DOI:
10.1039/B816356C
(Paper)
CrystEngComm, 2009,
11, 151-159
Four-, and six-connected entangled frameworks based on flexible bis(imidazole) ligands and long dicarboxylate anions†
Received
17th September 2008
, Accepted 18th September 2008
First published on 15th October 2008
Abstract
A series of four- and six-connected entangled structures, namely [Cd(oba)(bbi)]·0.5H2O (1), [Ni(oba)(bbi)]2·H2O (2), [Co(bpea)(bbi)]·H2O (3), [Co2(bpea)2(bbi)2]·1.5H2O (4), [Cd(oba)(1,4-bix)] (5), and [Ni2(oba)2(1,4-bix)(H2O)2] (6), where oba = 4,4′-oxybis(benzoate), bpea = biphenylethene-4,4′-dicarboxylate, 1,4-bix = 1,4-bis(imidazol-1-ylmethyl)benzene, and bbi = 1,1′-(1,4-butanediyl)bis(imidazole), have been successfully synthesized through a hydrothermal process. The structures of 1 and 2 both consist of two interpenetrating CdSO4 nets. In 1, the two nets are related by symmetry. By comparison, the individual nets are, unusually, crystallographically distinct in 2. The structures of 3 and 4 both contain interpenetrating diamond networks. In 3, there are 6 interpenetrating nets, and they show the usual mode of interpenetration. For 4, however, there are 7 nets, and a rare ‘abnormal’ [4 + 3] interpenetration mode is observed. In 5, the oba and 1,4-bix ligands linked the Cd(II) atoms into a deeply corrugated 2D sheet. The corrugated 2D sheets polycatenate each other in a parallel manner with DOC (degree of catenation) = 2, yielding a rare 2D → 3D parallel polycatenation net. In 6, the dinuclear Ni(II) units are bridged by the oba ligands to generate a 2D (4,4) square-grid with a rhombic window. It is interesting that the two identical (4,4) square-grids show 2D → 2D parallel interpenetration but are then further crosslinked by the 1,4-bix ligands to yield an unusual 3D self-penetrating net with an unique 6-connected 44.611 topology. The importance of bbi conformations and metal sources in the framework formation of complexes 1–6 was unraveled. Their infrared spectra, powder X-ray diffraction (XRD) and photoluminescent properties were also investigated in detail.
Introduction
The current interest in polymeric coordination networks is rapidly expanding not only for their potential applications in host–guest chemistry, ion exchange, gas storage, and nonlinear optics, but also for their intriguing variety of topologies.1 Structural diversity in coordination polymers can occur as a result of various processes, including supramolecular isomerism, interpenetration, or interweaving. Among these, entangled systems (catenanes, rotaxanes, and molecular knots) have attracted a great deal of attention from chemists because of their intrinsic aesthetic appeal.2 Interpenetrating networks, as an important subject in the area of entanglement, have provided a long-standing fascination for chemists. These species can be regarded as infinite, ordered polycatenanes or polyrotaxanes, and are characterized by the presence of two or more independent networks that are inextricably entangled through the rings belonging to one framework.2a So far, a variety of appealing interpenetrated frameworks have been constructed and discussed in several excellent reviews.2 Among the new types of entanglements in polymeric architectures, the phenomenon of self-penetration has attracted much attention in recent times.3 These species are single nets, having the peculiarity that the smallest topological rings are catenated by other rings belonging to the same network. Hitherto, only a limited number of self-penetrated nets have been observed in metal–organic frameworks, as evidenced in a review by Ciani and co-workers.2b Thus, it is a great challenge to achieve self-penetrating frameworks in coordination chemistry.
It is well-known that product topology can often be controlled and modified by selecting the coordination geometry preferred by the metal ion and the chemical structure of the organic ligand chosen. Usually, long ligands will lead to larger voids that may result in interpenetrated structures.3e Therefore, dicarboxylate and N-containing ligands with these characteristics are excellent candidates for the construction of entangled frameworks. In this contribution, six new entangled structures were obtained by using flexible bix(imidazole) ligands and long dicarboxylate ainons: [Cd(oba)(bbi)]·0.5H2O (1), [Ni(oba)(bbi)]2·H2O (2), [Co(bpea)(bbi)]·H2O (3), [Co2(bpea)2(bbi)2]·1.5H2O (4), [Cd(oba)(1,4-bix)] (5), and [Ni2(oba)2(1,4-bix)(H2O)2] (6), where oba = 4,4′-oxybis(benzoate), bpea = biphenylethene-4,4′-dicarboxylate, 1,4-bix = 1,4-bis(imidazol-1-ylmethyl)benzene, and bbi = 1,1′-(1,4-butanediyl)bis(imidazole) (Chart 1). The compounds are characterized by elemental analysis, IR spectra, powder X-ray diffraction (XRD), and X-ray crystallography. The crystal structures as well as the topological analysis of these compounds and the systematic investigation of the effect of conformation of the bis(imidazole) spacer, along with different metal ions on the ultimate frameworks, will be discussed. The photoluminescent properties for 1 and 5 are also discussed in detail.
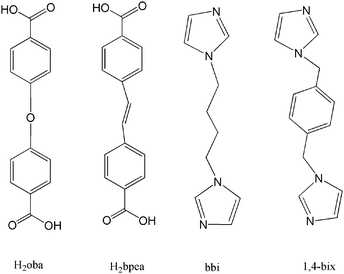 |
| Chart 1 The structures of carboxylates and di-nitrogen ligands | |
Experimental
Materials and methods
All reagents and solvents for the syntheses were purchased from commercial sources and used as received. The bbi and 1,4-bix were synthesized by following the procedures described previously.4 The C, H, and N elemental analyses were conducted on a Perkin–Elmer 240C elemental analyzer. The FT-IR spectra were recorded from KBr pellets in the range 4000–400 cm−1 on a Mattson Alpha-Centauri spectrometer. Powder XRD patterns of samples 1 and 5 were collected on a Rigaku Dmax 2000 X-ray diffractometer with graphite monochromated Cu Kα radiation (λ = 0.154 nm) and 2θ ranging from 4 to 40°. The solid-state emission/excitation spectra of compounds 1 and 5 were recorded on a Varian Cary Eclipse spectrometer at room temperature.
Synthesis of [Cd(oba)(bbi)]·0.5H2O (1).
Cd(NO3)2·4H2O (145 mg, 0.5 mmol), H2oba (134 mg, 0.5 mmol), and bbi (119 mg, 0.5 mmol) were dissolved in distilled water (10 mL), and triethylamine was added until the pH value of the system was adjusted to about 5.5. The resulting solution was stirred for about 1 h at room temperature, sealed in a 23-mL Teflon-lined stainless steel autoclave and heated at 140 °C for 6 d under autogenous pressure. Then the mixture was cooled to room temperature, and colourless crystals of 1 were obtained in a 49% yield based on Cd(II). Elemental analysis results for C48H46Cd2N8O11: Calcd (%): C 50.76, H 4.08, N, 9.87; Found (%): C 50.42, H 4.40, N 9.93. FT-IR (KBr pellet, cm−1): 3123 (w), 2937 (w), 2870 (w), 1683 (w), 1589 (w), 1509 (s), 1467 (m), 1402 (s), 1229 (s), 1086 (m), 930 (m), 740 (m), 657 (m).
Synthesis of [Ni(oba)(bbi)]2·H2O (2).
Compound 2 was prepared in the same way as 1, using Ni(NO3)2·6H2O (0.5 mmol) instead of Cd(NO3)2·4H2O as the metal source. Green crystals of 2 were obtained in 57% yield based on Ni(II). Elemental analysis results for C48H46N8Ni2O11: Calcd (%): C 56.06, H 4.51, N, 10.90; Found (%): C 56.43, H 4.67, N 10.61. FT-IR (KBr pellet, cm−1): 3446 (w), 3125 (w), 2937 (w), 1593 (s), 1535 (s), 1421 (s), 1288 (w), 1234 (s), 1158 (w), 1095 (m), 877 (m), 786 (w), 660 (m).
Synthesis of [Co(bpea)(bbi)]·H2O (3).
Compound 3 was prepared in the same way as 1, using Co(NO3)2·6H2O (0.5 mmol) and H2bpea (0.5 mmol) instead of Cd(NO3)2·4H2O and H2oba as the metal source and dicarboxylate ligand. Pink crystals of 3 were obtained in 36% yield based on Co(II). Elemental analysis results for C26H26CoN4O5: Calcd (%): C 58.54, H 4.91, N, 10.51; Found (%): C 58.71, H 4.33, N 10.70. FT-IR (KBr pellet, cm−1): 3227 (w), 3113 (w), 2937 (w), 2881 (w), 1681 (w), 1586 (m), 1540 (s), 1516 (s), 1379 (s), 1326 (s), 1228 (w), 1093 (s), 938 (w), 787 (m), 658 (m).
Synthesis of [Co2(bpea)2(bbi)2]·1.5H2O (4).
Pink crystals of 4 were obtained together with crystals of 3 in 27% yield based on Co(II). The former can be manually selected from the mixture by judging the shape of the crystals to some extent. Elemental analysis results for C104H99Co4N16O19: Calcd (%): C 59.12, H 4.72, N, 10.61; Found (%): C 59.29, H 4.48, N 10.80. FT-IR (KBr pellet, cm−1): 3229 (w), 3113 (w), 2937 (w), 1680 (w), 1587 (m), 1540 (s), 1516 (m), 1379 (s), 1227 (m), 1093 (m), 939 (w), 786 (w), 657 (w).
Synthesis of [Cd(oba)(1,4-bix)] (5).
Compound 5 was prepared in the same way as 1, using 1,4-bix (0.5 mmol) instead of bbi as the secondary ligand. Colorless crystals of 5 were obtained in 58% yield based on Cd(II). Elemental analysis results for C28H22CdN4O5: Calcd (%): C 55.41, H 3.65, N, 9.23; Found (%): C 55.70, H 3.81, N 9.03. FT-IR (KBr pellet, cm−1): 3111 (w), 2921 (w), 1682 (w), 1595 (s), 1543 (s), 1506 (m), 1399 (s), 1231 (s), 1157 (w), 1090 (m), 937 (w), 786 (s), 713 (m), 652 (m), 508 (w).
Synthesis of [Ni2(oba)2(1,4-bix)(H2O)2] (6).
Compound 6 was prepared in the same way as 1, using Ni(NO3)2·6H2O (0.5 mmol) and 1,4-bix (0.5 mmol) instead of Cd(NO3)2·4H2O and bbi as the metal source and secondary ligand. Green crystals of 6 were obtained in 53% yield based on Ni(II). Elemental analysis results for C84H68N8Ni4O248: Calcd (%): C 55.79, H 3.79, N, 6.20; Found (%): C 55.43, H 3.48, N 6.55. FT-IR (KBr pellet, cm−1): 3114 (w), 2941 (w), 1683 (w), 1593 (s), 1523 (s), 1501 (m), 1422 (m), 1232 (s), 1159 (m), 1096 (m), 947 (w), 875 (m), 768 (m), 712 (m), 654 (m).
Single-crystal X-ray diffraction data for complexes 1–6 were recorded on a Bruker-AXS Smart CCD diffractometer, using ω scan technique with Mo Kα radiation (λ = 0.71073 Å). The structures of the compounds were solved by the direct method using the program SHELXS-975 and refined by full-matrix least-squares techniques against F2 using the SHELXL-976 crystallographic software package. All non-hydrogen atoms were easily found from the Fourier difference maps and refined anisotropically, whereas the hydrogen atoms of the organic molecules were placed by geometrical considerations and were added to the structure factor calculation. The disordered C atoms of the bpea ligand in compound 3 (C13, C13′) were refined using a C atoms split over two sites, with a total occupancy of 1. The hydrogen atoms of the water molecules for 2 and 6 were located using Fourier difference maps. The hydrogen atoms of the water molecules for 1 and 3–5 were not located from Fourier difference maps. The detailed crystallographic data and structure refinement parameters for 1–6 are summarized in Tables 1 and 2.
Compound |
1
|
2
|
3
|
4
|
5
|
6
|
R
1 = ∑ ||F0| − |Fc||/∑ |F0| wR2 = {∑ [w(F20 − F2c)2]/∑ [w(F20)]2}1/2.
|
Formula |
C48H46Cd2N8O11 |
C48H46N8Ni2O11 |
C26H24CoN4O5 |
C104H99Co4N16O19 |
C28H22CdN4O5 |
C84H68N8Ni4O24 |
Formula mass |
1135.73 |
1028.35 |
531.42 |
2112.71 |
606.90 |
1808.30 |
Space group |
Fdd2 |
Cc
|
P2/c |
P-1
|
Pnma
|
C2/c
|
a/Å |
20.008(2) |
8.8554(11) |
12.999(3) |
8.6765(9) |
14.7584(10) |
28.442(3) |
b/Å |
53.655(6) |
19.877(2) |
6.7152(14) |
11.9414(12) |
14.2630(9) |
5.7468(6) |
c/Å |
8.9809(10) |
26.017(3) |
16.547(3) |
24.044(2) |
12.1990(8) |
24.666(3) |
α/° |
90 |
90 |
90 |
81.697(2) |
90 |
90 |
β/° |
90 |
97.727(2) |
95.999(4) |
88.455(2) |
90 |
112.039(2) |
γ [°] |
90 |
90 |
90 |
84.325(2) |
90 |
90 |
V/Å3 |
9641.1(18) |
4538.0(10) |
1436.5(5) |
2452.8(4) |
2567.9(3) |
3737.0(7) |
Z
|
8 |
4 |
2 |
1 |
4 |
2 |
ρ/g cm−3 |
1.565 |
1.505 |
1.229 |
1.430 |
1.570 |
1.607 |
µ/mm−1 |
0.951 |
0.902 |
0.636 |
0.743 |
0.897 |
1.082 |
Refl. measured |
13409 |
12657 |
7582 |
13946 |
13791 |
9981 |
Unique refl. (Rint) |
4753 (0.0566) |
6422 (0.0691) |
2748 (0.0845) |
9489 (0.0362) |
2643 (0.0221) |
3669 (0.0408) |
T/K |
293(2) |
293(2) |
293(2) |
293(2) |
293(2) |
293(2) |
Goodness-of-fit on F2 |
0.898 |
0.878 |
1.033 |
1.038 |
1.043 |
1.022 |
R [I > 2σ(I)] |
R
1 = 0.0471 |
R
1 = 0.0543 |
R
1 = 0.0950 |
R
1 = 0.0672 |
R
1 = 0.0224 |
R
1 = 0.0414 |
wR
2 = 0.0853 |
wR
2 = 0.0854 |
wR
2 = 0.2125 |
wR
2 = 0.1296 |
wR
2 = 0.0561 |
wR
2 = 0.0900 |
Results and discussion
Structure of [Cd(oba)(bbi)]·0.5H2O (1)
Selected bond distances and angles for compounds 1–6 are listed in Tables S1–S6 (see ESI).† Single-crystal X-ray structural analysis reveals that the structure of 1 contains one crystallographically unique Cd(II) atom, one oba anion, one bbi ligand, and one half water molecule. As shown in Fig. 1a, each Cd(II) center is six-coordinated to four carboxylate oxygen atoms (Cd1–O1 = 2.401(5), Cd1–O2 = 2.307(4), Cd1–O3#1 = 2.432(4) and Cd1–O4#1 = 2.298(5) Å) from two different oba ligands, two imidazole nitrogen atoms (Cd1–N1 = 2.284(7) and Cd1–N3 = 2.240(6) Å) from two distinct bbi ligands. The geometry around the Cd(II) center can be best described as a slightly distorted octahedron. The extension of the structure into a 3D network is accomplished by connecting two oba anions and two bbi ligands to the Cd(II) centers. The topological analysis of 1 reveals that it is a typically CdSO4 framework (Fig. 1b), where each Cd(II) atom acts as a 4-connected node. The two identical CdSO4 nets are further interpenetrated in 2-fold mode (Fig. 1c).
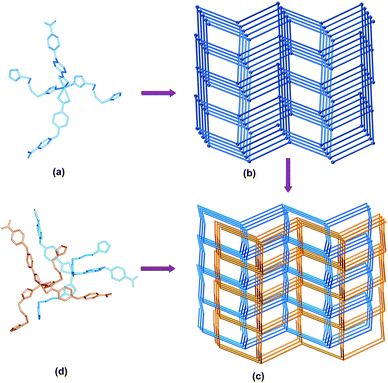 |
| Fig. 1 (a) Local coordination geometry of the central Cd(II) atom of 1. (b) Schematic representation of CdSO4 topology of 1. (c) The two interpenetrating CdSO4 networks. For 1, both nets are equivalent. For 2, the net containing Ni1 is shaded orange, while the net containing Ni2 is shaded blue. (d) Local coordination geometry of the central Ni(II) atom of 2. | |
Structure of [Ni(oba)(bbi)]2·H2O (2)
The structure of 2 contains two crystallographically unique Ni(II) atoms, two oba anions, two bbi ligands, and one water molecules. The view of the local coordination geometries around the Ni(II) atoms are shown in Fig. 1d. Each Ni(II) atom lies on a center of symmetry and is coordinated with two bbi ligands vianitrogen atoms (Ni1–N1 = 2.040(6) and Ni1–N4#3 = 2.017(6) Å) and two oba ligands via carboxylic oxygen atoms (Ni1–O1 = 2.101(5), Ni1–O2 = 2.139(5), Ni1–O3#1 = 2.135(5) and Ni1–O4#1 = 2.109(5) Å) in a distorted octahedral coordination geometry. Two crystallographically unique Ni(II) atoms are bridged by the oba anions and bbi ligands to give two crystallographically distinct CdSO4 nets, which are interpenetrated in 2-fold mode (Fig. 1c).
It should be pointed out that the structures of 1 and 2 both consist of two interpenetrating CdSO4 nets (Fig. 1c). Although the net topologies and the way they interpenetrate are identical, there are some significant differences in the two structures. They crystallise in different space groups, and in 2 the individual nets are, unusually, crystallographically distinct. By comparison, the two nets in 1 are related by symmetry. Compound 2 was prepared in the same way as 1, using Ni(NO3)2·6H2O instead of Cd(NO3)2·4H2O as the metal source. The differences, such as space groups and the individual nets, are mainly attributed to the metal sources used here. To the best of our knowledge, of the previously-reported coordination polymers, none of them are interpenetrated through two crystallographically distinct CdSO4 nets in 2-fold mode.7
Structure of [Co(bpea)(bbi)]·H2O (3)
Fig. 2 depicts a molecular structure showing the arrangements about the Co(II) center. There is one crystallographically independent Co(II) atom in the structure, and the Co(II) atom adopts a distorted octahedral geometry, completed by four carboxylate oxygen atoms (Co1–O1 = 2.219(7), Co1–O2 = 2.240(8), Co1–O1#1 = 2.219(7) and Co1–O2#1 = 2.240(8) Å) from two different bpea anions and two nitrogen atoms (Co1–N1 = 2.027(5) and Co1–N1#1 = 2.027(5) Å) from two distinct bbi ligands. The bpea anions in bis-chelating modes and the bbi ligands in TTT conformations (T = trans and G = gauche) (Fig. S7)† bridge the Co(II) atoms to give an overall 3D structure. The topological analysis of 3 reveals that it is a typically diamond framework containing large adamantanoid cages. Fig. 3 shows a single cage delimited by four cyclohexane-like windows in chair conformations. As can be seen, the adamantanoid cages are elongated significantly in one direction, and exhibit maximum dimensions (corresponding to the longest intracage Co⋯Co distances) of 30.911 × 28.457 Å. Because of the spacious nature of the single network, it allows five identical diamond networks to interpenetrate it in a normal mode, resulting in a 6-fold interpenetrating diamond array (Fig. 4), with isolated water molecules occupying the neutral channels.2d
![The coordination environment for Co(II) atom in 3. Displacement ellipsoids are drawn at the 20% probability level. [Symmetry code: (A) −x, y, 1.5 − z].](/image/article/2009/CE/b816356c/b816356c-f2.gif) |
| Fig. 2 The coordination environment for Co(II) atom in 3. Displacement ellipsoids are drawn at the 20% probability level. [Symmetry code: (A) −x, y, 1.5 − z]. | |
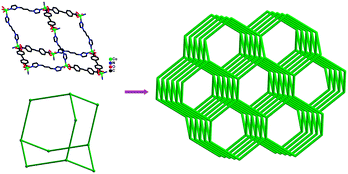 |
| Fig. 3 Single adamantanoid cages (left) and a schematic view of a single diamond framework (right). | |
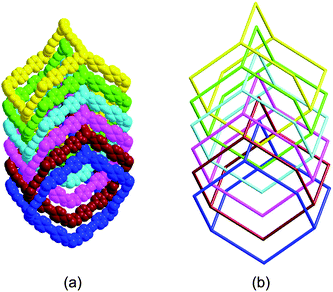 |
| Fig. 4 (a) Space-filling diagram of the six interpenetrating adamantanoid cages. (b) Schematic representation of the six interpenetrating diamond networks. | |
Structure of [Co2(bpea)2(bbi)2]·1.5H2O (4)
As shown in Fig. 5, in the fundamental building unit of polymer 4 each Co(II) atom in distorted octahedral coordination geometry is linked by four carboxylate oxygen atoms from two different bis-chelating bpea ligands and two nitrogen atoms from two distinct bbi ligands. The central Co(II) atoms are interlinked by the bpea anions and bbi ligands to generate an expanded diamond net. Fig. 6 shows a single cage delimited by four cyclohexane-like windows in chair conformations. Water molecules are found in the adamantane cages of the network. As shown in Fig. 6, the hexagonal channels of 28.978 × 21.060 Å are expanded from the diamond net (corresponding to the longest intracage Co⋯Co distances). However, polymer 4 avoids an extremely large void space by constructing a 7-fold interpenetrating framework (Fig. 7) in an unusual [4 + 3] mode. Obviously, this interpenetration mode differs from the normal mode and can be described as two sets of normal 4- and 3-fold nets, that is, an unusual [4 + 3] mode of interpenetration.8
![The coordination environments for Co(II) atoms in 4. Displacement ellipsoids are drawn at the 20% probability level. [Symmetry codes: (A) x − 2, y, 1 + z; (B) x, 1 + y, 1 + z].](/image/article/2009/CE/b816356c/b816356c-f5.gif) |
| Fig. 5 The coordination environments for Co(II) atoms in 4. Displacement ellipsoids are drawn at the 20% probability level. [Symmetry codes: (A) x − 2, y, 1 + z; (B) x, 1 + y, 1 + z]. | |
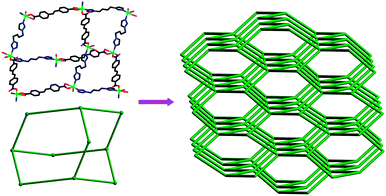 |
| Fig. 6 Single adamantanoid cages (left) and a schematic view of a single diamond framework (right). | |
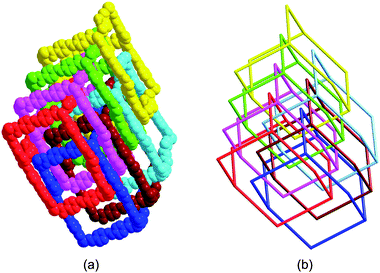 |
| Fig. 7 (a) Space-filling diagram of the seven interpenetrating adamantanoid cages. (b) Schematic representation of the seven interpenetrating diamond networks. | |
It is worth noting that the structures of 3 and 4 both contain interpenetrating diamond networks, although they have different numbers of nets and interpenetration topologies. In 3, there are 6 interpenetrating nets, and they show the usual mode of interpenetration. For 4, however, there are 7 nets, and a rare ‘abnormal’ [4 + 3] interpenetration mode is observed. Generally, the degree of interpenetration is strongly related to the spacer ligand. In contrast to 3, in 4 one bbi ligand adopts a TTT conformation, while the other bbi adopts a TGT mode (Fig. S7).† Further, the bpea anions coordinate to the Co(II) atoms in the same modes in 3 and 4 with the similar Co(II)⋯Co(II) separations (17.545 Å for 3, 17.402 and 17.688 Å for 4). Thus, the differences in interpenetrating modes displayed here, mainly arise from the different conformations of the bbi ligand.9
Structure of [Cd(oba)(1,4-bix)] (5)
The fundamental building unit of 5 contains one Cd(II) atom, one oba anion, and one 1,4-bix ligand (Fig. 8a). The Cd(II) center is coordinated by four carboxylate oxygen atoms (Cd1–O1 = 2.5313(18), Cd1–O2 = 2.2102(17), Cd1–O4#2 = 2.4191(14) and Cd1–O4#3 = 2.4191(14) Å) from two different oba ligands and two nitrogen atoms (Cd1–N1 = 2.2387(15) and Cd1–N1#1 = 2.2387(15) Å) from two distinct 1,4-bix ligands in a distorted octahedral coordination geometry. The Cd(II) centers are linked by oba anions and 1,4-bix ligands to form deeply corrugated 2D (4,4) sheets. Each four-membered ring is formed by four Cd(II) ions, two 1,4-bix, and two oba ligands (Fig. 8). The open space within each sheet leads to the formation of catenation between adjacent sheets that are parallel to one another and crystallographically equivalent (Fig. 9). This results in an infinite parallel polycatenation of the 4-connected sheets. Each sheet is penetrated by two others (one above and one below) which have parallel but not coincident mean planes, leading to an overall 3D entanglement (Fig. 9).
![(a) The coordination environment of Cd(II) atom in 5 (displacement ellipsoids are drawn at the 20% probability level). [Symmetry codes: (A) 1 + x, y, z; (B) 1 + x, 0.5 − y, z; (C) x, 0.5 − y, z]. (b) A (4,4) sheet in the structure of 5.](/image/article/2009/CE/b816356c/b816356c-f8.gif) |
| Fig. 8 (a) The coordination environment of Cd(II) atom in 5 (displacement ellipsoids are drawn at the 20% probability level). [Symmetry codes: (A) 1 + x, y, z; (B) 1 + x, 0.5 − y, z; (C) x, 0.5 − y, z]. (b) A (4,4) sheet in the structure of 5. | |
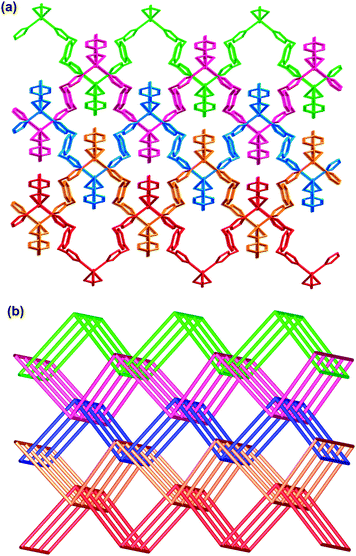 |
| Fig. 9 (a) The 2D → 3D parallel interpenetration of the sheets in 5. (b) Schematic representation of 2D → 3D parallel interpenetration of the sheets in 5. | |
To the best of our knowledge, only few examples of 2D → 3D entangled structures have been observed for polycatenating systems in parallel fashion. However, most of the studies have focused on the 3-connected (6,3) net due to their large voids. Among the known interpenetrating (4,4) networks in coordination polymers, the most examples are the mode of 2D → 2D parallel interpenetration or 2D → 3D inclined polycatenation.10 However, 2D → 3D parallel polycatenation with (4,4) nets is rarely observed, as evidenced in a review by Batten.2c
Structure of [Ni2(oba)2(1,4-bix)(H2O)2] (6)
Single crystal X-ray analysis revealed that compound 6 is constructed from one kind of Ni(II) atom, one kind of oba anion and one kind of 1,4-bix ligand (Fig. 10). The central Ni(II) atom is six-coordinated and exhibits a twisted octahedral coordination geometry, which is surrounded by three carboxylate oxygen atoms (Ni1–O1 = 1.9816(19), Ni1–O4#1 = 2.1046(18) and Ni1–O5#1 = 2.1194(19) Å) from two different oba anions, two water molecules (Ni1–O1W = 2.124(2) and Ni1–O1W#2 = 2.109(2) Å), and one imidazole nitrogen atom (Ni1–N1 = 2.047(2) Å) from one 1,4-bix ligand. All the bond distances and angles are within normal range. Two crystallographically equivalent Ni(II) atoms are bridged by two water molecules to give a Ni2(H2O)2 dimer, in which the Ni⋯Ni distance is 3.237 Å. Further, the Ni2(H2O)2 dimers are bridged by the oba ligands to generate a 2D (4,4) square-grid with a rhombic window. The dimensions of the net are 15.498 × 15.498 Å, corresponding to the distances between adjacent centers of dinuclear Ni(II) subunits at their corners. It is interesting that the two identical (4,4) square-grids show 2D → 2D parallel interpenetration but are then further crosslinked into a single 3D network by the 1,4-bix ligands (Fig. 10). Thus, the net is self-penetrating, with the 4-membered shortest circuits of the sheets containing further nodes of the same net at their centre rather than being penetrated by rods.
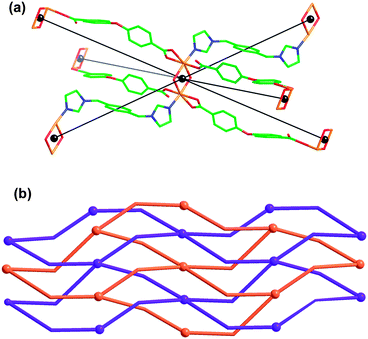 |
| Fig. 10 (a) Local coordination geometries and connectivity of the Ni2(H2O)2 dimer in 6. (b) Schematic representation of 2D → 2D parallel interpenetration network in 6. | |
Topologically, each Ni2(H2O)2 dimer is connected to six others through four oba anions and two 1,4-bix ligands, thus resulting in a unique 3D uninodal six-connected framework of 44.611 topology (Fig. 11). Up to now, interpenetrating networks have been extensively studied, as evident from a recent review by Ciani and co-workers, but the study of self-penetrating networks remains largely unexplored within coordination polymers, and is still in its infancy.2b It is well-known that the 6-connected nets are bridges, and the Ni(II) atoms are shown as red. One of the interpenetrating (4,4) subnets in the [Ni2(oba)2] layers is highlighted. essentially dominated by the α-polonium topology. Other 6-connected nets, especially 6-connected self-penetrating nets, are quite rare. There is an interesting relationship between current 44.611 net and other reported six-connected nets: LB-1 (446108), pcu (41263), roa (446108), and rob (48668): they are all six-connected nets based on (4,4) sheet.11 However, the greatest difference from our 44.611 net to other common 6-connected nets is the presence of the unique 2D → 2D parallel interpenetration of two identical (4,4) sheets.
![Schematic representation of the single self-penetrating 6-connected net in 6. Kinked orange links represent the oba bridges, straight black bonds represent the 1,4-bix bridges, and Ni(II) atoms are shown as red. One of the interpenetrating (4,4) subnets in the [Ni2(oba)2] layers is highlighted.](/image/article/2009/CE/b816356c/b816356c-f11.gif) |
| Fig. 11 Schematic representation of the single self-penetrating 6-connected net in 6. Kinked orange links represent the oba bridges, straight black bonds represent the 1,4-bix bridges, and Ni(II) atoms are shown as red. One of the interpenetrating (4,4) subnets in the [Ni2(oba)2] layers is highlighted. | |
The driving force for the formation of this unusual topology becomes apparent when the [Ni2(oba)2] sheets are examined in detail. Double helical chain motifs are formed in which the two strands are aligned so that multiple hydrogen bonds form between the clusterwater protons of one chain and carboxylate oxygen atoms of the other strand, and vice versa (Fig. 12 and Fig. S8†).
![(a) Space-filling diagram of the double helix motif in the [Ni2(oba)2] layers. (b) Schematic representation of the double helix motif.](/image/article/2009/CE/b816356c/b816356c-f12.gif) |
| Fig. 12 (a) Space-filling diagram of the double helix motif in the [Ni2(oba)2] layers. (b) Schematic representation of the double helix motif. | |
Photoluminescent properties
In order to substantiate the phase purity of the as-synthesized products 1 and 5, their powder XRD were performed before their photoluminescent properties were measured. The experimental powder XRD patterns are in good agreement with the corresponding simulated ones (Fig. 13) except for the relative intensity variation because of preferred orientations of the crystals. Coordination polymers with d10 metal centers and organic ligands are promising candidates for photoactive materials with potential applications.12 In this paper, the solid state photoluminescent properties of compounds 1 and 5 have been investigated in the solid state at room temperature. Two emission peaks at about 395 (λex = 250 nm) and 356 nm (λex = 300 nm) were observed for free bbi and 1,4-bix ligands, respectively.13 The emission peak of free H2oba ligand eas located at about 495 nm with the excitation peak at 425 nm.14 The emission bands for these free ligands are probably attributable to the π * →n or π * →π transitions.15 On complexation of these ligands with Cd(II) atoms, intense fluorescent emissions occur at 454 nm (λex = 395 nm) for 1 and 483 nm (λex = 395 nm) for 5 (Fig. 14). Compared with 1, a bathochromic shift of emission occurs in 5, which is probably due to the difference of the bis(imidazole) ligands because the photoluminescent behavior is closely associated with the ligands coordinated around the metal centres. However, the emissions arising from the free ligands are not observable for compounds 1 and 5. The absence of ligand-based emission suggests energy transfer from the ligands to the Cd(II) centers during photoluminescence. Thus, the photoluminescence can be attributed to the ligand-to-metal charge-transfer (LMCT) transitions.10j Both complexes 1 and 5 may be excellent candidates for fluorescent materials.
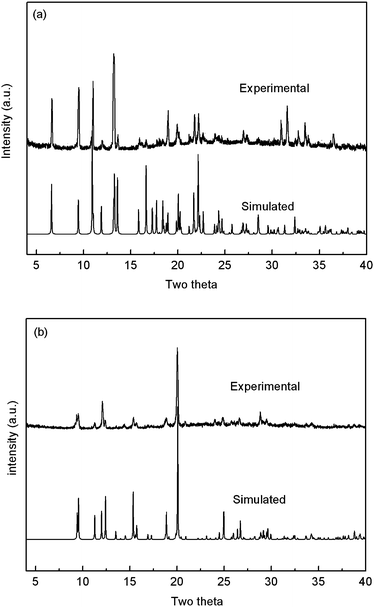 |
| Fig. 13 The simulated and experimental powder XRD patterns for compounds 1 (a) and 5 (b). | |
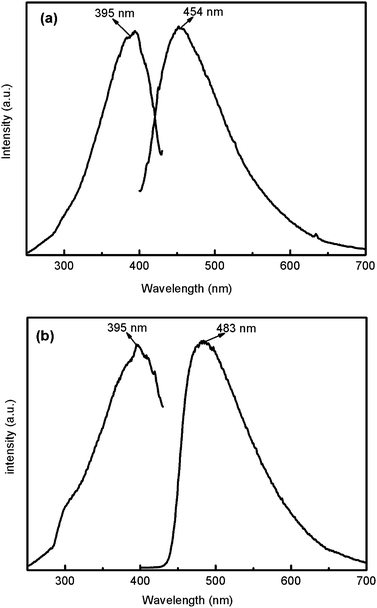 |
| Fig. 14 Solid-state excitation and emission spectra of compounds 1 (a) and 5 (b). | |
Conclusion
A series of four-, and six-connected entangled frameworks based on flexible bis(imidazole) ligands and long dicarboxylate anions have been successfully isolated under hydrothermal conditions. Through comparison of the structures of 3 and 4, it is apparent that the conformation of the flexible bbi ligand has a significant influence on the formation of the resulting structures. In 3, the bbi ligand adopts TTT conformation, leading to a normal 6-fold interpenetrating diamond structure. However, in 4, the presence of TGT bbi ligand leads to an unusual 7-fold interpenetrating the daimond framework. On the other hand, the metal centers also play an important role in the presence of the bis(imidazole) ligand as well as the same dicarboxylate ligands. Although compounds 1 and 2 were prepared by using Ni(NO3)2·6H2O and Cd(NO3)2·4H2O as the metal sources under a similar hydrothermal conditions, they show different structures. In 1, the two CdSO4 nets are related by symmetry, whereas the individual CdSO4 nets are crystallographically distinct in 2. Further, when the introduction of Ni(NO3)2·6H2O instead of Cd(NO3)2·4H2O as the metal source results in the formation of a 2D → 3D parallel polycatenation net of 5, which is significantly different from the unusual 3D self-penetrating net of 6. As mentioned above, the results indicate the importance of bbi conformations and metal sources in the framework formation of the coordination polymers 1–6. The photoluminescent emissions show that the complexes 1 and 5 may be good candidates for optical materials.
Acknowledgements
We thank the National Natural Science Foundation of China (No. 20471014), Program for the New Century Excellent Talents in Chinese University (NCET-05-0320), Program for Changjiang Scholars and Innovative Research Teams in Chinese University, the Postdoctoral Foundation of Northeast Normal University and the Science Foundation for Young Teachers of Northeast Normal University (No. 20060304) for support.
References
-
(a) O. M. Yaghi, M. O'Keeffe, N. W. Ockwig, H. K. Chae, M. Eddaoudi and J. Kim, Nature, 2003, 423, 705 CrossRef CAS;
(b) S. Kitagawa, R. Kitaura and S. I. Noro, Angew. Chem., Int. Ed., 2004, 43, 2334 CrossRef CAS;
(c) A. N. Khlobystov, A. J. Blake, N. R. Champness, D. A. Lemenovskii, A. G. Majouga, N. V. Zyk and M. Schröer, Coord. Chem. Rev., 2001, 222, 155 CrossRef CAS;
(d) B. Moulton and M. J. Zaworotko, Chem. Rev., 2001, 101, 1629 CrossRef CAS;
(e) S. R. Batten, B. F. Hoskins and R. Robson, Angew. Chem., Int. Ed. Engl., 1997, 36, 636 CrossRef CAS;
(f) C. Janiak, Dalton Trans., 2003, 2781 RSC;
(g) S. L. James, Chem. Soc. Rev., 2003, 32, 276 RSC;
(h) S. Hu, J.-C. Chen, M.-L. Tong, B. Wang, Y.-X. and S. R. Batten, Angew. Chem., Int. Ed., 2005, 44, 5471 CrossRef CAS.
-
(a) S. R. Batten and R. Robson, Angew. Chem., Int. Ed., 1998, 37, 1460 CrossRef;
(b) L. Carlucci, G. Ciani and D. M. Proserpio, Coord. Chem. Rev., 2003, 246, 247 CrossRef CAS;
(c) S. R. Batten, CrystEngComm, 2001, 3, 67 RSC;
(d) V. A. Blatov, L. Carlucci, G. Ciani and D. M. Proserpio, CrystEngComm, 2004, 6, 377 RSC.
-
(a) B. F. Abrahams, S. R. Batten, M. J. Grannas, H. Hamit, B. F. Hoskins and R. Robson, Angew. Chem., Int. Ed., 1999, 38, 1475 CrossRef CAS;
(b) M.-L. Tong, X.-M. Chen and S. R. Batten, J. Am. Chem. Soc., 2003, 125, 16170 CrossRef CAS;
(c) X.-H. Huang, T.-L. Sheng, S.-C. Xiang, R.-B. Fu, S.-M. Hu, Y.-M. Li and X.-T. Wu, Inorg. Chem., 2007, 46, 497 CrossRef CAS;
(d) S. R. Batten, A. R. Harris, P. Jensen, K. S. Murray and A. Ziebell, J. Chem. Soc., Dalton Trans., 2000, 3829 RSC;
(e) X.-L. Wang, C. Qin, E.-B. Wang, Y.-G. Li, Z.-M. Su, L. Xu and L. Carlucci, Angew. Chem., Int. Ed., 2005, 44, 5824 CrossRef CAS;
(f) X.-L. Wang, C. Qin, E.-B. Wang and Z.-M. Su, Chem.–Eur. J., 2006, 12, 2680 CrossRef CAS;
(g) L. Carlucci, G. Ciani, D. M. Proserpio and S. Rizzato, Chem.–Eur. J., 2002, 8, 1520 CrossRef.
-
(a) J.-F. Ma, J. Yang, G.-L. Zheng, L. Li and J.-F. Liu, Inorg. Chem., 2003, 42, 7531 CrossRef CAS;
(b) J. Yang, J.-F. Ma, S. R. Batten and Z.-M. Su, Chem. Commun., 2008, 2233 RSC.
-
G. M. Sheldrick, SHELXS-97, Programs for X-ray Crystal Structure Solution; University of Göttingen: Göttingen, Germany, 1997 Search PubMed.
-
G. M. Sheldrick, SHELXL-97, Programs for X-ray Crystal Structure Refinement; University of Göttingen: Göttingen, Germany, 1997 Search PubMed.
-
(a)
A. F. Wells, Further Studies of Three-dimensional Nets; ACA Monograph No. 8; American Crystallographic Association: Buffalo, NY, 1979 Search PubMed;
(b) J. V. Smith, Chem. Rev., 1988, 88, 149 CrossRef CAS;
(c)
M. O'Keeffe and B. G. Hyde, Crystal Structures, I. Patterns and Symmetry, Mineralogical Society of America, Washington, DC, 1996 Search PubMed;
(d) O. Delgado Friedrichs, M. O'Keeffe and O. M. Yaghi, Acta Crystallogr., Sect. A: Found. Crystallogr., 2003, 59, 22 CrossRef.
-
(a) Y.-F. Hsu, C.-H. Lin, J.-D. Chen and J.-C. Wang, Cryst. Growth Des., 2008, 8, 1096;
(b) D. S. Reddy, K. E. Dewa and Y. Aoyama, Angew. Chem., Int. Ed., 2000, 39, 4266 CrossRef CAS.
-
(a) B.-X. Dong, J. Peng, C. J. Gómez-García, S. Benmansour, H.-Q. Jia and N.-H. Hu, Inorg. Chem., 2007, 46, 5933 CrossRef CAS;
(b) S.-L. Li, Y.-Q. Lan, J.-F. Ma, J. Yang, G.-H. Wei, L.-P. Zhang and Z.-M. Su, Cryst. Growth Des., 2008, 8, 675 CrossRef CAS.
-
(a) B. J. Holliday and C. A. Mirkin, Angew. Chem., Int. Ed., 2001, 40, 2022 CrossRef CAS;
(b) S. Leininger, B. Olenyuk and P. J. Stang, Chem. Rev., 2000, 100, 853 CrossRef CAS;
(c) M. W. Hosseini, Acc. Chem. Res., 2005, 38, 313 CrossRef CAS;
(d) J.-Q. Liu, Y.-Y. Wang, L.-F. Ma, F. Zhong, X.-R. Zeng, W.-P. Wu and Q.-Z. Shi, Inorg. Chem. Commun., 2007, 10, 979 CrossRef CAS;
(e) P. J. Steel, Acc. Chem. Res., 2005, 38, 243 CrossRef CAS;
(f) L. Brammer, Chem. Soc. Rev., 2004, 33, 476 RSC;
(g) D. L. Caulder and K. N. Raymond, Acc. Chem. Res., 1999, 32, 975 CrossRef CAS;
(h) Y. Cui, S. J. Lee and W. Lin, J. Am. Chem. Soc., 2003, 125, 6014 CrossRef CAS;
(i) U. Knof and A. Zelewsky, Angew. Chem., Int. Ed., 1999, 38, 302 CrossRef;
(j) G.-H. Wei, J. Yang, J.-F. Ma, Y.-Y. Liu, S.-L. Li and L.-P. Zhang, Dalton Trans., 2008, 3080 RSC;
(k) J. Zhang, E. Chew, S. Chen, J. T. H. Pham and X. Bu, Inorg. Chem., 2008, 47, 3495 CrossRef CAS.
-
(a) J. Zhang, Y.-G. Yao and X. Bu, Chem. Mater., 2007, 19, 5083 CrossRef CAS;
(b) Y.-Y. Qin, J. Zhang, Z.-J. Li, L. Zhang, X.-Y. Cao and Y.-G. Yao, Chem. Commun., 2008, 2532 RSC.
-
(a) S.-L. Zheng and X.-M. Chen, Aust. J. Chem., 2004, 57, 703 CrossRef CAS;
(b) S.-L. Zheng, J.-H. Yang, X.-L. Yu, X.-M. Chen and W.-T. Wong, Inorg. Chem., 2004, 43, 830 CrossRef CAS;
(c) J. E. McGarrah, Y.-J. Kim, M. Hissler and R. Eisenberg, Inorg. Chem., 2001, 40, 4510 CrossRef CAS;
(d) G. D. Santis, L. Fabbrizzi, M. Licchelli, A. Poggi and A. Taglietti, Angew. Chem., Int. Ed. Engl., 1996, 35, 202 CrossRef.
- F.-F. Li, J.-F. Ma, S.-Y. Song and J. Yang, Cryst. Growth Des., 2006, 6, 209 CrossRef CAS.
- J. Yang, J.-F. Ma, Y.-Y. Liu, J.-C. Ma and S. R. Batten, Inorg. Chem., 2007, 46, 6542 CrossRef CAS.
-
(a) W. Chen, J. Y. Wang, C. Chen, Q. Yue, H. M. Yuan, J. S. Chen and S. N. Wang, Inorg. Chem., 2003, 42, 944 CrossRef CAS;
(b) X. Wang, C. Qin, E. Wang, Y. Li, N. Hao, C. Hu and L. Xu, Inorg. Chem., 2004, 43, 1850 CrossRef CAS;
(c) Y.-Q. Sun, J. Zhang, Y.-M. Chen and G.-Y. Yang, Angew. Chem., Int. Ed., 2005, 44, 5814 CrossRef CAS.
Footnote |
† Electronic supplementary information (ESI) available: IR spectra of compounds 1–6 (Fig. S1–S6); the TTT and TGT conformations of bbi ligands (Fig. S7); a double helix motif in the [Ni2(oba)2] layers (Fig. S8); selected bond lengths and angles for 1–6 (Tables S1–S6). CCDC reference numbers 693960–693965. For ESI and crystallographic data in CIF or other electronic format see DOI: 10.1039/b816356c |
|
This journal is © The Royal Society of Chemistry 2009 |
Click here to see how this site uses Cookies. View our privacy policy here.