amer. Chem. Soc., Washington, 2006, pp. 21–40.
- C. D. Harmon, W. H. Smith and D. A. Costa, Radiat. Phys. Chem., 2001, 60, 157–159 CrossRef CAS.
- V. A. Cocalia, K. E. Gutowski and R. D. Rogers, Coord. Chem. Rev., 2006, 250, 755–764 CrossRef;
K. E. Gutowski, N. J. Bridges, V. A. Cocalia, S. K. Spear, A. E. Visser, J. D. Holbrey, J. H. Davis and R. D. Rogers, in Ionic Liquids IIIB: Fundamentals, Progress, Challenges and Opportunities: Transformations and Processes, ed. R. D. Rogers and K. R. Seddon, Amer. Chem. Soc., Washington, 2005, pp. 33–48.
- K. Binnemans, Chem. Rev., 2007, 107, 2592–2614 CrossRef CAS.
- A. E. Visser and R. D. Rogers, J. Solid State Chem., 2003, 171, 109–113 CrossRef CAS;
M. L. Dietz, J. A. Dzielawa, M. P. Jensen, J. V. Beitz and M. Borkowski, in Ionic Liquids IIIB: Fundamentals, Progress, Challenges and Opportunities: Transformations and Processes, ed. R. D. Rogers and K. R. Seddon, Amer. Chem. Soc., Washington, 2005, pp. 2–18; V. A. Cocalia, J. D. Holbrey, K. E. Gutowski, N. J. Bridges and R. D. Rogers, Tsinghua Sci. Tech., 2006, 11, 188–193 Search PubMed.
- H. Luo, S. Dai, P. V. Bonnesen, T. J. Haverlock, B. A. Moyer and A. C. Buchanan, Solvent Extr. Ion Exch., 2006, 24, 19–31 CrossRef CAS; M. L. Dietz and D. C. Stepinski, Talanta, 2008, 75, 598–603 CrossRef CAS.
- P. Y. Chen and C. L. Hussey, Electrochim. Acta, 2005, 50, 2533–2540 CrossRef CAS; T. Tsuda, C. L. Hussey, H. M. Luo and S. Dai, J. Electrochem. Soc., 2006, 153, D171–D176 CrossRef CAS.
- A. P. Abbott and K. J. McKenzie, Phys. Chem. Chem. Phys., 2006, 8, 4265–4279 RSC.
-
H. Ohno, ed., Electrochemical Aspects of Ionic Liquids, John Wiley & Sons, New York, 2005 Search PubMed;
F. Endres, D. MacFarlane and A. Abbott, Electrodeposition from Ionic Liquids, Wiley, New York, 2008 Search PubMed.
-
J. F. Wishart and I. A. Shkrob, in Ionic Liquids: From Knowledge to Application, ed. R. D. Rogers, N. V. Plechkova and K. R. Seddon, Amer. Chem. Soc., Washington, 2009, in press.
- D. Allen, G. Baston, A. E. Bradley, T. Gorman, A. Haile, I. Hamblett, J. E. Hatter, M. J. F. Healey, B. Hodgson, R. Lewin, K. V. Lovell, B. Newton, W. R. Pitner, D. W. Rooney, D. Sanders, K. R. Seddon, H. E. Sims and R. C. Thied, Green Chem., 2002, 4, 152–158 RSC; L. Berthon, S. I. Nikitenko, I. Bisel, C. Berthon, M. Faucon, B. Saucerotte, N. Zorz and P. Moisy, Dalton Trans., 2006, 2526–2534 RSC; E. Bosse, L. Berthon, N. Zorz, J. Monget, C. Berthon, I. Bisel, S. Legand and P. Moisy, Dalton Trans., 2008, 924–931 RSC.
- I. A. Shkrob, S. D. Chemerisov and J. F. Wishart, J. Phys. Chem. B, 2007, 111, 11786–11793 CrossRef CAS.
- I. A. Shkrob and J. F. Wishart, J. Phys. Chem. B, 2009, 113, 5582–5592 CrossRef CAS.
-
J. S. Wilkes, in Ionic Liquids, ed. R. D. Rogers and K. R. Seddon, Amer. Chem. Soc., Washington, 2002, pp. 214–229.
- M. Galinski, A. Lewandowski and I. Stepniak, Electrochim. Acta, 2006, 51, 5567–5580 CrossRef CAS.
- D. R. Macfarlane, M. Forsyth, P. C. Howlett, J. M. Pringle, J. Sun, G. Annat, W. Neil and E. I. Izgorodina, Acc. Chem. Res., 2007, 40, 1165–1173 CrossRef CAS.
- T. Tsuda and C. L. Hussey, Electrochem. Soc. Interface, 2007, 16, 42–49 Search PubMed.
- P. Hapiot and C. Lagrost, Chem. Rev., 2008, 108, 2238–2264 CrossRef CAS.
- H. Sakaebe, H. Matsumoto and K. Tatsumi, Electrochim. Acta, 2007, 53, 1048–1054 CrossRef CAS; N. Byrne, P. C. Howlett, D. R. MacFarlane, M. E. Smith, A. Howes, A. F. Hollenkamp, T. Bastowe, P. Hale and M. Forsyth, J. Power Sources, 2008, 184, 288–296 CrossRef CAS.
- J. K. Chang, M. T. Lee, C. W. Cheng, W. T. Tsai, M. J. Deng and I. W. Sun, Electrochem. Solid-State Lett., 2009, 12, A19–A22 CrossRef CAS.
- C. Arbizzani, M. Biso, D. Cericola, M. Lazzari, F. Soavi and M. Mastragostino, J. Power Sources, 2008, 185, 1575–1579 CrossRef CAS.
- R. Devanathan, Energy Environ. Sci., 2008, 1, 101–119 RSC; C. A. Angell, N. Byrne and J. P. Elieres, Acc. Chem. Res., 2007, 40, 1228–1236 CrossRef CAS; R. F. de Souza, J. C. Padilha, R. S. Goncalves and J. Dupont, Electrochem. Commun., 2003, 5, 728–731 CrossRef CAS; J. P. Belieres and C. A. Angell, J. Phys. Chem. B, 2007, 111, 4926–4937 CrossRef CAS; H. Ye, J. Huang, J. J. Xu, N. Kodiweera, J. R. P. Jayakody and S. G. Greenbaum, J. Power Sources, 2008, 178, 651–660 CrossRef CAS.
- M. Yoshizawa, W. Xu and C. A. Angell, J. Am. Chem. Soc., 2003, 125, 15411–15419 CrossRef CAS; H. Luo, G. A. Baker, J. S. Lee, R. M. Pagni and S. Dai, J. Phys. Chem. B, 2009, 113, 4181–4183 CrossRef CAS; T. L. Greaves and C. J. Drummond, Chem. Rev., 2008, 108, 206–237 CrossRef CAS.
- W. Lu, A. G. Fadeev, B. H. Qi, E. Smela, B. R. Mattes, J. Ding, G. M. Spinks, J. Mazurkiewicz, D. Z. Zhou, G. G. Wallace, D. R. MacFarlane, S. A. Forsyth and M. Forsyth, Science, 2002, 297, 983–987 CrossRef CAS; C. Pozo-Gonzalo, D. Mecerreyes, J. A. Pomposo, M. Salsamendi, R. Marcilla, H. Grande, R. Vergaz, D. Barrios and J. M. Sanchez-Pena, Sol. Energy Mater. Sol. Cells, 2008, 92, 101–106 CrossRef CAS; L.
J. Ma, Y. X. Li, X. F. Yu, Q. B. Yang and C. H. Noh, Sol. Energy Mater. Sol. Cells, 2008, 92, 1253–1259 CrossRef CAS.
- B. J. Akle, M. D. Bennett and D. J. Leo, Sens. Actuators, A, 2006, 126, 173–181 CrossRef; K. Mukai, K. Asaka, K. Kiyohara, T. Sugino, I. Takeuchi, T. Fukushima and T. Aida, Electrochim. Acta, 2008, 53, 5555–5562 CrossRef CAS.
- D. Wei and A. Ivaska, Anal. Chim. Acta, 2008, 607, 126–135 CrossRef CAS.
- R. Tiwari, K. J. Kim and S. M. Kim, Smart Struct. Syst., 2008, 4, 549–563 Search PubMed.
- H. Olivier-Bourbigou and L. Magna, J. Mol. Catal., A, 2002, 182, 419–437 CrossRef; F. van Rantwijk and R. A. Sheldon, Chem. Rev., 2007, 107, 2757–2785 CrossRef; V. I. Parvulescu and C. Hardacre, Chem. Rev., 2007, 107, 2615–2665 CrossRef CAS; S. V. Malhotra, V. Kumar and V. S. Parmar, Curr. Org. Synth., 2007, 4, 370–380 Search PubMed; P. J. Dyson and T. J. Geldbach, Electrochem. Soc. Interface, 2007, 16, 50–53 Search PubMed; T. Welton, Coord. Chem. Rev., 2004, 248, 2459–2477 CrossRef CAS.
- S. Keskin, D. Kayrak-Talay, U. Akman and O. Hortacsu, J. Supercrit. Fluids, 2007, 43, 150–180 CrossRef CAS.
- J. E. Bara, E. S. Hatakeyama, D. L. Gin and R. D. Noble, Polym. Adv. Technol., 2008, 19, 1415–1420 CrossRef CAS; M. J. Muldoon, S. N. V. Aki, J. L. Anderson, J. K. Dixon and J. F. Brennecke, J. Phys. Chem. B, 2007, 111, 9001–9009 CrossRef CAS; J. Huang, A. Riisager, R. W. Berg and R. Fehrmann, J. Mol. Catal., A, 2008, 279, 170–176 CrossRef CAS.
- A. Yokozeki and M. B. Shiflett, Appl. Energy, 2007, 84, 351–361 CrossRef CAS.
- D. J. Tempel, P. B. Henderson, J. R. Brzozowski, R. M. Pearlstein and H. S. Cheng, J. Am. Chem. Soc., 2008, 130, 400–401 CrossRef CAS.
|
This journal is © The Royal Society of Chemistry 2009 |