DOI:
10.1039/B903941D
(Perspective)
Energy Environ. Sci., 2009,
2, 759-766
Improving biodiesel fuel properties by modifying fatty ester composition†
Received
25th February 2009
, Accepted 14th April 2009
First published on 23rd April 2009
Abstract
Biodiesel is an alternative to petroleum-derived diesel fuel composed of alkyl esters of vegetable oils, animal fats or other feedstocks such as used cooking oils. The fatty acid profile of biodiesel corresponds to that of its feedstock. Most common feedstocks possess fatty acid profiles consisting mainly of five C16 and C18 fatty acids, namely, palmitic (hexadecanoic), stearic (octadecanoic), oleic (9(Z)-octadecenoic), linoleic (9(Z),12(Z)-octadecadienoic) and linolenic (9(Z),12(Z),15(Z)-octadecatrienoic) acids, with the exception of a few oils such as coconut oil, which contains high amounts of saturated acids in the C12–C16 range or others. While in many respects biodiesel possesses advantages or is competitive with petroleum-derived diesel fuel, virtually all biodiesel fuels, typically the methyl esters, produced from these oils have performance problems such as poor low-temperature properties or insufficient oxidative stability. Considerable research has focused on solving or alleviating these problems and five approaches have been developed. Besides the approach of using additives, changing the fatty ester composition by either varying the alcohol or the fatty acid profile of the oil have been studied. Changing the fatty acid profile can be achieved by physical means, genetic modification of the feedstock or use of alternative feedstocks with different fatty acid profiles. In some cases approaches may overlap. This article briefly summarizes the five approaches currently used with an emphasis on those dealing with changing the fatty ester composition of a biodiesel fuel.
Gerhard Knothe | Gerhard Knothe obtained his PhD in organic polymer chemistry at the University of Bremen, (West) Germany, in 1988. After a brief postdoctoral appointment, he has been affiliated with the National Center for Agricultural Utilization Research of the U.S. Department of Agriculture in Peoria, IL, since 1989. He is the Lead Scientist on a research project concerned with alternative fuels derived from vegetable oils. |
Broader context
Most biodiesel fuels are derived from feedstocks with fatty acid profiles comprised of five major species. As a result, the resulting biodiesel fuels exhibit the same technical problems, mainly poor cold flow and/or oxidative stability. Modifying the fatty ester composition is a potentially promising route to improving biodiesel fuel properties by enriching it with components possessing more favorable properties. Several approaches to proceed along this route exist and they are summarized in this article. Other issues that affect biodiesel are broadening the supply base of potential biodiesel feedstocks, improving economics of biodiesel production including the feedstock, and the relevance of the foodvs.fuel issue.
|
Introduction
Biodiesel is an alternative diesel fuel obtained by transesterifying vegetable oils or other feedstocks largely comprised of triacylglycerols, including animal fats or used frying oils, with monohydric alcohols to give the corresponding mono-alkyl esters.1,2Methanol, yielding methyl esters of vegetable oils or of other feedstocks, is the most commonly used alcohol in biodiesel production. Important features of biodiesel include reduction in comparison to petroleum-derived diesel fuel (petrodiesel) of most regulated exhaust emissions with the exception of nitrogen oxides, biodegradability, lack of sulfur, inherent lubricity, positive energy balance, higher flash point, compatibility with the existing fuel distribution infrastructure, renewability and domestic origin. The development of biodiesel standards has accompanied the increasing interest in, production and use of biodiesel. Two biodiesel standards that have served in the development of other standards around the world are the standards ASTM D67513 in the United States and EN 142144 in Europe.
Important properties that affect the suitability of any material as diesel fuel are cetane number, viscosity, cold flow, oxidative stability and lubricity. These properties, with lubricity forming an exception to some extent, are largely determined by the fatty ester composition of the biodiesel fuel. Again with the exception of lubricity, which is addressed only in petrodiesel standards, these properties are specified in biodiesel standards. The corresponding specifications are given in Table 1.
Table 1 Specifications in biodiesel standards affecting the fuel properties of alkyl estersa
Specification |
Standard |
ASTM D6751 (United States) |
EN 14214 (Europe) |
Test method |
Limit |
Test method |
Limit |
ASTM = American Society for Testing and Materials; ISO = International Standards Organization.
|
Cetane number |
ASTM D613/D6890 |
47 minimum |
EN ISO 5165 |
51 minimum |
Kinematic viscosity |
ASTM D445 |
1.9–6.0 mm2 s−1 |
EN ISO 3104 |
3.5–5.0 mm2 s−1 |
Oxidative stability |
EN 14112 |
3 h minimum |
EN 14112 |
6 h minimum |
Cloud point |
ASTM D2500 |
Report |
— |
— |
Cold filter plugging point |
— |
— |
EN 116 |
Depending on time of year and location |
Cold soak |
Annex to D6751 |
Filtration time (s) to be reported |
— |
— |
Several technical performance problems related to some of these properties have impaired the use and commercialization of biodiesel. While the issue of increased NOx exhaust emissions that has beset biodiesel may diminish with time due to the increasing market penetration of new exhaust emissions control technologies, two major problems to be resolved are poor low-temperature properties and poor oxidative stability. These problems result from the physical and chemical properties of fatty esters, the major components of biodiesel. They are also influenced, however, by minor fuel constituents arising during the transesterification reaction or carried over from the feedstock.
Fatty acid esters are composed of two building blocks, the fatty acid chain and the alcohol. Therefore, both these moieties in fatty esters influence fuel properties and varying one or both of them can lead to a change in fuel properties. For biodiesel, this issue is rendered more complicated because its fatty ester composition reflects the fatty acid profile of the feedstock used for its production Thus the properties of all the individual fatty acid esters influence the properties of the aggregate that is biodiesel. Most common biodiesel feedstocks such as commodity vegetable oils (soybean, palm, rapeseed/canola), animal fats or used cooking oils possess fatty acid profiles consisting mainly of the five common C16 and C18 fatty acids, palmitic (hexadecanoic, C16:0), stearic (octadecanoic, C18:0), oleic (9(Z)-octadecenoic, C18:1), linoleic (9(Z),12(Z)-octadecatrienoic, C18:2) and linolenic (9(Z),12(Z),15(Z)-octadecadienoic, C18:3). This means that any biodiesel fuel derived from these feedstocks will face the problems of poor cold flow properties or insufficient oxidative stability or, in most cases, both.
Fig. 1 presents an overview of the various approaches that have been explored for improving the fuel-related properties of biodiesel. Instead of changing the fatty ester composition, the use of additives designed to influence specific fuel properties is probably the simplest approach. The second set of approaches, for which several paths exist as shown in Fig. 1, is to vary the fatty ester composition. As mentioned above, in this case the alcohol and/or fatty acids may be changed. Modifying the fatty acid profile can be accomplished by physical means such as fractionation after the biodiesel has been produced or modifying the fatty acid profile prior to biodiesel production. The latter can entail either the use of alternative feedstocks with fatty acid profiles differing from the common C16–C18 fatty acids or using oils, including commodity oils, whose fatty acid profile has been optimized to specifically improve fuel properties. This article presents an overview and brief summary of these approaches.
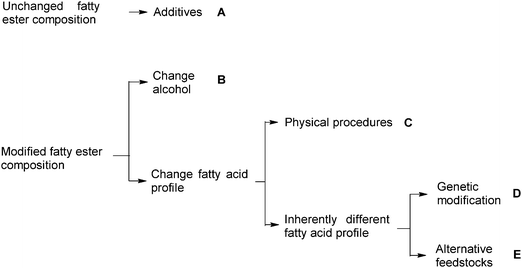 |
| Fig. 1 Five approaches to improving biodiesel fuel properties. | |
Discussion
A total of five approaches designated with the letters A to E in Fig. 1 can be currently distinguished. This article emphasizes the approaches which improve biodiesel fuel properties through inherent changes to the fatty ester composition, i.e., using feedstocks with modified fatty acid profile (D and E in Fig. 1) or varying the alcohol component (B in Fig. 1). Important fuel properties determined by fatty esters and included in biodiesel standards (Table 1) are discussed briefly first. Table 2 lists properties of some fatty esters essential to fuel use.
Table 2 Properties of some fatty acid methyl and ethyl esters.a
The cetane number is a dimensionless descriptor related to the ignition delay time a fuel experiences upon injection into the combustion chamber of a diesel engine. The higher the cetane number, the shorter the ignition delay time and vice versa. A cetane scale using hydrocarbons has been established and is commonly used with hexadecane being the high CN reference compound on this scale (CN = 100). The CN is included in biodiesel standards with prescribed minimum values (Table 1). The CN of fatty esters increases with increasing saturation and increasing chain length.5,6 Thus, the CN of esters of palmitic and stearic acids is >80 (Table 2),6 the CN of methyl oleate is in the range of 55–58, that of esters of linoleic acid around 40 and that of esters of linolenic acid around 25. Increasing CN to a certain level (around 60) has been brought into connection with decreasing NOx exhaust emissions.7 As indicated by the effect of CN on NOx exhaust emissions, chain length and saturation levels affect regulated exhaust emissions.8,9 Thus NOx exhaust emissions are reduced for saturated esters in comparison to unsaturated esters but particulate matter seemed to be affected less. Hydrocarbons and CO exhaust emissions decrease with increasing chain length.
Viscosity
Reducing the high viscosity of materials such as vegetable oils containing triacylglycerols is the major reason for producing alkyl esters from such oils. The high viscosity of untransesterified vegetable oils leads to operational problems in a diesel engine, for example increased engine deposits. Viscosity, in the form of kinematic viscosity, is specified in biodiesel standards. Since viscosity increases with decreasing temperature, handling of fuels at lower temperatures is facilitated by lower viscosity. In form of kinematic viscosity, this property is contained in biodiesel standards with minimum and maximum values (Table 1), although the minimum value for kinematic viscosity in the European standard exceeds that of most petrodiesel fuels, which does not appear technically justified. The viscosity of a fatty ester increases with increasing chain length and increasing saturation.10 However, only cis double bonds cause a noticeable reduction of viscosity as esters with trans double bonds display viscosity similar to their saturated counterparts.10
Oxidative stability
Biodiesel, like the vegetable oils it is derived from, can react with the oxygen in air.11 The reason for this autoxidation reaction is the presence of double bonds in the chains of many fatty compounds. The autoxidation of unsaturated fatty compounds proceeds with different rates depending on the number and position of double bonds. The CH2 positions allylic to double bonds in the fatty acid chains are those susceptible to oxidation. The bis-allylic positions in polyunsaturated fatty acids, such as linoleic acid (double bonds at C-9 and C-12, giving one bis-allylic position at C-11) and linolenic acid (double bonds at C-9, C-12, and C-15, giving two bis-allylic positions at C-11 and C-14), are even more prone to autoxidation than allylic positions. Relative rates of oxidation given in the literature [11 and references therein] are 1 for oleates (methyl, ethyl esters), 41 for linoleates, and 98 for linolenates. This is essential because most biodiesel fuels contain significant amounts of esters of oleic, linoleic or linolenic acids, which influence the oxidative stability of the fuels. The species formed during the oxidation process cause the fuel to eventually deteriorate. The oxidation of fatty acid chains is promoted by factors such as presence of air, light, elevated temperatures and presence of extraneous materials.
An issue to consider in the case of the European biodiesel standard EN 14214 is the iodine value (IV), which is a crude measure of the total unsaturation of a sample. The IV has often been used in connection with issues such as oxidative stability, with the implication that materials with a high IV are less oxidative stable than those with a low IV. While neat compounds have a well-defined IV, an infinite number of mixtures of compounds with different IV can afford the IV of a sample such as biodiesel. The IV is also molecular weight dependent, for example, ethyl esters have a lower IV than methyl esters although the kind of ester is highly unlikely to affect oxidative stability of the double bonds in a fatty acid chain. Therefore, the IV should not be considered in the choice of optimized biodiesel components.12
Cold flow
Since mixtures such as biodiesel do not possess melting points, but rather melting ranges, this is reflected in the specifications used in biodiesel standards. The cloud point is the temperature at which the first solids appear in a fuel but the fuel can still flow, although these solids can lead to fuel filter plugging.13 The pour point, usually a few degrees below the cloud point, is the temperature at which the fuel can no longer be freely poured. Several other methods exist for determining the low-temperature properties of biodiesel. These are the cold filter plugging point (CFPP) and low-temperature flow test (LTFT).13 The cloud point and CFPP are included in biodiesel standards but are “soft” specifications, the CP in ASTM D6751 requiring a report and the CFPP in EN 14214 varying with time of year and geographic location. The low-temperature properties of biodiesel are again influenced by the properties of the individual components. The melting points of fatty esters generally increase with chain length (although chains with odd numbers of carbons have slightly lower melting points that the preceding even-number chain) and increasing saturation.
Lubricity
The advent of low-sulfur petrodiesel fuels and, more recently, ultra-low sulfur diesel fuels (ULSD) as required by regulations in the United States, Europe and elsewhere, has led to failure of engine parts such as fuel injectors and pumps as they are lubricated by the fuel itself. The poor lubricity of low-sulfur petrodiesel fuels requires additization or blending with another fuel of sufficient lubricity to regain lubricity.14 The reason for the poor lubricity of low-sulfur diesel fuels apparently is not the removal of the sulfur-containing compounds but rather that other polar compounds with heteroatoms such as oxygen and nitrogen are also reduced in (ultra-)low sulfur diesel fuels,15 causing a reduction of lubricity. Biodiesel restores lubricity to such low-lubricity diesel fuels at blend levels of about 2% and higher [16 and references therein].
Biodiesel properties
The properties of biodiesel resulting from those of the major ester components can be illustrated by those of biodiesel fuels derived from some common feedstocks. Soybean methyl esters (fatty acid profile approximately 11% C16:0, 4% C18:0, 21–24% C18:1, 49–53% C18:2, 7–8% C18:3) display cetane numbers around 48–52 with kinematic viscosity (40 °C) in the range 4.10–4.15 mm2 s−1 and a cloud point of approximately 0 °C [1,2 and references therein]. Rapeseed/canola methyl esters (fatty acid profile approximately 4% C16:0, 2% C18:0, 58–62% C18:1, 21–24% C18:2, 10–11% C18:3) possesses a CN in the range of 51–55, kinematic viscosity (40 °C) around 4.5 mm2 s−1, and a CP of approximately −3 °C [1,2 and references therein]. Thus the difference in fatty acid profile, in this case approximately a reversal of the C18:1 and C18:2 content, causes a noticeable change in fuel properties. Palm oil contains about 40–45% palmitic acid in its fatty acid profile and thus the derived biodiesel has a high CP (>10 °C).
Minor constituents
Minor constituents can have significant effects on biodiesel properties as briefly summarized here. For example, mono- and diacylglycerols increased the cloud point of methyl soyate in concentrations as low as 0.1%.17 Especially the monoacylglycerols (MAG) of saturated C16 and C18 fatty acids, possessing melting points >70 °C,18 are problematic in this respect.17Monoolein (melting point of racemic 1-monoolein 35 °C18) in concentrations up to 1% had no effect on CP and PP.17 On the other hand, MAG and free fatty acids possess excellent lubricity and are likely to a large part responsible for the lubricity of low-level blends of biodiesel with petrodiesel.16 However, changed fatty acid profiles may also possess advantages in this respect. For example, monodecanoin in the racemic form has a melting point of 53 °C,18 approximately 20 °C below that of monopalmitin and monostearin, which may have be beneficial for cold flow properties. Besides MAG, recently sterol glucosides (SG), which have high melting points (>240 °C) and limited solubility in biodiesel, have been identified as a cause of precipitates forming when storing biodiesel in the cold or sometimes even at ambient temperature.19–21 Species such as MAG, DAG, TAG, FFA and SG may exist in biodiesel made from all feedstocks, including alternative feedstocks discussed here, and can influence fuel properties. The issue of minor components affecting low-temperature properties is addressed with the new cold soak specification in the biodiesel standard ASTM D6751 (Table 1).
Approaches to improving biodiesel fuel properties. Unchanged fatty ester composition
Additives
The use of additives with biodiesel has been largely of interest to improve cold flow properties and oxidative stability. Some research has involved the use of cetane improvers22 since some connection between reduced NOx exhaust emissions and higher CN exists.7 However, with increasing market penetration of exhaust emissions control technologies such as selective catalytic reduction which have the capability to reduce NOx exhaust emissions to the low levels prescribed in current exhaust emissions standards, the use of cetane improvers for this purpose may be of decreasing interest. Generally, biodiesel does not require cetane-improving additives only for the sake of improving the cetane number because biodiesel usually meets the minimum CN requirements in biodiesel standards. An exception can be soy methyl esters not meeting the minimum CN of 51 required in EN 14214 but soy methyl esters generally meet the minimum CN of 47 prescribed in ASTM D6751.
Regarding oxidative stability, a variety of commercial antioxidants, for example BHT (butylated hydroxytoluene), TBHQ (tert.-butylhydroquinone), BHA (butylated hydroxyanisole), PG (pyrogallol) and others have been studied and shown to be largely effective.23 Most vegetable oils contain naturally occurring antioxidants (tocopherols) but their presence in biodiesel is reduced as a result of the transesterification reaction. Adding such naturally occurring antioxidants to biodiesel has therefore also been studied23 but overall they appear to be less effective than synthetic antioxidants. As cold flow additives, a variety of polymers24 have been reported. Potential problems with the additive approach include those of additive compatibility and unintended effects on other fuel properties. For example, some diesel particulate filter additives were shown to have a negative influence on biodiesel oxidative stability and may reduce the effectiveness of some antioxidants.25
Modified fatty ester composition
Alcohol
Methanol has been the most employed alcohol in biodiesel production. As a result, although biodiesel is defined generally as fatty acid alkyl esters (FAAE), in many cases fatty acid methyl esters (FAME) have been used virtually synonymously as term for biodiesel. However, FAME is a narrow approach to biodiesel. The major reason for this approach is that methanol is the least expensive alcohol in most countries around the world. Brazil constitutes an exception since the large production of ethanol from sugarcane has caused availability of relatively inexpensive ethanol.
Besides ethanol, other alcohols have been studied for biodiesel production, with iso-propanol being the most one most commonly investigated. Literature data discussed briefly here show that ethyl esters and especially iso-propyl esters improve low-temperature properties of biodiesel compared to methyl esters. Isopropyl and isobutyl esters of common soybean oil exhibited crystallization temperatures 7–11 and 12–14 °C lower than the corresponding methyl esters.26Isopropyl esters of lard and tallow showed a crystallization onset temperature (TCO) similar to methyl soyate.26 Benefits of branched-chain esters increased when they were blended with petrodiesel.26 Similarly, ethyl esters of tallow and grease as well as isopropyl esters of tallow showed improved low-temperature properties.27 For a homologous series of C1–C4ester moieties for tallow, ethyl esters showed the overall best low-temperature properties.28 However, branched esters did not impart any advantages to blends with petrodiesel at the 3–5% blend level when compared to methyl or ethyl esters.29Isopropyl esters of soybean oil and yellow grease displayed exhaust emissions benefits similar to those of the corresponding ethyl esters30 with CO and hydrocarbon emissions being slightly higher compared to methyl esters.
Physical procedures.
Modifying the fatty ester composition of a biodiesel fuel can be accomplished after production by means of a fractionation (“winterization”) process.31–35 Such a process is based on the differences in melting points of the various fatty acid chains. The objective is to remove biodiesel components with higher melting points, for example methyl stearate and methyl palmitate from vegetable oil methyl esters, as they are the species responsible for the relatively high cloud points of biodiesel. The process entails a series of cooling cycles for the fuel although microprocess engineering has also been used. The biodiesel fuel is cooled sufficiently to cause higher melting components to solidify. These solid particles are then removed (for example, by filtration), the fuel is allowed to warm again and then the cooling process with subsequent removal of solids is repeated. Fractionation of biodiesel has also been carried out in the presence of cold flow improving additives or solvents such as hexane31,32 and isopropanol,32 enhancing yield and reducing the number of cooling cycles.31,32 With each cycle, the cold flow properties of the biodiesel fuel improve. The fractionation possesses some significant disadvantages inherent to the removal of the saturated fatty acid chains. These include reduction of the cetane number and related effects on combustion and poorer oxidative stability because saturated fatty esters possess higher CN and better oxidative stability than their unsaturated counterparts (see data in Table 2).
Inherently different fatty acid profile.
As mentioned above, most feedstocks used for biodiesel possess fatty acid profiles with varying amounts of the five most common C16–C18 fatty acids. As a result, most biodiesel fuels derived from these feedstocks exhibit cold flow and/or oxidative stability problems. In order to address these problems inherently, i.e., through the composition of the feedstock, two approaches are possible (D and E in Fig. 1), namely, deliberately changing the composition of classical feedstocks or using feedstocks with inherently different fatty acid profiles. In some cases, the two approaches overlap, for example, microalgae may be modified to not only enhance oil production but also to yield an improved fatty acid profile.36
Using thermal efficiency data, it was originally suggested37 that ethyl esters of monounsaturated or short-chain saturated fatty acids should make good alternative fuels and that they should be evaluated in long-term engine tests In recent work,38 it was shown that, while methyl oleate is the most desirable fatty acid among the five common ones to enrich in a feedstock, other acids may possess properties that could be even more advantageous. For example, the melting point of methyl palmitoleate is approximately −34 °C,38 about 14 °C lower than that of methyl oleate, a significant advantage with regards to cold flow properties, while the oxidative stability of these compounds is comparable. If saturated fatty acids are preferred due to their greater oxidative stability, esters of decanoic acid appear advantageous because they offer a compromise between cold flow and cetane number.
Genetic modification.
Genetic modification is an approach that has usually been applied to commodity oils, i.e., oils with sufficient commercial volume, to render them more amenable to specific purposes. Enhancement of oleic acid in the fatty acid profile has been the most common approach to find a compromise between the issue of oxidative stability and cold flow without reducing the CN to an unacceptably low level. An interesting approach in this connection is to take into consideration that commodity vegetable oils are used primarily for physiological, i.e., nutritional, purposes.39Unsaturated fatty acids, especially linoleic acid, possess favorable organoleptic properties, being the source of fried food flavor compounds, leading to the necessity of a balance between oleic and linoleic acid in an oil suitable for both physiological applications as well as biodiesel feedstock.39 Such an oil could contain about 71% oleic acid, 21% linoleic acid 2% linolenic acid and 3% saturates (C16:0 and C18:0). Another example is esters of low-palmitic (3.8% instead of the usual 10%) soybean oil (and esters of typical SME diluted with hexane) giving winterized biodiesel with low-temperature properties similar to those of iso-propyl esters.26
It has also been suggested to enrich ricinoleic acid (12-hydroxy-9(Z)-octadecenoic acid) together with oleic acid in soybean oil, in order to provide even better lubricity than is usually observed with soybean oil-derived biodiesel while preserving oxidative stability.40 However, methyl ricinoleate, while indeed possessing excellent lubricity,16,41 has high viscosity, low oxidative stability and a low cetane number,38 so that overall it appears to be a less desirable component to enhance in a biodiesel feedstock.
Generally, little other work has been performed on biodiesel obtained from genetically modified oils. This observation can likely be traced to the time it takes to develop such feedstocks and to reservations related to physiological applications. Therefore, besides addressing the supply issue, alternative feedstocks with inherently different fatty acid profiles can serve as models for biodiesel sources with different fatty acid profiles and possibly improved fuel properties. This approach is discussed below.
Alternative feedstocks.
The supply of commodity oils is not sufficient to produce enough biodiesel to fully replace all petroleum-based diesel fuel. Concern has also been voiced over the use of edible oils for fuel production as well as over the carbon cycle.42 Such issues have caused a search for additional feedstocks. Accordingly, inedible vegetable oils have generated significant interest, most notably jatropha oil.43–47 Other oils for which reports exist of the biodiesel fuel derived from them include camelina,48 moringa49 karanja,50 and rubberseed.51 Used cooking or frying oils and animal fats such as tallow are also of interest and biodiesel from these feedstocks is being produced commercially.52,53 However, most of these feedstocks have fatty acid profiles in the typical C16–C18 range. For example, jatropha contains approximately 21–22% saturated fatty acids (virtually all palmitic and stearic) with the oleic and linoleic acids comprising the most of the remaining profile,43 leading to a biodiesel fuel that likely possesses a CP well >0 °C. Thus they may provide additional sources of feedstock but face the same technical issues as more traditional feedstocks.
A vegetable oil with relatively large production numbers but a somewhat different fatty acid profile is coconut oil as it contains in the range of 45–50% lauric acid and 16–20% myristic acid with other saturated fatty acids comprising the majority of the remaining fatty acid profile and small amounts of unsaturated fatty acids. As a result biodiesel derived from coconut oil has poor cold flow properties, with its ethyl esters possessing a cloud point reportedly around 5 °C,54 although such a fatty acid profile, oxidative stability and cetane number will exceed that of the C16–C18 commodity oils. However, greater amounts of a saturated medium chain, approximately 65% decanoic acid, supplemented by about 12% oleic acid and 7% linoleic acid with C8–C18saturated fatty acids accounting for the rest of the fatty acid profile, in cuphea oil resulted in a cloud point of −9 to −10 °C for the methyl esters.55 The cetane number of cuphea methyl esters is 55–56 and the oxidative stability slightly exceeded the 3 h specification in ASTM D6751 standard but not the 6 h in EN 14214. Although cuphea oil has not been commercialized, the results can serve as model for other potential feedstocks with high C10fatty acid content and a resulting biodiesel fuel with overall improved fuel properties. Research up to the year 2001 regarding genetic engineering of feedstocks to yield higher levels of these medium chain length acids has been summarized.56
Oleaginous algae have attracted considerable interest as biodiesel feedstock because of their greater supply potential, and the idea of using them as a potential fuel source was first suggested in the late 1970’s and early 1980’s [57, 58 and references therein]. More than 40,000 species have been identified, these can be classified into several groups such as cyanobacteria, diatoms, dinoflagellates, pico-plankton, as well as green, yellow-green, golden, red, and brown algae.58 The lipids that are produced by algae include not only the desired TAG but also materials found in conventional vegetable oils such as wax esters, sterols, tocopherols and others.58 It may be noted that algae can be also a potential source of hydrocarbons as the example of Botryococcus braunii show.59 However, this feedstock has been beset by problems of its own, mainly related to production, such as isolation and harvest of the algae and extraction of the oil and overall economics.57,60 Work regarding genetic engineering to enhance lipid production in algae has been reported.36,61 An overview of the fatty acid profiles from lipids of seventeen algal species58 shows significant variety but most species contain monounsaturated fatty acids (mainly C16:1 and C18:1), significant amounts of polyunsaturated fatty acids (a feature observed in other marine oils such as fish oils) and/or saturated fatty acid chains, especially C14 and C16. The fatty acid profile produced by or obtained from algae is influenced by a variety of factors including nutrients provided, temperature, light intensity, growth phase, culture age, and environmental stress.58 Generally, lower temperatures and low light conditions, i.e., reduced energy input, appear to favor unsaturation in fatty acid chains while higher temperatures and high light conditions, i.e., exposure to energy sources, lead to increasing saturation. As mentioned above, even small amounts of more highly unsaturated fatty acids may have a greater influence on oxidative stability than these small amounts would indicate. On the other hand, the saturated fatty acid chains cause cold flow problems. Thus, biodiesel produced from the oils of algal species may face both problems, cold flow and oxidative stability, at the same time, just as is the case with biodiesel obtained from traditional feedstocks.
Interesting approaches are those that do not use classical lipid feedstocks for producing fatty esters. An approach that in some respects combines both the D and E approaches utilizes a variety of microorganisms62,63 which have been genetically engineered so that their metabolism has been altered. As a result of this genetic engineering, during a fermentation process the microorganisms can use non-lipid carbon sources, for example carbohydrates or, more generally, biomass to produce fatty esters. The number of carbon atoms used to produce the fatty acid chains is a major cost factor.63 For example, since the oxygen in oxygenated feedstocks such as carbohydrates must be released in form of CO2, a maximum metabolic efficiency of about 34% can be achieved. No specific fatty acid profile that can be achieved is given as this appears to be one of the variables that can be deliberately altered.63 One of the advantages of this approach is that some minor constituents of biodiesel that can influence fuel properties do not occur or occur only in very small amounts in these fermentation-derived biodiesel fuels.
Metabolically engineered Escherichia coli bacteria produced fatty acid ethyl esters (FAEE) termed “microdiesel”.62 The analysis of the FAEE in a medium supplemented with sodium oleate gave a mixture consisting mainly of ethyl oleate with minor amounts of ethyl palmitate and ethyl palmitoleate, indicating that some fatty acids from de novofatty acid biosynthesis were channeled to produce FAEE. With technical grade sodium oleate, ethyl esters corresponding to the other fatty acids in the technical sodium oleate were observed. The sodium oleate was converted to FAEE with an efficiency of 62–67% on a molar basis with a 26% cellular FAEE content. A disadvantage was, however, that substantial FAEE production depended on a supply of exogenous sodium oleate with another problem being the slow reaction rate of the acyltransferase with ethanol.
Two reviews64,65 summarize the work on microbial production of fuels (both biodiesel and petrodiesel-like hydrocarbons).
Conclusions and outlook
Five approaches currently exist for improving the fuel properties of biodiesel. Each approach has certain advantages and disadvantages, ranging from technical issues as discussed here to production, economics, supply and availability issues. Ideally, a fuel with optimized fatty ester composition could be produced inexpensively from an abundant feedstock but this ideal still seems to be some way down the road. In any case, it appears that biotechnologically-oriented approaches which enhance supply of alternative feedstocks or provide classical commodity oils with fatty acid profiles modified for improving fuel properties will play an increasingly important role. Other considerations such as foodvs.fuel (it may be noted that concerns over using edible oils for non-food purposes have been raised only in connection with fuel use, not in connection with other non-food uses such as lubricants, polymers, etc.) as well as the carbon footprint of growing and harvesting specific feedstocks, including possible carbon sequestration (for example, algae), are likely to affect this issue. For biodiesel to be successful, the issue of glycerol, although not addressed in the present work, is also important. Biotechnological approaches in which esters are produced directly without the need to produce biodiesel from a triacylglycerol oil may circumvent this issue.
Furthermore, fuels (“renewable diesel”) resembling the composition of petroleum-based diesel fuels can be obtained from lipid feedstocks (or other biomass) by means of hydrotreating. Thus, a possible competition may arise between biodiesel and renewable diesel (often called “green diesel” but this term is misleading since it implies that this petrodiesel-like fuel itself is “greener”, i.e., more environmentally friendly, than petrodiesel, which is not necessarily the case). On the other hand, biodiesel and petrodiesel may be used according to their properties, biodiesel where environmental issues play a more significant role, renewable diesel where cold flow is a major issue (for example, aviation). While it is improbable that bio-based fuels will be able to fully replace all petroleum-based diesel or diesel-like fuels in the near future, continuing research and other progress will likely further advance their production and use.
References
-
The Biodiesel Handbook, ed.G. Knothe, J. Van Gerpen and J. Krahl, AOCS Press, Champaign, IL 2005 Search PubMed.
-
M. Mittelbach and C. Remschmidt, Biodiesel - The Comprehensive HandbookM. Mittelbach, Graz, Austria, 2004 Search PubMed.
-
American Society for Testing and Materials (ASTM), D6751 Standard Specification for biodiesel fuel blend stock (B100) for middle distillate fuels. ASTM, West Conshohocken, PA, 2008 Search PubMed.
-
European Committee for Standardization (CEN), Standard EN 14214, Automotive fuels - fatty acid methyl esters (FAME) for diesel engines - Requirements and test methods, CEN, Brussels, 2003 Search PubMed.
- K. J. Harrington, Chemical and Physical Properties of Vegetable Oil Esters and Their Effect on Diesel Fuel Performance, Biomass, 1986,(9), 1–17 CrossRef CAS.
- G. Knothe, A. C. Matheaus and T. W. Ryan III, Cetane Numbers of Branched and Straight-Chain Fatty Esters Determined in an Ignition Quality Tester, Fuel, 2003, 82, 971–97 CrossRef CAS.
- N. Ladommatos, M. Parsi and A. Knowles, The effect of fuel cetane improver on diesel pollutant emissions, Fuel, 1996, 75, 8–14 CrossRef CAS.
- R. L. McCormick, M. S. Graboski, T. L. Alleman and A. M. Herring, Impact of biodiesel source material and chemical structure on emissions of criteria pollutants from a heavy-duty engine, Environ. Sci. Technol., 2001, 35, 1742–1747 CrossRef CAS.
- G. Knothe, C. A. Sharp and T. W. Ryan III, Exhaust Emissions of Biodiesel, Petrodiesel, Neat Methyl Esters, and Alkanes in a New Technology Engine, Energy Fuels, 2006, 20, 403–408 CrossRef CAS.
- G. Knothe and K. R. Steidley, Kinematic Viscosity of Biodiesel Fuel Components. Influence of Compound Structure and Comparison to Petrodiesel Fuel Components, Fuel, 2005, 84, 1059–1065 CrossRef CAS.
-
E. N. Frankel, Lipid Oxidation, The Oily Press, PJ Barnes & Associates, Bridgwater, England, 2nd edn, 2005 Search PubMed.
- G. Knothe, Structure Indices in FA Chemistry. How Relevant Is the Iodine Value?, J. Am. Oil Chem. Soc., 2002, 79, 847–854 CrossRef CAS.
- R. O. Dunn and M. O. Bagby, Low-Temperature Properties of Triglyceride-Based Diesel Fuels: Transesterified Methyl Esters and Petroleum Middle Distillate/Ester Blends, J. Am. Oil Chem. Soc., 1995, 72, 895–904 CrossRef CAS.
-
P. I. Lacey and S. R. Westbrook, Diesel Fuel Lubricity, SAE Technical Paper Series 950248, 1995.
-
R. H. Barbour, D. J. Rickeard and N. G. Elliott, Understanding Diesel Lubricity, SAE Technical Paper Series 2000-01-1918, 2000.
- G. Knothe and K. R. Steidley, Lubricity of Components of Biodiesel and Petrodiesel. The Origin of Biodiesel Lubricity, Energy Fuels, 2005, 19, 1192–1200 CrossRef CAS.
- L. Yu, I. Lee, E. G. Hammond, L. A. Johnson and J. H. Van Gerpen, The Influence of Trace Components on the Melting Point of Methyl Soyate, J. Am. Oil Chem. Soc., 1998, 75, 1821–1824 CrossRef CAS.
-
F. D. Gunstone, J. L. Harwood, A. J. Dijkstra, The Lipid Handbook, CRC Press, Boca Raton FL, Dictionary Section, 3rd edn, 2007 Search PubMed.
- P. Bondioli, N. Cortesi and C. Mariani, Identification and Quantification of Steryl Glucosides in Biodiesel, Eur. J. Lipid Sci. Technol., 2008, 110, 120–126 CrossRef CAS.
- R. A. Moreau, K. M. Scott and M. J. Haas, The Identification of Steryl Glucosides in Precipitates from Commercial Biodiesel, J. Am. Oil Chem. Soc., 2008, 85, 761–770 CrossRef CAS.
- V. Van Hoed, N. Zyaykina, W. De Greyt, J. Maes, R. Verhé and K. Demeestere, Identification and Occurrence of Steryl Glucosides in Palm and Soy Biodiesel, J. Am. Oil Chem. Soc., 2008, 85, 701–709 CrossRef CAS.
-
G. Knothe, M. O. Bagby and T. W. Ryan, III, Cetane Numbers of Fatty Compounds: Influence of Compound Structure and of Various Potential Cetane Improvers, SAE Technical Paper Series No. 97168, 1997.
- R. O. Dunn, Antioxidants for Improving Storage Stability of Biodiesel, Biofuels, Bioprod. Bioref., 2008, 2, 304–318 Search PubMed.
- G. Knothe, R. O. Dunn and M. O. Bagby, Biodiesel: The Use of Vegetable Oils and Their Derivatives as Alternative Diesel Fuels, ACS Symp. Ser., 1997, 666, 172–208 CAS (Fuels and Chemicals from Biomass).
- S. Schober and M. Mittelbach, Influence of Diesel Particulate Filter Additives on Biodiesel Quality, Eur. J. Lipid Sci. Technol., 2005, 107, 268–271 CrossRef CAS.
- I. Lee, L. A. Johnson and E. G. Hammond, Use of branched-chain esters to reduce the crystallization temperature of biodiesel, J. Am. Oil Chem. Soc., 1995, 72, 1155–1160 CrossRef CAS.
- W.-H. Wu, T. A. Foglia, W. N. Marmer, R. O. Dunn, C. E. Goering and T. E. Briggs, Low-Temperature Property and Engine Performance Evaluation of Ethyl and Isopropyl Esters of Tallow and Grease, J. Am. Oil Chem. Soc., 1998, 75, 1173–1178 CAS.
- T. A. Foglia, L. A. Nelson, R. O. Dunn and W. N. Marmer, Low-Temperature Properties of Alkyl Esters of Tallow and Grease, J. Am. Oil Chem. Soc., 1997, 74, 951–955 CrossRef CAS.
- A. Kleinová, J. Paligová, M. Vrbová, J. Mikulec and J. Cvengroš, Cold Flow Properties of Fatty Esters, Trans. IChemE, 2007, 85(B5), 390–395 Search PubMed.
- P. S. Wang, M. E. Tat and J. Van Gerpen, The production of fatty acid isopropyl esters and their use as a diesel engine fuel, J. Am. Oil Chem. Soc., 2005, 82, 845–849 CrossRef CAS.
- I. Lee, L. A. Johnson and E. G. Hammond, Reducing the crystallization temperature of biodiesel by winterizing methyl soyate, J. Am. Oil Chem. Soc., 1996, 73, 631–636 CrossRef CAS.
-
R. O. Dunn, M. W. Shockley and M. O. Bagby, Winterized methyl esters from soybean oil: An alternative diesel fuel with improved low temperature flow properties, SAE Technical Paper Ser. 971682, 1997.
- M. E. González Gómez, R. Howard-Hildige, J. J. Leahy and B. Rice, Winterisation of Waste Cooking Oil Methyl Ester to Improve Cold Temperature Fuel Properties, Fuel, 2002, 81, 33–39 CrossRef CAS.
- T. Sahlabji, H. Wichmann, H. Dieckmann, P. Jopke and M. Bahadir, Chemical Aspects of Fractional Crystallization of Fatty Acid Methyl Esters Produced from Waste Fats, Clean, 2007, 35, 323–328 Search PubMed.
- S. Kerschbaum, G. Rinke and K. Schubert, Winterization of Biodiesel by Micro Process Engineering, Fuel, 2008, 87, 2590–2597 CrossRef CAS.
- P. G. Roessler, L. M. Brown, T. G. Dunahay, D. A. Heacox, E. E. Jarvis, J. C. Schneider, S. G. Talbot and K. G. Zeiler, Genetic Engineering Approaches for Enhanced Production of Biodiesel Fuel from Microalgae, ACS Symp. Ser., 1994, 566, 255–270 CAS (Enzymatic Conversion of Biomass for Fuels Production).
- W. E. Klopfenstein and H. S. Walker, Efficiencies of Various Esters of Fatty Acids as Diesel Fuels, J. Am. Oil Chem. Soc., 1983, 60, 1596–1598 CrossRef CAS.
- G. Knothe, “Designer” Biodiesel: Optimizing Fatty Ester Composition to Improve Fuel Properties, Energy Fuels, 2008, 22, 1358–1364 CrossRef CAS.
-
N. A. Bringe, Soybean oil composition for biodiesel in The Biodiesel Handbook, ed. G. Knothe , J. Van Gerpen, J. Krahl, AOCS Press: Champaign, IL, 2005; pp 161–164 Search PubMed.
- A. J. Kinney and T. E. Clemente, Modifying soybean oil for enhanced performance in biodiesel blends, Fuel Process. Technol., 2005, 86, 1137–1147 CrossRef CAS.
- J. W. Goodrum and D. P. Geller, Influence of Fatty Acid Methyl Esters from Hydroxylated Vegetable Oils on Diesel Fuel Lubricity, Bioresour. Technol., 2005, 96, 851–855 CrossRef CAS.
-
R. E. Horne, N. D. Mortimer, M. A. Elsayed, Energy and Carbon Balances of Biofuels Production: Biodiesel and Bioethanol, The International Fertiliser Society, Proc. 510, 2003 Search PubMed.
- N. Foidl, G. Foidl, M. Sanchez, M. Mittelbach and S. Hackel,
Jatropha Curcas L. as a Source for the Production of Biofuel in Nicaragua, Bioresour. Technol., 1996, 58, 77–82 CrossRef CAS.
- P. Wood, Out of Africa. Could Jatropha be Europe's Biodiesel Feedstock?, Refocus, 2005, 6, 40–44 CrossRef.
-
S. Mandpe, S. Kadlaskar, W. Degen, S. Keppeler, On Road Testing of Advanced Common Rail Diesel Vehicles with Biodiesel from the Jatropha curcas plant, SAE Technical Paper Ser. 2005-26-356, 2005.
-
I. K. Reksowardojo, I. H. Lubis, W. MangalaS.A., T. P. Brodjonegoro, T. H. Soerawidjaja, W. Arismunandar, N. N. Dung, H. Ogawa, Performance and Exhaust Gas Emissions of Using Biodiesel Fuel from Physic Nut (Jatropha curcas L.) Oil on a Direct Injection Diesel Engine (DI), SAE Technical Paper Ser. 2007-01-2025, 2007.
- K. Prueksakorn and S. H. Gheewala, Full Chain Energy Analysis of Biodiesel from Jatropha curcas L. in Thailand, Environ. Sci. Technol., 2008, 42, 3388–3393 CrossRef CAS.
- A. Fröhlich and B. Rice, Evaluation of Camelina sativa Oil as a Feedstock for Biodiesel Production, Industr. Crops. Prod., 2005, 21, 25–31 Search PubMed.
- U. Rashid, F. Anwar, B. R. Moser and G. Knothe, Moringa oleifera Oil: A Possible Source of Biodiesel, Bioresour. Technol., 2008, 99, 8175–8179 CrossRef CAS.
- B. K. De and D. K. Bhattacharyya, Biodiesel from Minor Vegetable Oils like Karanja Oil and Nahor Oil, Fett/Lipid, 1999, 101, 404–406 CrossRef CAS.
- O. E. Ikwuagwu, I. C. Ononogbu and O. U. Njoku, Production of Biodiesel Using Rubber [Hevea brasiliensis (Kunth Muell.)] Seed Oil, Industr. Crops Prod., 2000, 12, 57–62 Search PubMed.
- Y. Zhang, M. A. Dubé, D. D. McLean and M. Kates, Biodiesel Production from Waste Cooking Oil: 2. Economic Assessment and Sensitivity Analysis, Bioresour. Technol., 2003, 90, 229–240 CrossRef CAS.
- M. G. Kulkarni and A. K. Dalai, Waste Cooking Oil - An Economical Source for Biodiesel: A Review, Ind. Eng. Chem. Res., 2006, 45, 2901–2913 CrossRef CAS.
- C. L. Peterson, J. S. Taberski, J. C. Thompson and C. L. Chase, The Effect of Biodiesel Feedstock on Regulated Emissions in Chassis Dynamometer Tests of a Pickup Truck, Trans. ASAE, 2000, 43, 1371–1381 Search PubMed.
- G. Knothe, S. C. Cermak and R. L. Evangelista, Cuphea Oil as Source of Biodiesel with Improved Fuel Properties Caused by High Content of Methyl Decanoate, Energy Fuels, 2009, 23, 1743–1747 CrossRef CAS.
- K. Dehesh, How can we genetically engineer oilseed crops to produce high levels of medium-chain fatty acids?, Eur. J. Lipid Sci. Technol., 2001, 103, 688–697 CrossRef CAS.
- Y. Chisti, Biodiesel from Microalgae, Biotechnol. Adv., 2007, 25, 294–306 CrossRef CAS.
- Q. Hu, M. Sommerfeld, E. Jarvis, M. Ghirardi, M. Posewitz, M. Seibert and A. Darzins, Microalgal Triacylglycerols as Feedstocks for Biofuel Production: Perspectives and Advances, Plant J., 2008, 54, 621–639 CrossRef CAS.
- A. Banerjee, R. Sharma, Y. Chisti and U. C. Banerjee,
Botryococcus braunii: A Renewable Source of Hydrocarbons and Other Chemicals, Crit. Rev. Biotechnol., 2002, 22, 245–279 CrossRef CAS.
- R. Luque, L. Herrero-Davila, J. M. Campelo, J. H. Clark, J. M. Hidalgo, D. Luna, J. M. Marina and A. A. Romero, Biofuels: a technological perspective, Energy Environ. Sci., 2008, 1, 542–564 RSC.
- T. G. Dunahay, E. E. Jarvis, S. S. Dais and P. G. Roessler, Manipulation of Microalgal Lipid Production Using Genetic Engineering, Appl. Biochem. Biotechnol., 1996, 57–58, 223–231 CrossRef.
- R. Kalscheuer, T. Stölting and A. Steinbüchel, Microdiesel: Escherichia coli engineered for fuel production, Microbiology, 2006, 152, 2529–2536 CrossRef CAS.
-
J. D. Keasling, Z. Hu, C. Somerville, G. Church, D. Berry, L. Friedman, A. Schirmer, S. Brubaker and S. B. del
Cardayré, Production of Fatty Acids and Derivatives Thereof, WO/2007/136762, November 29, 2007. http://www.wipo.int/pctdb/en/wo.jsp?WO%20=%202007136762.
- L. P. Wackett, Biomass to fuels via microbial transformations, Curr. Opin. Chem. Biol., 2008, 12, 187–193 CrossRef CAS.
- T. Stöveken and A. Steinbüchel, Bacterial acyltransferases as an alternative for lipase-catalyzed acylation for the production of oleochemicals and fuels, Angew. Chem., Int. Ed., 2008, 47, 3688–3694 CrossRef.
Footnote |
† Disclaimer: Product names are necessary to report factually on available data; however, the USDA neither guarantees nor warrants the standard of the product, and the use of the name by USDA implies no approval of the product to the exclusion of others that may also be suitable. |
|
This journal is © The Royal Society of Chemistry 2009 |
Click here to see how this site uses Cookies. View our privacy policy here.