DOI:
10.1039/B813226G
(Paper)
CrystEngComm, 2009,
11, 183-188
Syntheses, structures and sorption properties of two framework-isomeric porous copper-coordination polymers†
Received
31st July 2008
, Accepted 9th October 2008
First published on 3rd November 2008
Abstract
Solvothermal and solution reactions of a V-shaped organic ligand 4,4′-oxybis(benzoic acid) (H2oba) and Cu(NO3)2 yield two porous coordination polymers, namely [Cu(oba)(dmso)] (MCF-21) and [Cu2(oba)2(dmf)(C2H5OH)]·(dmf)2(H2O) (MCF-22·guest) (dmso = dimethyl sulfoxide, dmf = dimethylformamide), respectively. Single-crystal X-ray analyses reveal that the metal carboxylate backbones of MCF-21 and MCF-22·guest are framework-isomeric as two uninodal four-connected nets, i.e. a two-dimensional (2-D) 44 layer and a 3-fold interpenetrated lvt (4284) three-dimensional (3-D) network, respectively. After removing the solvent molecules, MCF-21 retains a rigid 2-D framework, but MCF-22·guest exhibits either rigid or dynamic behavior depending on the degree of desolvation, which is confirmed by powder X-ray diffraction and sorption isotherms. Meanwhile, the different sorption behavior of the framework-isomeric materials is elucidated by the discrepancies of their pore dimensions and framework rigidities.
Introduction
Porous coordination polymers (PCPs) have attracted intensive attention for the past decade owing to their diverse topologies and enormous potential applications for molecular storage and separation, catalysis, and ion exchange.1–5 Although a large number of PCPs with a certain level of surface area have been structurally established and verified by gas sorption, the rational design and synthesis of such materials with specific structures and functions are still a challenge. As a special phenomenon in supramolecular chemistry and crystal engineering, supramolecular isomerism has received considerable attention because of its close relation to supramolecular design and structure–property relationship investigations.6 Nevertheless, framework-isomeric PCPs are rarely observed in porous materials chemistry.7 Meanwhile, there is also an increasing interest in PCPs with flexible and dynamic frameworks, which are usually constructed by utilizing weak hydrogen bonds, π–π stacking and/or other supramolecular interactions,8 and in a unique case, by dangling side groups,9 to exhibit high selectivity for guest inclusion and structural transformation upon adsorption/desorption of guest molecules. Moreover, physically and chemically responsible PCPs whose porous properties can be tuned by external stimuli have emerged recently. Inspired by the fact that some flexible PCPs can be modified by guest species,10 one can expect that coordination modification of a suitable PCP may introduce more remarkable alteration for pore dimension and framework flexibility, giving rise to better responsive porous properties. Carboxylate-based coordination polymers, or metal-carboxylate frameworks (MCFs) have gained intensive interest as optoelectronic, magnetic and porous molecular materials. As a continuation of our extensive research on MCFs and a recent report of a 2-D guest-dependent dynamic MCF (MCF-20) constructed by Cu(II) and a V-shaped isophthalic acid (H2ip),11 we adopt a longer, flexible analogue 4,4′-oxybis(benzoic acid) (H2oba)12,13 to generate two framework-isomeric copper–organic framework materials [Cu(oba)(dmso)] (MCF-21) and [Cu2(oba)2(dmf)(C2H5OH)]·(dmf)2(H2O) (MCF-22·guest) under different synthetic conditions. Both compounds show a distinct structural character with another nonporous coordination polymer [Zn(oba)],12c in which oba2− exhibits complicated coordination modes and results in a solvent-free structure. Regarding the structural difference of the two framework-isomeric materials, they show distinct adsorption behavior. Moreover, the porous property of the Cu(II) carboxylate framework derived from MCF-22 can be successfully controlled by means of guest coordination.
Experimental
Materials and methods
All solvent and starting materials for synthesis were purchased commercially and used as received. Infrared (IR) spectra were obtained from KBr pellets on a Bruker TENSOR 27 Fourier transform infrared spectrometer in the 400–4000 cm−1 region. Elemental analysis (C, H, N) was performed on a Perkin-Elmer 240 elemental analyzer. Thermogravimetric analysis (TGA) was performed at a rate of 10 °C min−1 under N2 using a NETZSCH TG 209 system. Powder X-ray diffraction (PXRD) data were recorded on a Rigaku D/M-2200T automated diffractometer or on a Bruker D8 ADVANCE X-ray powder diffractometer (Cu Kα, 1.5418 Å).
Synthesis of [Cu(oba)(dmso)] (MCF-21)
A solution of Cu(NO3)2·3H2O (0.124 g, 0.5 mmol), H2oba (0.131 g, 0.5 mmol), dmso (5.0 mL), and isopropanol (5.0 mL) was placed in a 12-mL Teflon-lined autoclave and heated to 120 °C for 3 d affording block blue crystals (0.045 g, yield ca. 23% based on Cu). Anal. calcd (%) for C16H14CuO6S (397.89): C, 48.3; H, 3.6; Found: C, 48.1; H, 3.8. IR (KBr pellet, cm−1): 2922 (w), 1624(s), 1503(m), 1401(s), 1230(s), 1161(m), 1012(m), 873(m), 779(m), 664(w), 478(w).
Synthesis of [Cu2(oba)2(dmf)(C2H5OH)]·(dmf)2(H2O) (MCF-22·guest)
An aqueous solution (5 mL) of Cu(NO3)2·3H2O (0.121 g, 0.5 mmol) was added to a H2oba (0.133 g, 0.5 mmol) in a dmf–ethanol mixture (4
:
1, 25 mL) in the presence of benzimidazole (0.031 g, 0.25 mmol). The resulting solution was filtered, and allowed to stand at room temperature. Blue prism single crystals suitable for X-ray analysis were obtained after several days (0.152 g, yield ca. 63% based on Cu). Anal. calcd (%) for C39H45Cu2N3O15 (922.88): calcd. C, 50.8; H, 4.9; N, 4.6%; Found: C, 50.1; H, 4.6; N, 5.2%. IR (KBr pellet, cm−1): 3448 (w), 1668(m), 1612(s), 1500(m), 1396(vs), 1246(vs), 1159(s), 874(s), 776(s), 664(m), 474(m).
Sorption measurements
Sorption isotherms for N2 and CO2 gas was measured with an automatic volumetric sorption apparatus (BELSORP-max, Bel Japan) at 77 and 195 K, respectively, and the sorption isotherms for organic vapors were measured with an automatic gravimetric sorption apparatus (IGA-003 series, Hiden Isochema Ltd) at 298 K.
X-Ray diffraction data were recorded on a Bruker SMART Apex CCD system with graphite-monochromated Mo Kα radiation (λ = 0.71073 Å). Absorption corrections were applied by SADABS. The structures were solved by direct methods and refined by full-matrix least-squares techniques on F2 using the SHELXTL program package.14 All non-hydrogen atoms were refined with anisotropic displacement parameters except for the disordered solvent molecules. MCF-22·guest includes a large region of disordered solvent molecules, which could not be modeled as discrete atomic sites. Instead, PLATON/SQUEEZE15 was employed to calculate the diffraction contribution of the guest molecules and, thereby, to produce a set of guest-free diffraction intensities. The final chemical formula was calculated from the SQUEEZE results combined with TGA and elemental analysis. Statistics prior to treatment of data with SQUEEZE were R1 = 0.0794 (I > 2σ(I)), wR2 = 0.2612 (all data) and S = 1.089. Crystal data and details of data collections and refinements for MCF-21 and MCF-22·guest are summarized in Table 1. CCDC reference numbers 688066–688067 for MCF-21 and MCF-22·guest, respectively.†
Complexes |
MCF-21
|
MCF-22·guest
|
R
1 = Σ||FO| − |FC||/Σ|FO|,wR2 = {∑w(|FO| − |FO|)2/∑wFO2}1/2.
|
Formula |
C16H14CuO6S |
C39H45Cu2N3O15 |
FW
|
397.87 |
922.88 |
T/K |
293(2) |
150(2) |
Space group |
P21/c |
C2/c |
a/Å |
12.0877(15) |
30.8976(12) |
b/Å |
16.227(2) |
16.0853(5) |
c/Å |
9.2338(11) |
20.9200(6) |
β/° |
109.345(2) |
124.480(2) |
V
/Å3 |
1709.0(4) |
8570.6(5) |
Z
|
4 |
8 |
D
c/g cm−3 |
1.546 |
1.176 |
µ/mm−1 |
1.428 |
1.042 |
Reflns collected/unique |
3324/2397 |
7561/3647 |
R
int
|
0.0494 |
0.0682 |
R
1 (I > 2σ) |
0.0508 |
0.0622 |
wR
2 (all data) |
0.1237 |
0.1806 |
S
|
1.016 |
1.029 |
Results and discussion
Syntheses and structural characterizations
In general, interpenetration of frameworks producing only small pores or even no pores, especially those synthesized under hydrothermal conditions (high temperature, high pressure and water as the solvent), is considered an obstacle for contributing sorption properties to PCPs.13a,16 In contrast, mild reaction conditions at low temperature/pressure are favorable for more porous metal–organic frameworks. Actually, by using the same metal and organic ligand source, less porous and densely packed MCF-21 was prepared under solvothermal condition while the triply interpenetrated but more porous MCF-22·guest was prepared under a conventional solution reaction at room temperature. Besides, the difference in reaction temperature, solvents, and additives (such as benzimidazole in the synthesis of MCF-22·guest) may also play a role in the formation of the two framework-isomers.[11,17]
In MCF-21, each Cu(II) ion is square-pyramidally coordinated by four carboxylate oxygen atoms at the basal positions [Cu–O 1.964(3) Å] and one dmso molecule [Cu–O 2.145(3) Å] at the apical position (Fig. S1).† Each pair of Cu(II) ions are bridged by four carboxylate groups to generate a well-known paddle-wheel second building unit (SBU), while four adjacent SBUs are interlinked into a curved structural unit [Cu2(oba)2]4via the curved, bridging oba ligands (Fig. 1a), resulting in a corrugated 2-D network with a 44 topology perpendicular to the c-axis. Interestingly, these layers are π-stacked (ca. 3.3 Å) along the c-axis between the phenyl rings of oba ligands from the neighboring layers, affording a 1-D microchannel with an effective window size (excluding the van der Waals radii) of ca. 3.1 × 4.1 Å2,18 where dmso resides as a labile ligand (Fig. 1b). The solvent-accessible volume of MCF-21 is estimated to be 35.4% of the unit cell volume considering the removal of dmso.19
![Perspective views of the [Cu2(oba)2]4 structural unit (a) and stacking of two adjacent layers (b) in MCF-21 (solvent ligands have been omitted for clarity).](/image/article/2009/CE/b813226g/b813226g-f1.gif) |
| Fig. 1 Perspective views of the [Cu2(oba)2]4 structural unit (a) and stacking of two adjacent layers (b) in MCF-21 (solvent ligands have been omitted for clarity). | |
The solvent ligands occupying the channels can be removed at an elevated temperature without collapsing the host framework, as confirmed by TGA and PXRD studies (Fig. S2 and S3).† The TGA curve of MCF-21 reveals an initial weight loss of 20.6% from 232 to 285 °C corresponding to the removal of one dmso molecule per formula unit (calcd. 19.6%), resulting in the solvent-free microporous framework MCF-21′ with unsaturated metal centers (UMCs), which starts decomposition at 310 °C. After the complete removal of solvent at 250 °C for 2 h under a reduced pressure, the PXRD pattern of framework MCF-21′ coincides with that of the as-synthesized MCF-21, indicating that the framework is maintained after the removal of the dmso molecules, which can be attributed to the strong interlayer π-π stacking interaction in MCF-21.
In MCF-22·guest, each Cu(II) ion is also square-pyramidally coordinated by four carboxylate oxygen atoms at the basal positions with one dmf or ethanol molecule at the apical position (Fig. S4).† Compared with MCF-21, although MCF-22·guest consists of similar dinuclear Cu2 paddle-wheel SBUs, two types of ternary structural units are found. The first one, a four-member ring [Cu2(oba)2]4, has the same connectivity as that of MCF-21, but possesses a saddle-like conformation (Fig. 2a). The second one is a rhombic structural unit [Cu2(oba)2]8 with a very large cavity (ca. 49.5 × 7.6 Å2), which is formed by eight adjacent paddle-wheel SBUs through the bridging oba ligands (Fig. 2b).20 Subsequently, the extension of these two types of structural units produces a complicated 3-D network (Fig. 3a). While the Cu2 SBUs are simplified as nodes and the bridging oba ligands are simplified as the linkers, an unusual, uninodal four-connected lvt topology can be rationalized (Fig. 3b).21 Although the triple interpenetration is formed to avoid an extremely large void space (Fig. 4), MCF-22·guest still possesses a significant solvent-accessible space. Actually, disordered dmf and H2O guest molecules were found in the irregular rectangular channels with an estimated gate-size of 10.2 × 9.5 Å2 running along the c-axis, which intersect with rectangular channels of ca. 7.6 × 5.4 Å2 (occupied by ligated DMF and ethanol molecules) along the b-axis to produce a 2-D intersected microporous network (Fig. S5).† Taking into account the lattice solvent molecules as well as the coordinated solvent molecules, the solvent-accessible volume in MCF-22·guest is estimated to be 53.1% of the unit cell volume, among them 34.3% occupied by disordered guest molecules.19
![Views of the saddle-like [Cu2(oba)2]4(a) and the rhombic [Cu2(oba)2]8 (b) structural units in MCF-22·guest.](/image/article/2009/CE/b813226g/b813226g-f2.gif) |
| Fig. 2 Views of the saddle-like [Cu2(oba)2]4(a) and the rhombic [Cu2(oba)2]8 (b) structural units in MCF-22·guest. | |
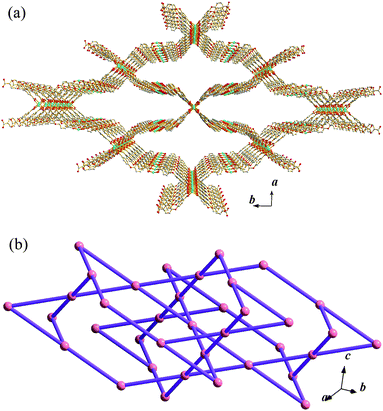 |
| Fig. 3 A single 3-D net viewed along the a-axis (a) and schematic illustration of the single 4-connected 3-D lvt net in MCF-22·guest (b). | |
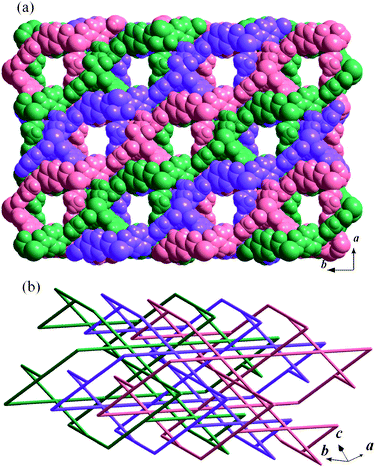 |
| Fig. 4 Perspective views of the crystal structure in MCF-22·guest along the c-axis (a) (disordered guest molecules have been omitted for clarity) and schematic illustration of the 3-fold interpenetrated lvt net in MCF-22·guest (b). | |
The TGA of MCF-22·guest shows the release of the lattice solvent molecules (16.1%, calc. 17.8%) over 30 to 180 °C to give a microporous phase MCF-22. With increasing temperature up to 255 °C, one dmf and one ethanol ligands per formula unit (13.7%, calcd. 12.9%) are liberated to afford the all-solvent-free phase [Cu(oba)] (MCF-22′) with UMCs. A plateau was observed from 255 to 280 °C in the TGA profile, indicating no further weight loss, while above 280 °C the framework starts to decompose rapidly (Fig. S7).† Since there exist only van der Waals interactions between the 3-fold interpenetrated frameworks, MCF-22 or MCF-22′ might potentially serve as a dynamic PCP material. Therefore, PXRD analyses were carried out to evaluate the structural information during the removal of lattice and coordinated solvent molecules. Assisted by TGA measurement, a sample of MCF-22·guest was heated to 100 °C under vacuum for 5 h, resulting in MCF-22 (Fig. S8), which has a similar PXRD pattern to that of MCF-22·guest (Fig. S6).† Therefore, MCF-22·guest can be regarded as a rigid framework. The PXRD pattern of MCF-22′ was apparently different from that of MCF-22, which may be ascribed to the structural transformation upon losing all coordinating solvent molecules.22 When MCF-22 was heated to 180 °C under vacuum for 4 h, the solvent-free MCF-22′ can be facilely obtained, which is confirmed by the disappearance of the C
O stretching peak of dmf at 1668 cm−1 and the TGA plot (Fig. S9).† Unfortunately, the poor single-crystallinity did not allow a single-crystal diffraction of MCF-22′. However, MCF-22 could be regenerated by treating MCF-22′ with a dmf–ethanol–water mixture, as verified by PXRD (Fig. S6).† Therefore, MCF-22 and MCF-22′ can be reversibly transferred, thus this Cu(II) carboxylate framework can be regarded as a guest-dependent dynamic PCP material.
To further examine porosity and reactivity, the sorption properties of MCF-21′, MCF-22 and MCF-22′ have been performed with N2 and CO2 gases as well as different volatile organic molecules. The sorption isotherm of N2 for MCF-21′ at 77 K reveals only surface adsorption (15 m2 g−1), indicating that N2 molecules cannot diffuse into the channel because of the smaller channel window size than the adsorbate. On the contrary, MCF-21′ adsorbs appreciable quantities of CO2 (27 cm3 g−1 at P/P0 = 0.98), giving a specific area of 157 m2 g−1 by analyzing the CO2 adsorption data (Fig. S11a,b).† The results can be explained by a size-exclusion behavior of the small channels in MCF-21′. MCF-21′ also shows a methanol sorption capacity of 70 mg g−1 at P/P0 = 0.83, which corresponds to the adsorption of approximately one methanol molecule per formula unit (Fig. S11c).† In addition, the desorption curve does not retrace the adsorption isotherm and shows hysteresis, implying that chemisorption occurs and the methanol molecules may directly ligate to the unsaturated Cu(II) centers.8h,11 Similar sorption behavior was also observed for other known PCPs.23
As shown in Fig. 5, the N2 and CO2sorptions of MCF-22 exhibit a reversible type I isotherm, characteristic of a microporous material. Fitting the N2 adsorption isotherm gives a BET (Brunauer–Emmett–Teller) surface area of 368 m2 g−1 and a Langmuir surface area of 442 m2 g−1. The pore volume is estimated to 0.157 cm3 g−1 by applying the Dubinin–Raduskhvich equation. The small hysteresis of the CO2 sorption isotherm may be resulted from the relatively strong adsorbent–adsorbate interactions. MCF-22 also exhibits certain adsorption capacity for organic vapors at 298 K and the adsorbed molecules per formula unit (at P/P0 = 0.83) are as follows: 1.7 for methanol, 1.2 for ethanol, 0.8 for acetone, 0.5 for benzene, as well as 0.2 for toluene, respectively (Fig. 6). Apparently, the organic vapor sorption isotherms of MCF-22 are not fully saturated, as seen, the second one increases on adsorption at around the P/P0 = 0.83. Such phenomena have also been widely reported.5
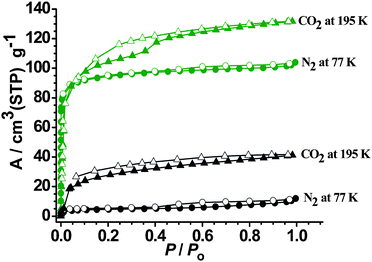 |
| Fig. 5 N2 and CO2 adsorption (solid) and desorption (open) isotherms for MCF-22 (olive) and MCF-22′ (black). | |
Interestingly, MCF-22′ basically exhibits no N2 sorption at 77 K (corresponding to just 6.3% of MCF-22), indicating that its framework structure is completely different from that of MCF-22. The CO2 adsorption capacity (40 cm3 g−1 at P/P0 = 0.9) for MCF-22′ at 195 K (Fig. 5) can be attributed to the smaller adsorbate size of CO2 (3.3 Å) as compared to that of N2 (3.64 Å). Therefore, MCF-22′ is nonporous for N2 at 77 K but slightly porous for CO2 at 195 K. These adsorption phenomena can be well associated with the structural dynamics between MCF-22 and MCF-22′. After removal of the coordinated ligands (dmf and C2H5OH) from MCF-22, coordinatively unsaturated Cu(II) centers are formed. Consequently, to accomplish coordination saturation and close-packing, the flexible, interpenetrating Cu(II) carboxylate frameworks can move toward each other to form the closed phase MCF-22′ locked by weak coordination bonds between the Cu(II) sites of one network and the carboxylate oxygen of another network. Nevertheless, the “locked” MCF-22′ cannot be opened by adsorbates with weak adsorbent–adsobate interactions. On the other hand, MCF-22′ should exhibit sorption capacity for other adsorbates with coordinating ability. This deduction is exactly confirmed by the methanol sorption isotherm of MCF-22′ at 298 K. As shown in Fig. 7, MCF-22′ shows a rather high adsorption capacity for methanol (92 mg g−1, at P/P0 = 0.83), corresponding to the adsorption of approximately 1.8 methanol molecules per formula unit. Furthermore, higher methanol adsorption capacity may be expected under higher vapor pressure since the methanol sorption isotherm of MCF-22′ is not fully saturated. Accordingly, on exposure of MCF-22′ to the methanol vapor for 2 d at room temperature, the TGA curve of the methanol resolved MCF-22′ shows that 6.4 methanol molecules were adsorbed per formula unit (Fig. S10),† which signifies that two methanol molecules were directly ligated to the vacant Cu(II) coordination sites and the residue was capsuled in the cavities. The methanol adsorption experiment indicated that the relatively nonporous Cu(II) carboxylate framework MCF-22′ can be ‘unlocked’ by not only the original keys (dmf and C2H5OH) but also by other coordinating guests. Therefore, the structure and porosity of this Cu(II) carboxylate framework can be systematically modified by a rich family of coordinating guests. As exemplified by our N2 and CO2 sorption experiments, inserting dmf and C2H5OH into the Cu(II) carboxylate framework does significantly increase its gas sorption capacity.
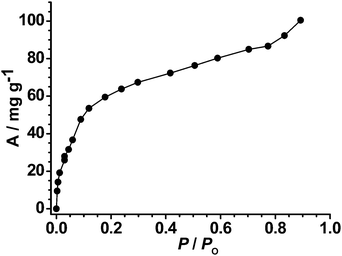 |
| Fig. 7 Temperature methanol adsorption isotherm of MCF-22′ at 298 K. P0 is the saturated vapor pressure at 298 K. | |
Conclusions
In conclusion, two framework-isomeric PCPs with two uninodal four-connected topologies were successfully isolated and structurally characterized, in which the densely packed structure of MCF-21 was obtained by a solvothermal reaction, while a triply interpenetrated but loose and porous framework MCF-22·guest was isolated by a conventional solution reaction. Among them, MCF-21 can retain a rigid framework and selectively adsorb CO2 over N2, whereas MCF-22·guest exhibits the unique features of either permanent porosity upon removal of lattice solvent molecules or a guest-responsive dynamic character upon removal of all the lattice and coordinated solvent molecules, which may be expected for finding useful application in molecule separation or sensors.
Acknowledgements
This work was supported by the NSFC (No. 20531070 and 20821001), “973 Program” (2007CB815302), and the Science and Technology Department of the Guangdong Province (No. 04205405).
References
-
(a) S.-I. Noro, S. Kitagawa, M. K. Kondo and K. Seki, Angew. Chem., Int. Ed., 2000, 39, 2081 CrossRef;
(b) N. L. Rosi, J. Eckert, M. Eddaoudi, D. T. Vodak, J. Kim, M. O'Keeffe and O. M. Yaghi, Science, 2003, 300, 1127 CrossRef CAS;
(c) G. Férey, C. Mellot-Draznieks, C. Serre, F. Millange, J. Dutour, S. Surblé and I. Margiolaki, Science, 2005, 309, 2040 CrossRef CAS;
(d) X. C. Huang, Y. Y. Lin, J. P. Zhang and X. M. Chen, Angew. Chem., Int. Ed., 2006, 45, 1557 CrossRef CAS;
(e) M. Dincă, A. F. Yu and J. R. Long, J. Am. Chem. Soc., 2006, 128, 8904 CrossRef CAS.
-
(a) L. Pan, D. H. Olson, L. R. Ciemnolonski, R. Heddy and J. Li, Angew. Chem., Int. Ed., 2006, 45, 616 CrossRef CAS;
(b) D. N. Dybtsev, H. Chun, S. H. Yoon, D. Kim and K. Kim, J. Am. Chem. Soc., 2004, 126, 32 CrossRef CAS;
(c) L. Alaerts, C. E. A. Kirschhock, M. Maes, M. A. van der Veen, V. Finsy, A. Depla, J. A. Martens, G. V. Baron, P. A. Jacobs, J. F. M. Denayer and D. E. De Vos, Angew. Chem., Int. Ed., 2007, 46, 4293 CrossRef CAS;
(d) J. Y. Lee, D. H. Olson, L. Pan, T. J. Emge and J. Li, Adv. Funct. Mater., 2007, 17, 1255 CrossRef CAS.
-
(a) A.-G. Hu, H. L. Ngo and W.-B. Lin, J. Am. Chem. Soc., 2003, 125, 114901;
(b) J. S. Seo, D. Whang, H. Lee, S. I. Jun, J. Oh, Y. Jeon and K. Kim, Nature, 2000, 404, 982 CrossRef CAS;
(c) R.-Q. Zou, H. Sakurai, S. Han, R.-Q. Zhong and Q. Xu, J. Am. Chem. Soc., 2007, 129, 8402 CrossRef CAS.
-
(a) T. K. Maji, R. Matsuda and S. Kitagawa, Nat. Mater., 2007, 6, 142 CrossRef CAS;
(b) R. Custelcean and B. A. Moyer, Eur. J. Inorg. Chem., 2007, 2007, 1321 CrossRef;
(c) T. Uemura, S. Horike and S. Kitagawa, Chem.–Asian J., 2007, 1, 36.
-
(a) O. M. Yaghi, M. O'Keeffe, N. W. Ockwig, H. K. Chae, M. Eddaoudi and J. Kim, Nature, 2003, 423, 705 CrossRef CAS;
(b) S. Kitagawa, R. Kitaura and S.-I. Noro, Angew. Chem., Int. Ed., 2004, 43, 2334 CrossRef CAS;
(c) C. N. R. Rao, S. Natarajan and R. Vaidhyanathan, Angew. Chem., Int. Ed., 2004, 43, 1466 CrossRef CAS;
(d) M. J. Rosseinsky, Microporous Mesoporous Mater., 2004, 73, 3 CrossRef CAS;
(e) G. Férey, C. Mellot-Draznieks, C. Serre and F. Millange, Acc. Chem. Res., 2005, 38, 217 CrossRef CAS.
- B. Moulton and M. J. Zaworotko, Chem. Rev., 2001, 101, 1629 CrossRef CAS.
-
(a) S.-Q. Ma, D.-F. Sun, M. Ambrogio, J. A. Fillinger, S. Parkin and H.-C. Zhou, J. Am. Chem. Soc., 2007, 129, 1858 CrossRef CAS;
(b) H. Chun and J. Moon, Inorg. Chem., 2007, 46, 4371 CrossRef CAS;
(c) E. Tynan, P. Jensen, P. E. Kruger and A. C. Lees, Chem. Commun., 2004, 776 RSC;
(d) B. Rather, B. Moulton, R. D. Bailey Walsh and M. J. Zaworotko, Chem. Commun., 2002, 694 RSC;
(e) J.-P. Zhang and S. Kitagawa, J. Am. Chem. Soc., 2008, 130, 907 CrossRef CAS;
(f) C.-C. Wang, W.-Z. Lin, W.-T. Huang, M.-J. Ko, G.-H. Lee, M.-L. Ho, C.-W. Lin, C.-W. Shih and P.-T. Chou, Chem. Commun., 2008, 1299 RSC.
-
(a) S. Kitagawa and K. Uemura, Chem. Soc. Rev., 2005, 34, 109 RSC;
(b) G. J. Halder and C. J. Kepert, Aust. J. Chem., 2006, 59, 597 CrossRef CAS;
(c) M. P. Suh, J. W. Ko and H. J. Choi, J. Am. Chem. Soc., 2002, 124, 10976 CrossRef CAS;
(d) D. N. Dybtsev, H. Chun and K. Kim, Angew. Chem., Int. Ed., 2004, 43, 5033 CrossRef CAS;
(e) C. Serre, F. Millange, C. Thouvenot, M. Nogués, G. Marsolier, D. Louër and G. Férey, J. Am. Chem. Soc., 2002, 124, 13519 CrossRef CAS;
(f) X.-N. Cheng, W.-X. Zhang, Y.-Y. Lin, Y.-Z. Zheng and X.-M. Chen, Adv. Mater. (Weinheim, Ger.), 2007, 19, 1494 CrossRef CAS;
(g) K. Biradha and M. Fujita, Angew. Chem., Int. Ed., 2002, 41, 3392 CrossRef CAS;
(h) T. K. Maji, M. Ohba and S. Kitagawa, Inorg. Chem., 2005, 44, 9225 CrossRef CAS.
- J.-P. Zhang and X.-M. Chen, J. Am. Chem. Soc., 2008, 130, 6010 CrossRef CAS.
-
(a) P. L. Llewellyn, S. Bourrelly, C. Serre, Y. Filinchuk and G. Férey, Angew. Chem., Int. Ed., 2006, 45, 7751 CrossRef CAS;
(b) T. Haneda, M. Kawano, T. Kawanchi and M. Fujita, J. Am. Chem. Soc., 2008, 130, 1578 CrossRef CAS.
- D.-X. Xue, Y.-Y. Lin, X.-N. Cheng and X.-M. Chen, Cryst. Growth Des., 2007, 7, 1332 CrossRef CAS.
-
(a) X.-M. Chen and G.-F. Liu, Chem.–Eur. J., 2002, 8, 4811 CrossRef CAS;
(b) Z.-B. Han, X.-N. Cheng and X.-M. Chen, Cryst. Growth Des., 2005, 5, 695 CrossRef CAS;
(c) J. Tao, X. Yin, R.-B. Huang and L.-S. Zheng, Main Group Met. Chem., 2002, 25, 523 CAS.
-
(a) X.-L. Wang, C. Qin, E.-B. Wang, Y.-G. Li and Z.-M. Su, Chem. Commun., 2005, 5450 RSC;
(b) X.-L. Wang, C. Qin, E.-B. Wang, Y.-G. Li, Z.-M. Su, L. Xu and L. Carlucci, Angew. Chem., Int. Ed., 2005, 44, 582;
(c) X.-L. Wang, C. Qin, E.-B. Wang and Z.-M. Su, Chem.–Eur. J., 2006, 12, 2680 CrossRef CAS;
(d) M. Kondo, Y. Irie, Y. Shimizu, M. Miyazawa, H. Kawaguchi, A. Nakamura, T. Naito, K. Maeda and F. Uchida, Inorg. Chem., 2004, 43, 6139 CrossRef.
-
(a)
SMART Version 5.625, SAINT+
Version 6.22. Bruker Analytical X-ray System, Inc., Madison, Wisconsin, USA, 2001 Search PubMed;
(b)
G. M. Sheldrick, SHELXTL, Version 6.10. Bruker Analytical X-ray System, Siemens Industrial Automation Inc.,Madison, Wisconsin, USA, 2000 Search PubMed.
- A. L. Spek, J. Appl. Crystallogr., 2003, 36, 7 CrossRef CAS.
-
(a) J. Lu, C. Yu, T. Niu, T. Paliwala, G. Crisci, F. Somosa and A. J. Jacobson, Inorg. Chem., 1998, 37, 4637 CrossRef CAS;
(b) C. Livage, N. Guillou, J. Marrot and G. Ferey, Chem. Mater., 2001, 13, 4387 CrossRef CAS;
(c) S.-I. Noro, R. Kitaura, M. Kondo, S. Kitagawa, T. Ishii, H. Matsuzaka and M. Yamashita, J. Am. Chem. Soc., 2002, 124, 2568 CrossRef CAS;
(d) P. Ayyappan, O. R. Evans and W. Lin, Inorg. Chem., 2002, 41, 3328 CrossRef CAS;
(e) Y.-B. Dong, R. C. Layland, N. G. Pschirer, M. D. Smith, U. H. F. Bunz and H.-C. zur Loye, Chem. Mater., 1999, 11, 1413 CrossRef CAS;
(f) M.-L. Tong, H.-J. Chen and X.-M. Chen, Inorg. Chem., 2000, 39, 2235 CrossRef CAS.
-
(a) J.-P. Zhang, Y.-Y. Lin, X.-C. Huang and X.-M. Chen, Dalton Trans., 2005, 3681 RSC;
(b) J.-P. Zhang, Y.-Y. Lin, X.-C. Huang and X.-M. Chen, Chem. Commun., 2005, 1258 RSC;
(c) J.-P. Zhang and X.-M. Chen, Chem. Commun., 2006, 1689 RSC.
- All pore sizes reported in this paper are determined by considering van der waals radii for constituent atoms (C 1.7, H 1.2, O 1.52, Cu 1.40 Å) A. Bondi, J. Phys. Chem., 1964, 68, 441 Search PubMed.
- A. L. Spek, Acta Crystallogr., Sect. A: Found. Crystallogr., 1990, 46, C34.
- M. Eddaoudi, J. Kim, D. Vodak, A. Sudik, J. Wachter, M. O'keeffe and O. M. Yaghi, Proc. Natl. Acad. Sci. U. S. A., 2002, 99, 4900 CrossRef CAS.
- O. D. Friedrichs, M. O'Keeffe and O. M. Yaghi, Acta Crystallogr., Sect. A: Found. Crystallogr., 2003, 59, 515 CrossRef.
- B.-L. Chen, C.-D. Dai, J. Yang, D. S. Contreras, Y. L. Clancy, E. B. Lobkovsky, O. M. Yaghi and S. Dai, Angew. Chem., Int. Ed., 2006, 45, 1390 CrossRef CAS.
-
(a) N. L. Rosi, M. Eddaoudi, J. Kim, M. O'Keeffe and O. M. Yaghi, Angew. Chem., Int. Ed., 2002, 41, 284 CrossRef;
(b) T. K. Maji, K. Uemura, H.-C. Chang, R. Matsuda and S. Kitagawa, Angew. Chem., Int. Ed., 2004, 43, 3269 CrossRef CAS.
Footnote |
† Electronic supplementary information (ESI) available: TGA curves, PXRD, N2, CO2 and methanol sorption isotherms of MCF-21′, additional structural plot. CCDC reference numbers 688066 and 688067. For ESI and crystallographic data in CIF or other electronic format see DOI: 10.1039/b813226g |
|
This journal is © The Royal Society of Chemistry 2009 |