DOI:
10.1039/B811725J
(Paper)
CrystEngComm, 2009,
11, 109-117
Synthesis, structures and properties of Mn(II) coordination frameworks based on R-isophthalate (R = –CH3 or –C(CH3)3) and various dipyridyl-type co-ligands†
Received
9th July 2008
, Accepted 17th September 2008
First published on 8th October 2008
Abstract
Five new Mn(II) metal–organic coordination polymers, [Mn(tbip)(bipy)]n (1), {[Mn(tbip)(dpe)]·1.5H2O}n (2), [Mn(tbip)(bpa)]n (3), [Mn(mip)(bpa)]n (4) and {[Mn3(mip)2(Hmip)2(bpp)2(H2O)2]·2H2O}n (5), were prepared through hydrothermal reactions of Mn(II) acetate with H2tbip (H2tbip = 5-tert-butyl isophthalic acid) or H2mip (H2mip = 5-methylisophthalic acid) in the presence of different dipyridyl co-ligands (bipy = 4,4′-bipyridine, dpe = 1,2-bi(4-pyridyl)ethene, bpa = 1,2-bi(4-pyridyl)ethane and bpp = 1,3-di(4-pyridyl)propane). All these compounds were characterized by elemental analysis, IR spectroscopy and X-ray single-crystal diffraction. The structures of 1 and 2 are constructed of [Mn2(tbip)2]n layers, which consist of alternate left- and right-handed helical chains, and further pillared by bipy or dpe ligands into a 3D porous framework. Interestingly, there are two different sizes of pores in 1 and 2. From the topology point of view, both complexes 1 and 2 exhibit the rare rob network based on the dimeric [Mn(COO)]2 subunits as the 6-connected nodes. The ribbon-like chains having 8- and 16-membered rings in 3 and 4 result from dimeric Mn(II) units bridged by H2tbip or H2mip ligands, which are connected by the flexible dpa into 2D open layers. Complex 5 contains rare isophthalato-bridged trimanganese clusters as building units, which are further linked by mip anions to form a one-dimensional ladder-like chain with less common unidentate coordinated bpp decorated as pendants. The thermal stabilities and X-ray powder diffraction studies indicate that the microporous framework of 2 can keep stable even after the loss of guest water molecules. In addition, dominant antiferromagnetic coupling was observed in compounds 1–3 and 5.
Introduction
Metal–organic hybrid materials of paramagnetic metal ions exhibiting extended structures are currently of great interest in the field of molecular magnetism and materials chemistry due to their fascinating structural diversities and potential application as functional materials.1–6 A commonly used strategy in the construction of such extended architectures is to employ appropriate bridging ligands, which can transmit the magnetic interactions as well as bind several metal centers of specific coordination geometry. In this respect, phenyldicarboxylates are frequently used in the design of polymeric complexes as efficient linkers/spacers.7,8 Although magnetic interactions transmitted through phenyldicarboxylate bridges are usually weak, the magnitude and nature of the coupling interaction can be influenced by a series of factors, such as the separation of the metal centers, bridging modes and the dihedral angles between the carboxylate planes and the phenyl ring.9 Among the first-row transition-metal ions, the high-spin Mn(II) contains five unpaired electrons and is quite oxophilic, and thus the assembly of Mn(II) with an organic ligand containing the carboxylic group is inclined to the formation of larger clusters and extended solids.10 Moreover, carboxylato-bridged Mn(II) complexes are known to exist at the active centers of some manganese-containing enzymes.11 Due to these novel properties, increasing attention has been devoted to the construction of manganese-carboxylate frameworks. Recently, isophthalic acid has been used to construct one- and two- dimensional polymeric Mn(II) complexes where isophthalic acid can function as a mediator for transmitting the exchange interactions between the paramagnetic metal centers.12–17
On the other hand, exo-bidentate rodlike N,N′-donor ligands, such as 4,4′-bipyridine, can have significant influence on the assembly systems of multicarboxylate ligands and metal centers. Its derivatives dpe (1,2-bi(4-pyridyl)ethene), bpa (1,2-bi(4-pyridyl)ethane) and bpp (1,3-di(4-pyridyl)propane) with certain spacers between the two 4-pyridyl rings may also lead to fascinating architectures with interesting properties. Related results are quite useful for understanding the coordination-driven assembly and recognition process.18–20
Very recently, we have initiated to utilize a multifunctional amino acid derivative, namely N-[(3-carboxyphenyl)-sulfonyl]glycine whose structure is similar to isophthalic acid except for the nitrogen atom and oxygen atoms of the sulfonate groups, to create a series of unusual metal–organic frameworks.21 To further probe the influence of systematic variations of the ligand structure and metal ions on the overall molecular architectures, we extend the building blocks from N-[(3-carboxyphenyl)-sulfonyl]glycine to 5-tert-butyl isophthalic acid and 5-methylisophthalic acid. In contrast to isophthalic acid, H2tbip and H2mip not only contain two bridging carboxylate moieties but also possess the electron-donating –CH3– or –C(CH3)3 substituents, which can generate distinct complexes to those of the R-isophthalate (R = –H, –NO2, –OH) ligands.22 Here we report five novel coordination polymers by self-assembly of H2tbip or H2mip and Mn(II) salts with bipy and other N-donor co-ligands, respectively. Their physical properties such as thermal, PXRD, and magnetic properties have also been investigated.
Experimental
Materials and physical measurements
All reagents used in the syntheses were of analytical grade. Elemental analyses for carbon, hydrogen and nitrogen atoms were performed on a Vario EL III elemental analyzer. The infrared spectra (4000∼600 cm−1) were recorded by using KBr pellet on an Avatar™ 360 E. S. P. IR spectrometer. Thermogravimetric analyses (TG) were carried out on a STA449C integration thermal analyzer. The PXRD patterns were recorded with a Rigaku D/Max 3III diffractometer with a scanning rate of 4° min−1. Variable-temperature magnetic susceptibilities were measured using a MPMS-7 SQUID magnetometer. Diamagnetic corrections were made with Pascal's constants for all constituent atoms.
Preparation of complexes 1–5
[Mn(tbip)(bipy)]n (1).
A mixture of H2tbip (0.104 mmol, 23.2 mg), bipy (0.083 mmol, 15.9 mg), Mn(OAc)2·4H2O (24.1 mg, 0.098 mmol), KOH (0.10 mmol, 5.6 mg) and H2O (16 mL) was placed in a Teflon-lined stainless steel vessel, heated to 130 °C for 3 d, and then cooled to room temperature over 24 h. Yellow block crystals of 1 were obtained. Yield: 0.020 g, 31%. Elemental analysis (%): calcd for C22H20MnN2O4: C 61.26, H 4.67, N 6.49; found: C 61.21, H 4.59, N 6.43. IR (cm−1): 3442 m, 2946 m, 1602 s, 1579 m, 1439 m, 1420s, 1374 m, 1220 m, 813 m.
{[Mn(tbip)(dpe)]·1.5H2O}n (2).
2 was synthesized in a similar way to that described for 1, except that bipy was replaced by dpe. Yield: 0.032 g, 36%. Elemental analysis (%): calcd for C24H25MnN2O5.5: C 59.51, H 5.20, N 5.78; found: C 59.44, H 5.11, N 5.71. IR (cm−1): 3399 m, 2964 m, 1698 s, 1606 m, 1563 m, 1406 m, 1249 m, 1016 m, 829 m, 771m.
[Mn(tbip)(bpa)]n (3).
3 was synthesized in a similar way to that described for 1, except that bipy was replaced by bpa. Yield: 0.024 g, 30%. Elemental analysis (%): calcd for C24H24MnN2O4: C 62.75, H 5.27, N 6.10; found: C 62.71, H 5.41, N 6.03. IR (cm−1): 3415 m, 2960 m, 1613 s, 1499 m, 1382 m, 1272 m, 1013 m, 824 m, 721m.
[Mn(mip)(bpa)]n (4).
A mixture of H2mip (0.097 mmol, 17.7 mg), bpa (0.101 mmol, 18.4 mg), Mn(OAc)2·4H2O (24.1 mg, 0.098 mmol), KOH (0.10 mmol, 5.6 mg) and H2O (16 mL) was placed in a Teflon-lined stainless steel vessel, heated to 130 °C for 3 d, and then cooled to room temperature over 24 h. Yellow block crystals of 4 were obtained. Yield: 0.157g, 20%. Elemental analysis (%): calcd for C21H18MnN2O4: C 60.44, H 4.35, N 6.71; found: C 60.48, H 4.39, N 6.62. IR (cm−1): 3438 m, 2950 m, 1617 s, 1579 m, 1381 m, 1212 m, 1047 m, 821 m, 768 m.
{[Mn3(mip)2(Hmip)2(bpp)2(H2O)2]·2H2O}n (5).
5 was synthesized in a procedure analogous to that of 4 except that bpa was replaced by bpp. Yellow block crystals of 5 were obtained. Yield: 0.023 g, 37%. Elemental analysis (%): calcd for C62H62Mn3N4O20: C 55.24, H 4.64, N 4.16; found: C 55.29, H 4.56, N 4.27. IR (cm−1): 3353 m, 2944 m, 1718 m, 1614 s, 1580 m, 1377 m, 1219 m, 1063 m, 804 m, 771m.
Single crystal X-ray diffraction analyses of the five compounds were carried out on a Bruker SMART APEX II CCD diffractometer equipped with a graphite monochromated Mo Kα radiation (λ = 0.71073 Å) by using ϕ/ω scan technique at room temperature. The structures were solved by direct methods with SHELXS-97. The hydrogen atoms were assigned with common isotropic displacement factors and included in the final refinement by use of geometric restrains. A full-matrix least-squares refinement on F2 was carried out using SHELXL-97. In the structures of 2 and 3, the disordered methyl carbon atoms of tert-butyl groups were restrained in order to obtain reasonable thermal parameters. The crystallographic data and selected bond lengths and angles for 1–5 are listed in Table 1 and Tables S1–S5 (ESI).†Crystallographic data for the structural analysis have been deposited with the Cambridge Crystallographic Data Center. CCDC reference numbers: 694054–694058.
Compound |
1
|
2
|
3
|
4
|
5
|
Formula |
C44H40Mn2N4O8 |
C48H50Mn2N4O11 |
C24H24MnN2O4 |
C21H18MnN2O4 |
C62H62Mn3N4O20 |
FW
|
862.68 |
968.80 |
459.39 |
417.31 |
1347.98 |
Temperature |
291(2) |
293(2) |
291(2) |
291(2) |
291(2) |
Crystal system |
Monoclinic |
Monoclinic |
Triclinic |
Triclinic |
Triclinic |
Space group |
C2/c |
C2/c |
P-1
|
P-1
|
P-1
|
Unit cell dimensions/Å, ° |
a = 18.438(2) |
a = 24.291(3) |
a = 10.3090(12) |
a = 9.934(11) |
a = 9.8326(13) |
b = 14.600(16) |
b = 13.558 (2) |
b = 11.4973(14) |
b = 10.242(11) |
b = 10.9290(15) |
c = 15.503(17) |
c = 16.280(2) |
c = 11.4980(14) |
c = 11.064(12) |
c = 16.539(2) |
β = 90.140(10) |
β = 110.495(2) |
α = 73.4920(10) |
α = 78.351(12) |
α = 71.419(2) |
|
|
β = 71.7230(10) |
β = 82.391(13) |
β = 79.114(2) |
|
|
γ = 76.4970(10) |
γ = 67.257(12) |
γ = 70.6480(10) |
V/Å3 |
4173.2(8) |
5022.5(12) |
1225.0(3) |
1014.9(19) |
1582.5(4) |
Z
|
8 |
8 |
2 |
2 |
1 |
ρ/g cm−3 |
1.373 |
1.281 |
1.245 |
1.366 |
1.414 |
F(000)
|
1784 |
2016 |
478 |
430 |
697 |
GOF |
1.083 |
1.052 |
1.044 |
1.063 |
1.022 |
R
1, wR2 [I > 2σ (I)] |
0.0376,0.1058 |
0.1318, 0.2914 |
0.0519, 0.1385 |
0.0404, 0.1121 |
0.0327, 0.0812 |
R
1, wR2 (all data) |
0.0398, 0.1079 |
0.1949, 0.3313 |
0.0640, 0.1525 |
0.0532, 0.1221 |
0.0407, 0.0871 |
Results and discussion
Hydrothermal synthesis has been widely employed to produce new materials with diverse structural architectures although the mechanism is not completely clear so far. This method can minimize the problems associated with ligand solubility and enhance the reactivity of reactants. Our first attempts to react Mn(II) salts with H2tbip or H2mip directly by hydrothermal methods gave only some precipitates or microcrystalline products that are unsuitable for single crystal X-ray diffraction analysis, which may be due to the fast rate of polymerization of Mn(II) ions with H2tbip or H2mip. Considering that the introduction of an N-containing auxiliary ligand may tune the structures of the resultant metal–organic complexes, we chose bipy as the ancillary ligand in this system. Fortunately, 1 was successfully isolated in its crystalline form with good yield. In order to perform a systematic investigation of the Mn(II) complexes with H2tbip or H2mip, we change bipy by other N-donor ligands (dpe, bpa and bpp) to obtain compounds 2–5. Compounds 1–5 are air stable, insoluble in common organic solvents, and can retain their crystalline integrity at ambient conditions for a considerable length of time.
Structural descriptions
[Mn(tbip)(bipy)]n (1).
The asymmetric unit contains one Mn (II) ion, one tbip dianion and one bipy. As shown in Fig. 1, the Mn1 ion has a slightly distorted octahedral environment (MnO4N2) consisting of two transnitrogen atoms from two different bipy molecules and four oxygen atoms from three different tbip ligands. The Mn–N bond lengths are 2.298(2) and 2.331(2) Å and the Mn–O bond lengths are in the range of 2.093(2)–2.480(2) Å. The tbip ligand in 1 acts as a tetradentate ligand, in which one carboxylate group adopts a chelating-bidentate mode, while the other exhibits a bis-monodentate bridging mode (Scheme 1a). Thus, the Mn1 atoms are linked by tbip to form the alternate left- and right-handed helical chains running along the crystallographic 21 axis with a pitch of 14.6 Å, as shown in Fig. 2. Two Mn(II) ions form a carboxylate-bridged dimer and the Mn(II) dimers are present between two helicates to make a 2D layered structure (Fig. 2 and Fig. S1a, ESI†). Interestingly, the adjacent helical layers are connected by bipy pillars to generate a novel 3D open framework featuring cavities along the b axis with dimensions of 5.34 × 3.75 Å2. When viewed along the c axis, such a 3D open framework is also a microporous coordination polymer containing rectangular-shaped channels with a cross-section of ca. 8.96 × 4.6 Å2 (measured by the Mn–Mn distance), as indicated in Fig. 3, Fig. S1b and S1c, ESI.† The diameter of these channels is too small to hold any guest molecules. The effective free volume of 1 was calculated by PLATON analysis as 6.2% of the crystal volume (256.7 out of the 4173.2 Å3 unit cell volume).
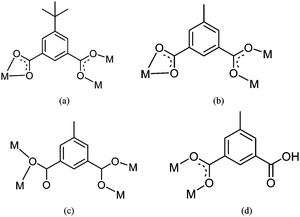 |
| Scheme 1 Coordination modes of H2tbip and H2mip observed in 1–5. | |
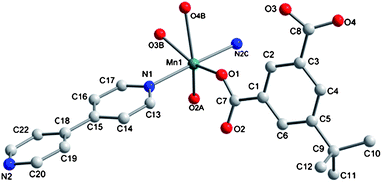 |
| Fig. 1 Coordination environment of the Mn (II) ion in 1. Hydrogen atoms are omitted for clarity. Symmetry codes: A, 0.5 − x, 1.5 − y, −z; B, 0.5 − x, −0.5 + y, 0.5 − z; C, −0.5 + x, 0.5 + y, z. | |
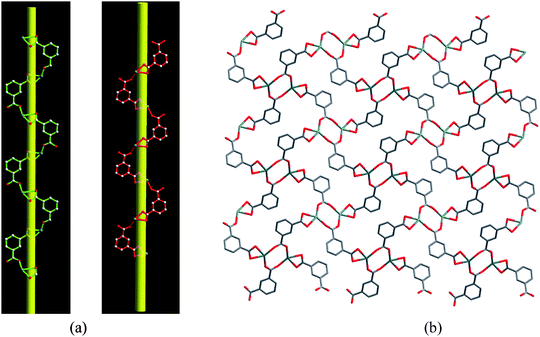 |
| Fig. 2 (a) Perspective view of the left-handed and right-handed helical chains in 1. (b) 2D helical layer in 1. | |
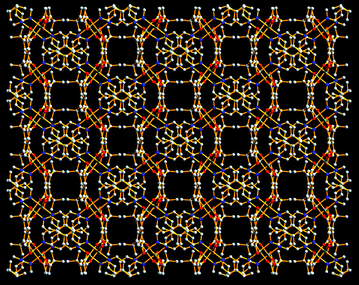 |
| Fig. 3 Perspective view of the 3D network in 1. | |
From the viewpoint of network topology, each tbip anion in 1 is three-connected by linking to three Mn(II) ions with a vertex symbol of (4.6.8), and each Mn(II) ion is five-connected to three tbip as well as two adjacent Mn(II) centers viabipy with a vertex symbol of (4.6.62.62.62.63.82.82.82.1018]. Thus, a 3-D (3,5)-connected network with the Schläfli symbol of (4.6.8)(4.65.83.10) is formed, which represents a new network topology. To further reduce this 3-D structure, the familiar dimeric [Mn(COO)]2 units can be considered as the nodes. In this condition, a uniform 3-D 6-connected rob network with the Schläfli symbol of (48.66.8) is generated, as shown in Fig. 4.
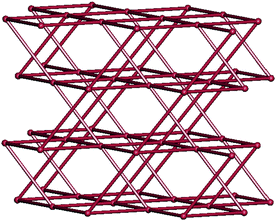 |
| Fig. 4 Schematic view of the net topology of 1. | |
{[Mn(tbip)(dpe)]·1.5 H2O}n (2).
From the above results, it may be anticipated that the longer pillar linker dpe would lead to the larger pores. X-Ray crystallography reveals that complexes 1 and 2 show similar 3-D network structures which are topologically equivalent. The fundamental building unit of 2 contains one Mn(II) ion, one tbip dianion, one dpe, and one and a half-lattice water molecules (Fig. 5). As a consequence, the dpe ligand acts as a pillar to support the 2D helical layer to lead to an extended 3D open framework with channels of 4.06 × 9.1 Å2 along the b axis and of 12.62 × 9.8 Å2 along the c axis. which are occupied by water molecules (Fig. 6 and Fig. S2, ESI).† Interestingly, the lattice water molecules within the channels form straight-chain water arrays (O⋯O distances: 2.85 and 2.96 Å, respectively). The effective free volume of 2 was calculated by PLATON analysis as 15% of the crystal volume (754.7 out of the 5022.5 Å3 unit cell volume). To the best of our knowledge, compounds 1 and 2 represent the first example of 3D Mn coordination polymers constructed from isophthalate or its derivatives.
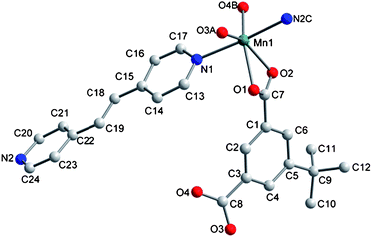 |
| Fig. 5 (a) Coordination environment of the Mn (II) ion in 2. Hydrogen atoms are omitted for clarity. Symmetry codes: A, x, 1 − y, − 0.5 + z; B, 0.5 − x, −0.5 + y, 0.5 − z; C, 0.5 + x, − 0.5 + y, z. | |
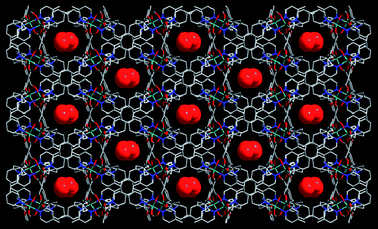 |
| Fig. 6 Projection of 2 along the c axis showing the cavities encapsulating free water molecules. | |
When isop (isop = isophthalate) and Mn(II) salt are selected as the starting materials, [Mn(isop)(bipy)]n (6) and {[Mn(isop)(dpe)]·0.5dpe·H2O}n (7) were generated12,13 in which the Mn(II) center is in a distorted octahedral geometry and bipy or dpe also behaves as a bridging ligand to link two Mn(II) ions, similar to that in 1 and 2. However, both 6 and 7 show the 2D double-layer structures made of parallel [Mn(ip)]n double chains that are cross-linked by bipy or dpe ligands. This structural difference between 1/2 and 6/7 may be ascribed to the synergetic electron-donating and steric effects of the –C(CH3)3group in H2tbip.
[Mn(tbip)(bpa)]n (3).
The asymmetric unit of 3 contains one Mn(II) cation, one bpa ligand and one tbip anion. As shown in Fig. 7a, each Mn atom is octahedrally coordinated by two transN atoms of two bpa ligands and four oxygen atoms from three tbip ligands. The Mn–N bond lengths are 2.89(3) and 2.299(3) Å and the Mn–O bond lengths are in the range of 2.090(2)–2.377(2) Å, respectively. X-Ray structural analysis reveals that 3 consists of a 2D layer constructed from the linkage of 1D [Mn(bpa)]n chains via flexible bpa. The ribbon chain is similar to that of {[Mn(isop)(H2O)2]·(dpyo)·H2O}n (dpyo = 4,4′-dipyridyl N,N′-dioxide) (8).14 The infinite chain comprises 8- and 16- membered rings built up by Mn(II) ion and tbip anion (Fig. 7b). The 8-membered ring is formed by two symmetry related bridging carboxylate groups with a Mn⋯Mn distance of 4.32 Å. The coordination modes of the carboxylate groups of tbip in this case are similar to those in 1 and 2 (Scheme 1a). The adjacent ribbon chains are interlinked by the flexible bpa ligands to form a 2D layer with two kinds of rectangular grids (4.32 × 13.76 Å2 and 7.64 × 13.76 Å2), as shown in Fig. 7c.
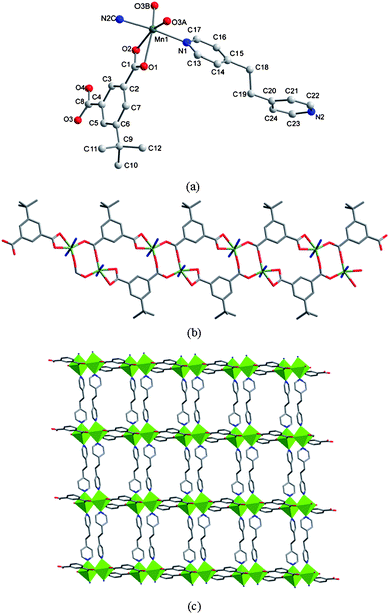 |
| Fig. 7 (a) Coordination environment of the Mn (II) ion in 3. The hydrogen atoms are omitted for clarity. Symmetry codes: A, −1 + x, y, z; B, 2 − x, −y, 2 − z; C, x, −1 + y, 1 + z. (b) View of a 1D ribbon-like chain of Mn atoms bridged by tbip ligand. (c) Ribbonlike chains pillared by flexible bpa ligands into a 2D layer. The tert-butyl groups are omitted for clarity. | |
[Mn(mip)(bpa)]n (4).
The structure of 4 is similar to that of compound 3 except that tbip is replaced by mip. Part of the structure of 4 is shown in Fig. 8. The Mn–O and Mn–N bond lengths and related angles for 3 and 4 are slightly different. The Mn(II) ions are bridged by mip ligands to form a 1D ribbon-like chain and then the bpa components connect the 1D chains to form a 2D layer with 4.54 × 13.86 Å2 and 7.13 × 13.86 Å2 windows (see Fig. S3, ESI).† The Mn⋯Mn separations embracing the 8-membered and 16-membered rings are 4.54 and 7.13 Å, being significantly different from those in 3 (4.32 and 7.64 Å). These separations seem to be modulated by different ligands (tbip and mip) occupying the axial positions of Mn(II) coordination spheres.
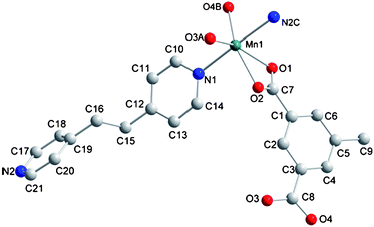 |
| Fig. 8 Coordination environment of the Mn (II) ion in 4. The hydrogen atoms are omitted for clarity. Symmetry codes: A, 1 − x, 1 − y, 1 − z; B, x, 1 + y, z; C, 1 + x, y, −1 + z. | |
{[Mn3(mip)2(Hmip)2(bpp)2(H2O)2]·2H2O}n (5).
The structure of 5 possesses a 1D ladder-like framework composed of the linear trimetallic cluster containing two five-coordinated and one six-coordinated manganese centers. The Mn2 atom lies on an inversion centre. The Mn1, Mn2 and Mn1A centers are bridged through six carboxylate groups, four bound as in Scheme 1c and two in Scheme 1d, respectively, to form a corner-sharing trinuclear unit, as shown in Fig. 9a, in which the Mn1⋯Mn2 distance is 3.48 Å.
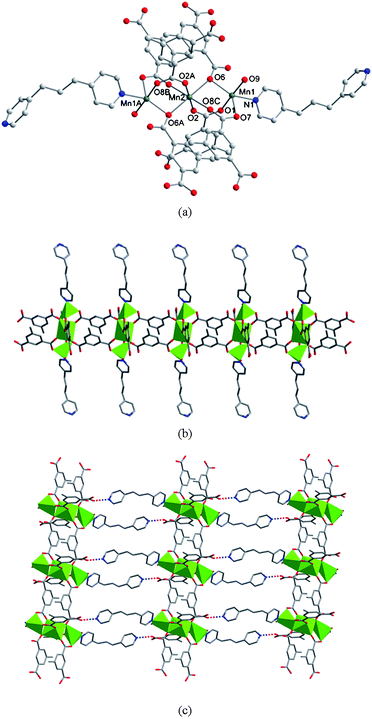 |
| Fig. 9 (a) Coordination environments of the trinuclear Mn centers in 5. Symmetry codes: A, 1 − x, −y, 1 − z; B, −x, −y, 1 − z; C, 1 + x, y, z. (b) A view of the ladder-like double chain structure extended along the c axis. (c) 2D supramolecular network in 5. Interchain hydrogen bonds are represented as dashed lines. | |
The Mn1 atom is five coordinated [MnO4N] and resides in a trigonal-bipyramidal coordination environment. Herein, the Mn(II) center could be described by a geometrical parameter τ in accord with the method of Addison et al. with τ = |β − α|/60, in which β and α are the bond angles that involve trans donor atoms in the basal plane.23 The τ value is around 0.52. Two oxygen atoms (O6 and O7) from two different tbip ligands and one nitrogen atom (N1) from a unidentate bpp form the equatorial plane and the apical positions are occupied by two oxygen atoms from one Htbip and one water with the O1–Mn1–O9 angle of 177.12°. The Mn2 adopts a distorted octahedral [MnO6] coordination geometry with four carboxylate oxygen atoms from four tbip ligands and two carboxylate oxygen atoms from two Htbip ligands. The Mn1–N distance is 2.232(2) Å and the Mn–O distances are in the range of 2.121(2)–2.529(2) Å, respectively.
It is worth noting that the adjacent trinuclear subunits are bridged by two mip ligands to form a ladder-like array running along the c-direction. Furthermore, these 1D motifs are decorated with bpp ligands at two sides in an outward fashion, as depicted in Fig. 9b. To our knowledge, this represents the second example for a 1D ladder-like framework constructed from linear trimetallic clusters and further dicarboxylate bridges (the first case is {[Mn3(ip)2(Hip)2(2,2-bipyridine)2]·2.5 H2O}n (9)15). These 1D arrays are further linked by O(3)-H(3)⋯N(2) hydrogen bonds to afford a 2D layer (Fig. 9c). In 5, the bpp ligand adopts a unidentate coordination mode and the unligated N2 atom acts as the hydrogen-bonding acceptor. Obviously, this unusual binding fashion of bpp will be sustained by the carboxylic groupvia the formation of such hydrogen bond. Otherwise, bpp would prefer the bridging mode as that of bipy, dpe and bpa in 1–4. Of further interest, when isop is used in the synthetic procedure, a different complex {[Mn(isop)(bpp)2]·0.5H2O}n (10) with a 1D ribbon chain was obtained.16 The structure of 10 consists of 1D polymeric chains built up by distorted octahedral Mn ions with dicarboxylate anions and trans-situated unicoordinated bpp. One of the carboxylate groups of isop acts as a chelating ligand while the other as a bis-monodentate towards two crystallographically related metal centers. The lattice water molecules connect the uncoordinated nitrogen atoms bpp in the adjacent chains through hydrogen bonds to form a 2D layer. Thus, although mip and isop possess similar carboxylate sites, they display an obviously different coordination chemistry in 5 and 10 (including the coordination environment of Mn, the coordination modes of carboxylate, the arrangement of 1D chain and the 2D supramolecular layer). This difference further confirms that the uncoordinated electron-donating coexistent groups in such ligands have an important effect on the final coordination complexes.
Thermal analysis and PXRD
The thermogravimetric analysis (TGA) pattern of 1–5 is shown in Fig. S4 (ESI).† The 3-D coordination polymer 1 remained stable until ∼380 °C, whereupon expulsion of its organic components occurred. The final mass remnant of 17.0% at ∼800 °C likely represented deposition of MnO (16.4% calcd). For complex 2, the first weight loss of 6.41% (calcd: 5.57%) was observed from 30 to 160 °C corresponding to the loss of one and a half free water molecules per formula unit. The second process might start from 160 to 455 °C, indicating the decomposition of the coordination framework. The final residue of 13.98% is close to the calculated 14.64% based on MnO. Complex 3 exhibited no mass loss until ∼175 °C, at which point a series of mass losses ensued. Its mass remnant of 15.5% corresponding to MnO (15.4% calcd). Complex 4 was stable up to ∼340 °C, and its mass remnant of 17.6% at ∼800 °C corresponding to MnO (17.0% calcd). The TG analysis of 5 shows the first weight loss of 5.17% (calcd. 5.34%) observed from 30 to 150 °C corresponding to the loss of two lattice water and two coordinated water molecules per formula unit. The second process might include three steps from 150 to 800 °C. This represents the decomposition of the material. In principle, the stability of an open structure cannot be solely determined from the TGA measurement since it may collapse without a notable change in weight. To further test the stability of the microporous framework of 2, we examined the samples by X-ray diffraction powder analysis. The fully evacuated sample of 2 was found to be unchanged at least up to 200 °C (Figure S5, ESI),† indicating that the microporous framework can keep stable after the loss of guest water molecules.
Magnetic properties
From a magnetic point of view, complexes 1, 2 and 3 can be considered as dinuclear ones, in which two Mn(II) ions are linked by two carboxylate bridges since couplings through tbip and bipy/dpe/bpa are almost negligible. The magnetic susceptibilities of 1–3 were measured in the 2–300 K temperature range, and shown as χMT and χMversusT plots in Fig. 10. The experimental χMT values of 1, 2 and 3 at room temperature are 8.80, 8.52 and 8.16 cm3K mol−1, respectively, which are close to that expected for a non-interacting pair of S = 5/2 ions (8.75 cm3K mol−1, S = 5/2) of the Mn(II). As temperature lowered to 2 K, the χMT values decreased first slowly and then rapidly. This behavior suggests that antiferromagnetic interactions are operative in 1, 2 and 3.
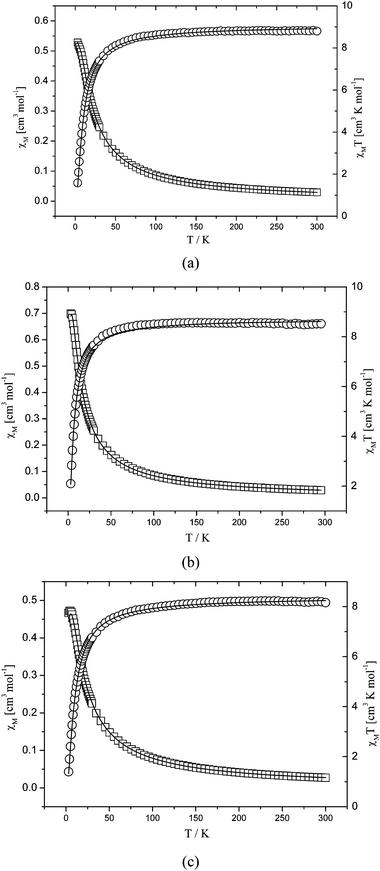 |
| Fig. 10 Temperature dependence of χMT and χM for 1 (a), 2 (b) and 3 (c). Open circles are the experimental data, and the solid line represents the best fit obtained from the Hamiltonian given in the text. | |
In order to quantitatively evaluate these magnetic interactions, for similar binuclear Mn(II) complexes, the following eqn (1) is induced from the Hamiltonian Ĥ = −JS1S224
|  | (1) |
A = exp[2J/KT] + 5exp[6J/KT] + 14exp[12J/KT] + 30exp[20J/KT] + 55exp[30J/KT] |
B = 1 + 3exp[2J/KT] + 5exp[6J/KT] + 7exp[12J/KT] + 9exp[20J/KT] + 11exp[30J/KT] |
The least-squares analysis of magnetic susceptibilities data led to J = −1.35 cm−1, g = 2.04 and R = 8.39 × 10−4 for 1, J = −0.93 cm−1, g = 1.99 and R = 7.88 × 10−5 for 2 and J = −1.33 cm−1, g = 1.96 and R = 2.88 × 10−4 for 3. The J values suggest that weak antiferromagnetic interactions between the neighboring Mn(II) ions are mediated through the double syn–syn mode μ-carboxylate bridges, which are comparable to those reported for other similar Mn(II) species.12–16
The magnetic susceptibilities, χM, of 5 were measured in the 2–300 K temperature range, and shown as χMT and χMversusT plots in Fig. 11. The experimental χMT value at 300 K is 12.87 cm3K mol−1, which is slightly lower than the spin-only value (13.13 cm3K mol−1) expected for three uncoupled high-spin Mn(II) ions. As the temperature lowers, the χMT value decreases gradually, which indicates the presence of an antiferromagnetic interactions in 5. Temperature dependence of the reciprocal susceptibilities (1/χM) obeys the Curie–Weiss law above 50 K with θ = −8.09 K, C = 12.87 and R = 1.03 × 10−3. The moderately negative θ value indicates the presence of antiferromagnetic interactions among the adjacent Mn(II) ions in 5.
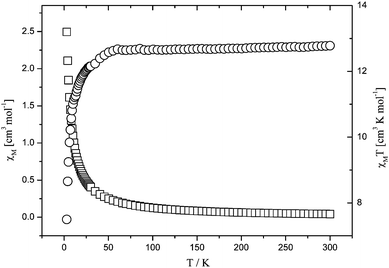 |
| Fig. 11 Temperature dependence of χMT and χM for 5. | |
Conclusions
By utilizing H2tbip or H2mip with different dipyridyl-type ligands, we have produced a variety of new coordination frameworks, including examples of 1D, 2D and 3D covalent connectivity. More relevant to our purpose, the different structures demonstrate the influence of a second ligand on the assembly of MOFs. For 1, rigid rod-like bipy was selected as an auxiliary ligand, and found to act as a pillar connector to link adjacent 2D layers into a 3D open structure. In 2, another rigid ligand (dpe) was taken into account, and a robust 3D structure encapsulating free water molecules with larger pores was formed. When the flexible bpa ligand was used, complex 3 with a 2D network structure, rather than the 3D frameworks in 1 and 2, was obtained. This may be attributed to the fact that the two methylene groups in dpa can rotate freely, which prohibits the connection of 2D layers into a 3D structure. For 4 and 5, flexible bpa and bpp ligands with different spacers also result in distinct 1D and 2D structures. Moreover, the bulky steric hindrance of H2tbip plays an important role in influencing the structural assembly. In addition, 2 has a stable metal–organic framework subject to the loss of guest water molecules. The magnetic susceptibilities of 1–3 and 5 reveal weak antiferromagnetic exchange interactions between the adjacent Mn(II) centers.
Acknowledgements
This work was supported by the Natural Science Foundation of China (Nos. 20771054 and 20771090) and Henan tackle key problem of science and technology (Nos. 072102270030 and 072102270034) and the Foundation of the Education Committee of Henan Province (Nos. 2006150017 and 2008A150018).
References
-
(a) M. Dinca, A. F. Yu and J. R. Long, J. Am. Chem. Soc., 2006, 128, 17153 CrossRef CAS;
(b) E. Tynan, P. Jensen, N. R. Kelly, P. E. Kruger, A. C. Lees, B. Moubaraki and K. S. Murray, Dalton Trans., 2004, 3440 RSC;
(c) L. F. Anne-Marie, A. L. David, J. L. Paul, M. Z. Alexandra and D. B. Walker, J. Am. Chem. Soc., 2005, 127, 12612 CrossRef CAS;
(d) X. Y. Duan, J. G. Lin, Y. Z. Li, C. J. Zhu and Q. J. Meng, CrystEngComm, 2008, 10, 207 RSC;
(e) A. K. Cheetham and C. N. R. Rao, Science, 2007, 318, 58 CrossRef CAS.
-
(a) J. Yang, Q. Yue, G. D. Li, J. J. Cao, G. H. Li and J. S. Chen, Inorg. Chem., 2006, 45, 2857 CrossRef CAS;
(b) Q. Yue, J. Yang, G. H. Li, G. D. Li, W. Xu, J. S. Chen and S. N. Wang, Inorg. Chem., 2005, 44, 5241 CrossRef CAS;
(c) B. Q. Ma, D. S. Zhang, S. Gao, T. Z. Jin and C. H. Yan, Angew. Chem., Int. Ed., 2000, 39, 3644 CrossRef CAS;
(d) Z. G. Li, G. H. Wang, H. Q. Jia, N. H. Hu and J. W. Xu, CrystEngComm, 2008, 10, 173 RSC.
-
(a) R. Matsuda, R. Kitaura, S. Kitagawa, Y. Kubota, R. V. Belosludov, T. C. Kobayashi, H. Sakamoto, T. Chiba, M. Takata, Y. Kawazoe and Y. Mita, Nature, 2005, 436, 238 CrossRef CAS;
(b) J. R. Li, Q. Yu, Y. Tao, X. H. Bu, J. Ribas and S. R. Batten, Chem. Commun., 2007, 2290 RSC;
(c) B. Moulton, J. Lu, R. Hajndl, S. Hariharan and M. J. Zaworotko, Angew. Chem., Int. Ed., 2002, 41, 2821 CrossRef CAS;
(d) H. Hayashi, A. P. Cote, H. Furukawa, M. O'Keeffe and O. M. Yaghi, Nat. Mater., 2007, 6, 501 CrossRef CAS.
-
(a) C. Aoki, T. Ishida and T. Nogami, Inorg. Chem., 2003, 42, 7616 CrossRef CAS;
(b) C. Beghidja, G. Rogez, J. Kortus, M. Wesolek and R. Welter, J. Am. Chem. Soc., 2006, 128, 3140 CrossRef CAS;
(c) S. Kitagawa, R. Kitaura and S. Noro, Angew. Chem., Int. Ed., 2004, 43, 2334 CrossRef CAS;
(d) Z. B. Han, X. N. Cheng and X. M. Chen, Cryst. Growth Des., 2005, 5, 695 CrossRef CAS;
(e) G. Férey, F. Millange, M. Morcrette, C. Serre, M. L. Doublet, J. M. Greneche and J. M. Tarascon, Angew. Chem., Int. Ed., 2007, 46, 3259 CrossRef CAS.
-
(a) M. A. M. Abu-Youssef, A. Escuer, M. A. S. Goher, F. A. Mautner, G. J. Reiss and R. Vicente, Angew. Chem., Int. Ed., 2000, 39, 1624 CrossRef CAS;
(b) J. L. Manson, A. M. Arif and J. S. Miller, Chem. Commun., 1999, 1479 RSC;
(c) H. Z. Kou, S. Gao, B. Q. Ma and D. Z. Liao, Chem. Commun., 1309 Search PubMed;
(d) M. Dinca, A. F. Yu and J. R. Long, J. Am. Chem. Soc., 2006, 128, 17153 CrossRef CAS.
-
(a) Y. Z. Zhang, W. Wernsdorfer, F. Pan, Z. M. Wang and S. Gao, Chem. Commun., 2006, 3302 RSC;
(b) K. Barthelet, J. Marrot, D. Riou and G. Fe'rey, Angew. Chem., Int. Ed., 2002, 41, 281 CrossRef CAS;
(c) P. M. Froster and A. K. Cheetham, Angew. Chem., Int. Ed., 2002, 41, 457 CrossRef CAS;
(d) O. M. Yaghi, M. O'Keeffe, N. W. Ockwig, H. K. Chael, M. Eddaoudi and J. Kim, Nature, 2003, 423, 705 CrossRef CAS;
(e) J. W. Ko, K. S. Min and P. Suh, Inorg. Chem., 2002, 41, 2151 CrossRef CAS;
(f) P. M. Forster, J. Eckert, B. D. Heiken, J. B. Parise, J. W. Yoon, S. H. Jhung, J. S. Chang and A. K. Cheetham, J. Am. Chem. Soc., 2006, 128, 16846 CrossRef CAS.
-
(a) W. Z. Shen, X. Y. Chen, P. Cheng, S. P. Yan, B. Zhai, D. Z. Liao and Z. H. Jiang, Eur. J. Inorg. Chem., 2005, 2297 CrossRef CAS;
(b) O. M. Yaghi, C. E. Davis, G. Li and H. Li, J. Am. Chem. Soc., 1997, 119, 2861 CrossRef CAS.
-
(a) R. H. Groeneman, L. R. MacGillivray and J. L. Atwood, Chem. Commun., 1998, 2735 RSC;
(b) X. M. Chen and G. F. Liu, Chem.–Eur. J., 2002, 8, 4811 CrossRef CAS;
(c) J. Cano, G. De Munno, J. Sanz, R. Ruiz, F. Lloret, J. Faus and M. Julve, J. Chem. Soc., Dalton Trans., 1994, 3465 RSC.
-
(a) C. E. Xanthopulos, M. P. Sigalas, G. A. Katsoulos, C. A. Tsipis, A. Terzis, M. Mentzafos and A. Hountas, Inorg. Chem., 1993, 32, 5433 CrossRef CAS;
(b) C. S. Hong, S. K. Son, Y. S. Lee, M. J. Jun and Y. Do, Inorg. Chem., 1999, 38, 5602 CrossRef CAS.
-
(a) R. H. Wang, D. Q. Yuan, F. L. Jiang, L. Han, S. Gao and M. C. Hong, Eur. J. Inorg. Chem., 2006, 1649 CrossRef CAS;
(b) T. K. Maji, S. Sain, G. Mostafa, T. H. Lu, J. Ribas, M. Monfort and N. R. Chaudhuri, Inorg. Chem., 2003, 42, 709 CrossRef CAS.
-
(a) S. Konar, P. S. Mukherjee, M. G. B. Drew, J. Ribas and N. R. Chaudhuri, Inorg. Chem., 2003, 42, 2545 CrossRef CAS;
(b) G. S. Waldo, S. Yu and J. E. Penner-Hahn, J. Am. Chem. Soc., 1992, 114, 5869 CrossRef CAS;
(c) X. S. Tan, D. F. Xiang, W. X. Tang and J. Sun, Polyhedron, 1997, 16, 689 CrossRef CAS;
(d) C. Policar, F. Lambert, M. Cesario and I. Morgenstern-Badarau, Eur. J. Inorg. Chem., 1999, 2201 CrossRef CAS;
(e) F. S. Delgado, N. Kerbellec, C. Ruiz-Pe'rez, J. Cano, F. Lloret and M. Julve, Inorg. Chem., 2006, 45, 1012 CrossRef CAS.
- C. B. Ma, C. N. Chen, Q. T. Liu, D. Z. Liao, L. C. Li and L. C. Sun, New J. Chem., 2003, 27, 890 RSC.
- C. B. Ma, C. N. Chen, Q. T. Liu, F. Chen, D. Z. Liao, L. C. Li and L. C. Sun, Eur. J. Inorg. Chem., 2004, 3316 CrossRef CAS.
- S. C. Manna, E. Zangrando, J. Ribas and N. R. Chaudhuri, Eur. J. Inorg. Chem., 2008, 1400 CrossRef CAS.
- C. B. Ma, C. N. Chen, Q. T. Liu, D. Z. Liao and L. C. Li, Eur. J. Inorg. Chem., 2008, 1865 CrossRef CAS.
- S. Konar, S. C. Manna, E. Zangrando, T. Mallah, J. Ribas and N. R. Chaudhuri, Eur. J. Inorg. Chem., 2004, 4202 CrossRef CAS.
-
(a) J. G. Lin, S. Q. Zang, Z. F. Tian, Y. Z. Li, Y. Y. Xu, H. Z. Zhu and Q. J. Meng, CrystEngComm, 2007, 9, 915 RSC;
(b) L. Y. Zhang, M. H. Zeng, X. Z. Sun, Z. Shi, S. H. Feng and X. M. Chen, J. Mol. Struct., 2004, 697, 181 CrossRef CAS;
(c) X. S. Tan, J. Sun, D. F. Xiang and W. X. Tang, Inorg. Chim. Acta, 1997, 255, 157 CrossRef CAS.
-
(a) Q. M. Wang, G. C. Guo and T. C. W. Mak, Chem. Commun., 1999, 1849 RSC;
(b) T. L. Hennigar, D. C. Macquarrie, P. Losier, R. D. Rogers and M. J. Zaworotko, Angew. Chem., Int. Ed. Engl., 1997, 36, 972 CrossRef CAS;
(c) S. N. Wang, J. F. Bai, Y. Z. Li, Y. Pan, M. Scheer and X. Z. You, CrystEngComm, 2007, 9, 1084 RSC;
(d) K. Biradha, M. Sarkar and L. Rajput, Chem. Commun., 2006, 4169 RSC;
(e) B. L. Chen, C. D. Liang, J. Yang, D. S. Contreras, Y. L. Clancy, E. B. Lobkovsky, O. M. Yaghi and S. Dai, Angew. Chem., Int. Ed., 2006, 45, 1390 CrossRef CAS;
(f) S. Q. Zang, Y. Su, Y. Z. Li, H. Z. Zhu and Q. J. Meng, Inorg. Chem., 2006, 45, 2972 CrossRef CAS;
(g) D. Bradshaw, T. J. Prior, E. J. Cussen, J. B. Claridge and M. J. Rosseinsky, J. Am. Chem. Soc., 2004, 126, 6106 CrossRef CAS.
-
(a) A. K. Cheetham, C. N. R. Rao and R. K. Feller, Chem. Commun., 2006, 4780 RSC;
(b) C. N. R. Rao, S. Natarajan and R. Vaidhyanathan, Angew. Chem., Int. Ed., 2004, 43, 1466 CrossRef CAS;
(c) M. G. Amiri, G. Mahmoudi, A. Morsali, A. D. Hunter and M. Zeller, CrystEngComm, 2007, 9, 686 RSC;
(d) Q. Y. Liu and L. Xu, CrystEngComm, 2005, 7, 87 RSC;
(e) D. R. Xiao, E. B. Wang, H. Y. An, Y. G. Li, Z. M. Su and C. Y. Sun, Chem.–Eur. J., 2006, 12, 6528 CrossRef CAS;
(f) M. Xue, G. S. Zhu, Y. J. Zhang, Q. R. Fang, I. J. Hewitt and S. L. Qiu, Cryst. Growth Des., 2008, 8, 427 CrossRef CAS;
(g) W. G. Lu, L. Jiang and T. B. Lu, Cryst. Growth Des., 2008, 8, 986 CrossRef CAS;
(h) X. M. Gao, D. S. Li, J. J. Wang, F. Fu, Y. P. Wu, H. M. Hu and J. W. Wang, CrystEngComm, 2008, 10, 479 RSC.
-
(a) D. R. Xiao, E. B. Wang, H. Y. An, Y. G. Li, Z. M. Su and C. Y. Sun, Chem.–Eur. J., 2006, 12, 6528 CrossRef CAS;
(b) B. R. Bhogala, S. Basavoju and A. Nangia, CrystEngComm, 2005, 7, 551 RSC;
(c) W. J. Zhuang, X. J. Zheng, L. C. Li, D. Z. Liao, H. Ma and L. P. Jin, CrystEngComm, 2007, 9, 653 RSC;
(d) C. B. Aakeroy, J. Desper and J. F. Urbina, CrystEngComm, 2005, 7, 193 RSC;
(e) Z. Z. Lin, F. L. Jiang, D. Q. Yuan, L. Chen, Y. F. Zhou and M. C. Hong, Eur. J. Inorg. Chem., 2005, 1927 CrossRef CAS;
(f) J. L. Rowsell and O. M. Yaghi, J. Am. Chem. Soc., 2006, 128, 1304 CrossRef CAS;
(g) H. Y. Wang, S. Gao, L. H. Huo, S. W. Ng and J. G. Zhao, Cryst. Growth Des., 2008, 8, 665 CrossRef CAS;
(h) H. P. Jia, W. Li, Z. F. Ju and J. Zhang, Dalton Trans., 2007, 3699 RSC.
-
(a) L. F. Ma, L. Y. Wang, X. K. Huo, Y. Y. Wang, Y. T. Fan, J. G. Wang and S. H. Chen, Cryst. Growth Des., 2008, 8, 620 CrossRef CAS;
(b) L. F. Ma, Y. Y. Wang, L. Y. Wang, J. Q. Liu, Y. P. Wu, J. G. Wang, Q. Z. Shi and S. M. Peng, Eur. J. Inorg. Chem., 2008, 693 CrossRef CAS.
-
(a) L. Pan, B. Parker, X. Y. Huang, D. H. Oison, J. Y. Lee and J. Li, J. Am. Chem. Soc., 2006, 128, 4180 CrossRef CAS;
(b) H. Chun, J. Am. Chem. Soc., 2008, 130, 800 CrossRef CAS.
- A. W. Addison and T. N. Rao, J. Chem. Soc., Dalton Trans., 1984, 1349 RSC.
-
R. L. Carlin, Magnetochemistry, Springer, Berlin, 1986 Search PubMed.
Footnote |
† Electronic supplementary information (ESI) available: Additional experimental details. CCDC reference numbers 694054–694058. For ESI and crystallographic data in CIF or other electronic format see DOI: 10.1039/b811725j |
|
This journal is © The Royal Society of Chemistry 2009 |
Click here to see how this site uses Cookies. View our privacy policy here.