DOI:
10.1039/B812458B
(Paper)
Analyst, 2009,
134, 107-113
Turn-on fluorescent cyanide sensor based on copper ion-modified CdTe quantum dots†
Received
22nd July 2008
, Accepted 9th September 2008
First published on 20th October 2008
Abstract
A new fluorescent sensor for the sensitive and selective detection of cyanide (CN−) in aqueous media was developed herein. The sensing approach is based on CN−-modulated quenching behavior of Cu2+ toward the photoluminescence (PL) of CdTe quantum dots (QDs). In the presence of CN−, the PL of QDs that have been quenched by Cu2+ was found to be efficiently recovered, which then allows the detection of CN− in a very simple approach. Experimental results showed that the pH of the buffer solution, concentration of copper ions, and size of CdTe QDs all influenced the response of the sensor to CN−. Under the optimal conditions, a good linear relationship between the PL intensity and the concentration of CN− can be obtained in the range of 3.0 × 10−7 to 1.2 × 10−5 M, with a detection limit as low as 1.5 × 10−7 M. In addition, the present fluorescent sensor possesses remarkable selectivity for cyanide over other anions, and negligible influences were observed on the cyanide detection by the coexistence of other anions or biological species (such as albumin and typical blood constituents). Therefore, we expect the proposed copper ion-modified QDs to be an efficient and reliable sensing system to monitor cyanide concentration in environmental or clinical applications.
Introduction
The recognition and sensing of anions have received considerable attention due to their important roles in biological, environmental, and industrial processes.1–6 While the detection of anions that can be potentially harmful to the environment or humans is of particular interest, cyanide is one such anion that has drawn the attention of researchers in recent years.7Cyanide is known as one of the most lethal poisons, and the mechanism of human toxicity for cyanide lies in its ability to suppress the transport of oxygen.8,9 In addition to being found in many foods and plants, cyanide is also commonly used worldwide in industrial applications such as metal plating, metal mining and plastics manufacture.10 As a consequence, accidental cyanide release in wastewater or rivers may lead rapidly to serious contamination of groundwater and even drinking water.11 Thus, there exists an emergent need for an efficient and reliable sensing system for cyanide to monitor cyanide concentration from contaminant sources. On the other hand, researchers have reported that cyanide played an important role in the cause of death of fire cases, and thus the determination of blood cyanide levels in fire victims has also been an area of active research.12–14
Up to now, a variety of analytical methods have been developed to detect cyanide such as colorimetric,15–17 chromatographic,18,19 fluorimetric,7,20–25 flow injection,26,27 and electrochemical28,29 analyses. Among the various reported methods, fluorescent sensors present many appealing advantages, including high sensitivity, remote control, low cost, easy detection, and especially suitability as a diagnostic tool for biological concern.30 While a number of synthetic organic fluorophore receptors for cyanide anion have been designed, relatively few could work in aqueous media and few of them are capable of displaying high selectivity over other anions. In addition, these organic fluorophores suffer from low quantum yield and poor photostability, which thus limits their practical applications.31,32 In this regard, the recently developed semiconductor quantum dots (QDs) show great promise as the fluorescence reporter for sensing events.32–34
QDs have unique photophysical properties in comparison with traditional organic dyes due to their quantum-size confinement effects, including high fluorescence quantum yield, size-tunable narrow emissions and minimal photobleaching.32–34 Therefore, by virtue of the excellent photophysical properties of QDs, researchers have made efforts toward the development of QD-based fluorescent sensors for detecting anion species such as cyanide. For example, Sanz-Medel and co-workers reported the use of both photoactivated35 and surface-modified36CdSe QDs for cyanide detection, but they suffered from either low sensitivity or the necessity to work in organic solvents. While both sensors by Sanz-Medel worked in a turn-off mode, a recent work by Mareque-Rivas and co-workers37 reported a turn-on cyanide sensor based on hydrophobic CdSe QDs, which appeared more favorable in the fluorescent sensors design. Unfortunately, the use of toxic, volatile organic solvent (chloroform) and low sensitivity (the detection limit is 20 μM at pH 10) also restricts their further application in developing a practical cyanide sensor.
Herein, we report a new QD-based fluorescent sensor for cyanide (CN−). The sensing mechanism is based on CN−-modulated quenching behavior of Cu2+ toward the photoluminescence (PL) of CdTe QDs (shown in Scheme 1). In the absence of CN−, the PL of copper ion-modified QDs was very weak due to the strong quenching ability of paramagnetic Cu2+. However, in the presence of CN−, Cu2+ could be desorbed from the surface of the QDs because of the much higher stability constant of the complex of CN− and copper ions.37,38 As a result, the quenched PL would be effectively recovered, based on which the detection of CN− can be achieved. Notably, the present sensor functions in a turn-on mode, works in pure aqueous media, possesses an extremely low detection limit, and displays high selectivity over other anions.
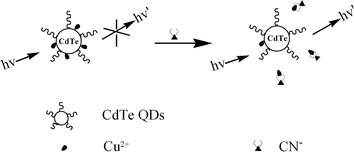 |
| Scheme 1 Schematic illustration of QD-based fluorescent cyanide sensor. | |
Experimental
Reagents
Mercaptosuccinic acid and bovine serum albumin (BSA; fraction V) were bought from Sigma-Aldrich. Sodium borohydride was purchased from Acros. Na2TeO3 was brought from Germany. Cadmium chloride, trisodium citrate dihydrate, lactic acid, uric acid, sodium pyruvate, potassium cyanide, and other salts were all bought from Beijing Chemical Co. (China). All chemicals were of analytical grade, and used without further purification. The water used was purified through a Millipore system.
Preparation of CdTe QDs
CdTe QDs were prepared according to the reported method.39 In a typical synthesis, cadmium chloride (CdCl2, 0.04 M, 4 mL) was diluted to 50 mL in a one-necked flask, and trisodium citrate dihydrate (100 mg), Na2TeO3 (0.01 M, 1 mL), mercaptosuccinic acid (50 mg), and sodium borohydride (NaBH4, 100 mg) were added successively with stirring. When the color of the solution changed to green, the flask was attached to a condenser and refluxed at 110 ± 1 °C under open-air conditions for 10 h. The resulting CdTe QDs were washed with ethanol and separated by centrifugation twice. Finally, the purified CdTe QDs were dispersed in the Tris-HCl buffer (0.01 M, pH 7.0) solution.
Calculation of the size and concentration of CdTe QDs
The concentration and size of as-synthesized CdTe QDs was calculated according to the method proposed by Peng and co-workers.40 Firstly, the particle size can be determined from the first absorption maximum in virtue of the following empirical formula: | D = (9.8127 × 10−7)λ3 − (1.7147 × 10−3)λ2 + (1.0064)λ − 194.84 | (1) |
where D (nm) is the size of CdTe QDs, and λ (nm) is the wavelength of the first excitonic absorption peak of the corresponding sample. Then, the extinction coefficient of CdTe QDs (ε) can be obtained from the following equation: | ε = 10 043D2.12. | (2) |
Finally, the concentration of the QDs (C) can be calculated upon the measurement of the absorbance of the QD solution (A) according to Beer's Law:
PL quenching of CdTe QDs by Cu2+
A 2.4 mM stock solution of copper nitrate was prepared, from which various Cu2+ concentrations were prepared by serial dilution. For the quenching studies, solution of QDs and Cu2+ with different concentrations were mixed in Tris-HCl buffer, and equilibrated for 5 min before the spectral measurements. The preliminary investigation showed that the interaction could reach equilibrium within 5 min. The PL quenching data were analyzed by using Stern–Volmer equation:41where F and F0 are the PL intensity at 610 nm in the presence and absence of Cu2+, respectively, KSV is the Stern–Volmer quenching constant, and [Q] is the quencher concentration ([Cu2+]). To investigate the selectivity of CdTe QDs to Cu2+, a 2.4 mM stock solution of the following salts was used: KNO3, NaNO3, Ca(NO3)2, Ba(NO3)2, Mg(NO3)2, MnCl2, Cd(NO3)2, Al(NO3)3, Zn(NO3)2, Fe(NO3)3, Co(NO3)2, Ni(NO3)2, and Pb(NO3)2.
Fluorescent detection of CN−
A 0.12 M stock solution of KCN was prepared in Tris-HCl buffer of pH 7.0, from which various CN− concentrations were prepared by serial dilution. The stock solutions were stored at 4 °C and used within three days. A freshly prepared mixture solution containing 22 nM CdTe QDs and 3.0 × 10−7 M Cu2+ in 0.01 M Tris-HCl buffer of pH 7.0 (we denote this particular mixture solution as ‘Cu2+-QDs’ in the following) was used for CN− detection, into which KCN with different concentrations was added and equilibrated for 10 min before the spectral measurements. All the experiments were performed at room temperature (290 ± 1 K).
Selectivity and interference measurements
To probe the anion selectivity of copper ion-modified QDs toward CN−, the following salts were used: CH3COONa (NaAc), NaBr, KBrO3, NaCl, NaClO4, Na2CO3, K2C2O4, KF, KIO3, NaI, NaNO2, Na3PO4, Na2SO3, Na2SO4, Na2S, and KSCN. A 0.12 M stock solution was prepared. Subsequently, salt solution with an appropriate volume was mixed with Cu2+-QDs. Then the solution was equilibrated for 10 min before the spectral measurements. For the interference investigations, the above salt solution with a final concentration of 2.4 × 10−4 M was mixed with Cu2+-QDs in the presence of 1.2 × 10−5 M CN−. The effect of BSA, uric acid, pyruvate, and lactic acid to the CN− detection was investigated by mixing BSA (0.5 g L−1), uric acid (0.3 mM), pyruvate (0.05 mM), or lactic acid (1.4 mM) with Cu2+-QDs in the presence of 1.2 × 10−5 M CN−.
Preparation of samples for XPS
In order to facilitate the X-ray photoelectron spectroscopy (XPS) characterization, the concentration of all reactants increased by ten-fold. Briefly, 3.0 × 10−6 M Cu2+ and 2.2 × 10−7 M CdTe QDs were mixed and equilibrated for 10 min. The mixture solution was then dialyzed in a 3500 MWCO (molecular weight cut off) dialysis membrane against 0.01 M Tris-HCl buffer (pH 7.0) for 48 h with the buffer solution refreshed every 12 h to remove any free Cu2+. Then 6.0 × 10−3 M KCN was added to the above purified solution and equilibrated for 30 min, which was followed by dialysis in a 3500 MWCO membrane against 0.01 M Tris-HCl buffer (pH 7.0) for 24 h with the buffer solution refreshed every 8 h. The Tris-HCl buffer solution was collected and concentrated. Finally, 20 μL of the obtained solution was dropped onto a clean silicon wafer for XPS characterization.
Instruments
Absorption measurements were performed with a Cary 500 UV-vis-NIR spectrometer (Varian). Fluorescence measurements were carried out on an LS-55 Luminescence Spectrometer (Perkin-Elmer). The emission spectra were recorded in the wavelength range of 520–700 nm upon excitation at 500 nm. Each spectrum was the average of three scans. A 1.00 cm path length rectangular quartz cell was used for these measurements. X-Ray photoelectron spectroscopy (XPS) measurements were carried out on an ESCALAB-MKII spectrometer (VG Co., UK) with Al Kα X-ray radiation as the X-ray source for excitation.
Results and discussion
PL quenching of CdTe QDs by Cu2+
The as-prepared mercaptosuccinic acid-capped CdTe QDs possess a well-resolved absorption maximum of the first electronic transition at 566 nm. According to the literature,40 the size of the CdTe QDs is calculated to be ca. 3.4 nm. The PL spectrum of QDs displays an emission maximum at 610 nm upon excitation at 500 nm. Since the PL of QDs is known to be pH-dependent, Tris-HCl buffer (0.01 M, pH 7.0) was used to disperse the QDs. In the following experiments, a 22 nM solution of CdTe QDs was used. At this concentration, the absorbance of the QD solution at the excitation wavelength is less than 0.01, thus the possible self-absorption and inner filter effect in the fluorescence measurements can be minimized.41 In addition, a strong fluorescence signal can be obtained to ensure a good signal-to-noise ratio.
Fig. 1 displays the PL spectra of the CdTe QDs in the presence of Cu2+ with varying concentrations. As shown, with increasing the concentration of Cu2+, the PL intensity decreased gradually. Meanwhile, there was no discernible change in the shape of the fluorescence spectrum accompanied quenching, except a slight red-shift at higher Cu2+ concentration. The fluorescence quenching data are analyzed by Stern–Volmer equation (see Experimental section). The resulting plots are given in the inset of Fig. 1, which showed a good linear relationship. KSV, which is a measurement of the quenching efficiency of the quencher, was calculated to be 2.9 × 107 M−1. Such a large KSV value suggested that Cu2+ exhibited a strong quenching ability toward the PL of the CdTe QDs. In addition, the quenching of QDs by Cu2+ was highly specific. Among the various metal ions investigated, only Cu2+ quenched the PL of CdTe QDs effectively (see Fig. S1 in the ESI†). While several recent studies have thoroughly addressed the quenching feature between QDs and Cu2+,42–45 detailed investigations on the interaction between Cu2+ and CdTe QDs were not performed in the present study.
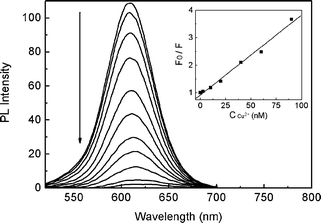 |
| Fig. 1
Fluorescence emission spectra of CdTe QDs in the presence of increasing amounts of Cu2+. The inset displays the linear plots by Stern–Volmer analysis. | |
Recovery on the PL of copper ion-modified QDs by CN−
Cyanide can react with copper ions forming particularly stable [Cu(CN)n](n
−
1)− species,46 and thus copper ions may be removed from other weakly-bound surfaces by CN−.37,38,47 Therefore we further explored whether the quenched PL of copper ion-modified QDs could be illuminated in the presence of CN− upon desorption of copper ions from the QD surfaces. The experimental results then verified our above thought. As shown in Fig. 2, the weak PL (curve a) was enhanced gradually after adding increasing concentrations of CN− (curves b–d). Meanwhile, a blue-shift on the emission maximum was observed. It is necessary to note that addition of CN− to pure CdTe QD solutions produced no obvious PL changes. For example, in the presence of 6.0 × 10−4 M KCN, the PL of QDs only decreased by 3.4%. This was contrary to the previous studies which observed the strong interaction of CN− itself with QDs.35,36 We infer it was the different capping ligand that caused the different reaction behavior of QDs with CN−.
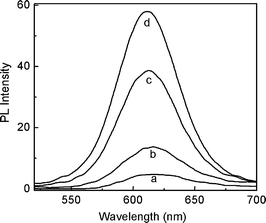 |
| Fig. 2
Fluorescence emission spectra of 22 nM QDs containing 3.0 × 10−7 M Cu2+ in the absence (curve a) and presence of 3.0 × 10−6 M (curve b), 3.0 × 10−5 M (curve c), and 6.0 × 10−4 M (curve d) KCN. | |
Fig. 3 presents the PL data as a function of F/F0 (F and F0 are the PL intensity at 615 nm in the presence and absence of CN−, respectively) versus the concentration of CN−. As can be seen, the presence of trace amounts of CN− gave rise to an obvious increase in the PL of copper ion-modified QDs. For instance, an over four-fold PL enhancement could be obtained in the presence of only 1.2 × 10−5 M CN−. This suggested a sensitive response of the present system toward CN−. The PL intensity of copper ion-modified QDs upon adding CN−versus the incubation time is also shown in the inset of Fig. 3, which suggested the reaction reached equilibrium within 10 min. The relatively long incubation time reflected the slow reaction kinetic character between Cu2+-modified QDs and CN−.
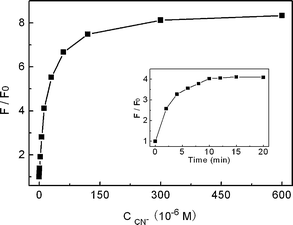 |
| Fig. 3 Relative PL intensity (F/F0) of QD solution containing 3.0 × 10−7 M Cu2+ at 615 nm versus the concentration of added KCN at pH 7.0. The inset displays the PL intensity upon adding 1.2 × 10−5 M KCN as a function of the incubation time. | |
Effect of pH, Cu2+ concentration, and QD size on the response toward CN−
The sensitive PL response of Cu2+-modified QDs toward CN− afforded the possibility to develop a fluorescent cyanide sensor. In order to choose the optimal conditions for cyanide determination, we then investigated various possible influences on the detection. Fig. 4 shows the effect of pH on the PL response toward 3.0 × 10−5 M of CN−. The results showed a larger PL increase at higher pH in the range between 5.3 and 7.0, which can be rationalized by the fact that a higher pH favors the binding of CN− to copper due to the deprotonation of CN−. The PL enhancement at pH 8.0 was, however, weaker than that at pH 7.0, which was possibly due to the interference from the forming Cu(OH)2 precipitates.17 In basic solution, the background PL intensity would be higher due to the precipitation of Cu2+, which then decreased the enhancement effect. Thus a Tris-HCl buffer solution of pH 7.0 was used in the cyanide detection.
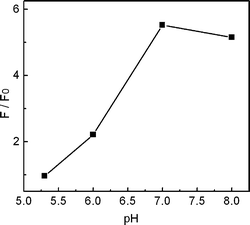 |
| Fig. 4
PL response of QD solutions containing 3.0 × 10−7 M Cu2+ toward 3.0 × 10−5 M KCN at different pH values. | |
The concentration of Cu2+ in the system was also found to be essentially important. As shown in Fig. 5, a Cu2+ concentration of 3.0 × 10−7 M corresponding to 22 nM CdTe QDs gave the best enhancement results. Either more or less Cu2+ than this amount would result in a decrease on the enhancement effect. For example, when more copper ions were present, the added CN− would react firstly with free Cu2+, which then decreases the enhancement effect. On the other hand, if less copper ions were present, the background PL intensity of the QDs would be higher which could also affect the sensitivity for CN− detection greatly.
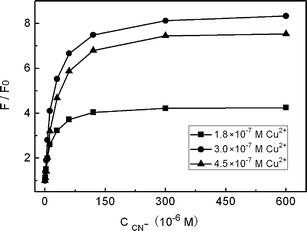 |
| Fig. 5
PL response of QD solutions containing different Cu2+ concentrations versus the concentration of KCN at pH 7.0. | |
Next, we studied the effect of QD size on the response toward CN−. Fig. 6 presents the PL results using two different-sized CdTe QDs with other conditions being identical. As shown, the PL enhancement effect of QDs of 3.4 nm size was much larger than that with 2.5 nm size. The possible reason of this QD size-dependence should be related with size-dependent quenching behavior of Cu2+ to the CdTe QDs. In one recent work,45 Zhu and co-workers found that Cu2+ quenched larger CdTe QDs more efficiently. This meant that larger QDs would report the presence of Cu2+ more sensitively, which then accordingly enhanced the PL change of Cu2+-modified QDs toward CN−.
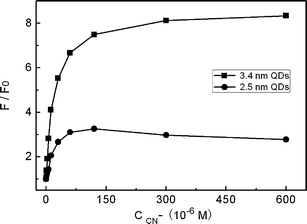 |
| Fig. 6
PL response of QD solutions containing 3.0 × 10−7 M Cu2+versus the concentration of KCN at pH 7.0 with different QD sizes. | |
Detection of CN− based on copper ion-modified QDs
Analytical parameters of the present sensor system for cyanide detection were then evaluated. Under the above optimal conditions, a good linear relationship between F/F0 and the concentration of CN− was obtained in the range of 3.0 × 10−7 to 1.2 × 10−5 M (R2 = 0.9936), as shown in Fig. 7. The detection limit was calculated, following the IUPAC criterion, as the concentration of CN− which produced an analytical signal three times the standard deviation of the blank signal. The result then suggested a detection limit of 1.5 × 10−7 M, which compared favorably with previously reported fluorescence methods for CN− detection.7,22–25,35–38 According to the World Health Organization (WHO), cyanide concentrations lower than 1.9 μM are acceptable in drinking water,48 and recent studies have shown that the lethal cyanide concentration in the blood of fire victims is ca. 20 μM.12–14 This meant that our QD-based sensor was sensitive enough to monitor cyanide concentration in drinking water or in fire victims. The low detection limit obtained in the present sensor system can be ascribed to the following facts. Firstly, the present sensor works in a ‘turn-on’ mode which is usually more sensitive than a turn-off assay, since the enhanced fluorescence nature of the signal transduction affords a much better signal-to-noise ratio for the sensing scheme.23 Secondly, CdTe QDs can report the presence of Cu2+ in a particularly sensitive approach due to the strong quenching ability of paramagnetic Cu2+ as evidenced by the large KSV value. In addition, the strong complexing ability of CN− to copper ions and the high PL quantum yield of QDs should also contribute to the high sensitivity. The relative standard deviation for five repeated measurements of 1.2 × 10−5 M of CN− was 2.2%, which illustrated that the response of copper ion-modified QDs toward CN− was highly reproducible.
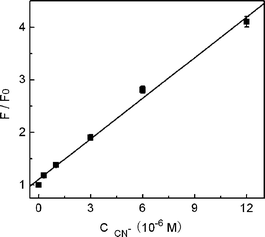 |
| Fig. 7 Linear plots of relative PL intensity (F/F0) at 615 nm as a function of KCN concentration. The error bars represent the standard deviation of three measurements. | |
To evaluate the CN−-selectivity of the present sensor system, PL changes in the presence of other anions, including Ac−, Br−, BrO3−, Cl−, ClO4−, CO32−, C2O42−, F−, IO3−, I−, NO2−, PO43−, SO32−, SO42−, S2−, and SCN−, were then investigated. As shown in Fig. 8A, with the identical concentration (1.2 × 10−4 M), anions other than CN− did not cause any significant changes in the PL intensity of copper ion-modified QDs. Thus, the selectivity profile for CN− over other anions was remarkably high. In order to further verify the performance of our proposed sensor system in real samples, the fluorescence detection was then investigated in the presence of competing anions. As shown in Fig. 8B, the PL intensity in the presence of 1.2 × 10−5 M of CN− was hardly changed by the coexistence of 20-fold completing anions (2.4 × 10−4 M). It is evident these anions did not have a significant impact on the ability of the present sensor system to detect CN−. As in the case for monitoring cyanide in biological samples, it is important to consider the possible interference from other physiological species.29 We subsequently investigate the fluorescence assay of CN− in the presence of albumin (BSA) or other blood constituents (such as uric acid, pyruvate, and lactic acid), which indicated a less than 5% perturbation on the sensor response. In addition, the influence of foreign metal ions to the sensor performance was tested, where an error of ±5% in the relative fluorescence intensity was considered tolerable. Among the tested metal ions, K+, Na+, Mg2+, Ba2+, Ca2+, Zn2+ and Al3+ could be allowed to be higher than 2.4 × 10−5 M, and Cd2+, Mn2+, Pb2+ and Fe3+ could be allowed to be higher than 3.0 × 10−6 M. The tolerance of Co2+ and Ni2+ was at a relatively low level, 1.0 × 10−6 M, which was over three-fold of the existed copper ions. Therefore, for the sample with higher metal ions contaminations, pretreatment would be necessary at present. Nevertheless, our sensing scheme still affords many appealing advantages compared with previous studies.
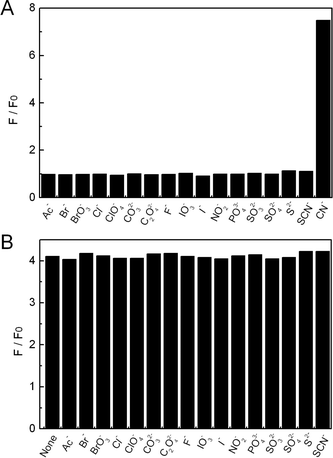 |
| Fig. 8 (A) Relative PL intensity (F/F0) of QD solutions containing 3.0 × 10−7 M Cu2+ in the presence of 1.5 × 10−4 M various anions at pH 7.0. (B) Relative PL intensity (F/F0) of QD solutions containing 3.0 × 10−7 M Cu2+ in the presence of 1.2 × 10−5 M KCN with the coexistence of 2.4 × 10−4 M other anions at pH 7.0. | |
As compared with the recent work of Mareque-Rivas and co-workers37 which also utilized the ability of CN− to remove copper ions for developing a turn-on fluorescent cyanide sensor, the present method has many distinct advantages. Firstly, the use of water-soluble QDs in the present sensor rather than hydrophobic QDs can avoid the use of toxic, volatile organic solvents which would restrict the analytical potential of the cyanide sensor. Secondly, the present fluorescent sensor has a detection limit for cyanide as low as 1.5 × 10−7 M, which is much lower than the method of Mareque-Rivas. In addition, while a copper-binding ligand like 2,2′-bipyridine had to be used in their sensor to bridge QDs and Cu2+, the present sensor works just by the direct interaction between QDs and Cu2+, which makes our method much simpler. Therefore, the present copper ion-modified QDs provide a more clean, simple, and sensitive fluorescent sensor for the detection of cyanide in pure aqueous media at physiological pH.
Mechanism of the present turn-on fluorescence CN− sensor
Cu2+ is a well-known and highly efficient fluorescence quencher. Several recent studies have shown that the PL of thiol-capped semiconductor QDs such as CdS, CdSe and CdTe could be efficiently quenched by Cu2+ and the quenching mechanism has been investigated thoroughly.42–45,49 According to previous studies, the addition of Cu2+ to QDs led to binding of Cu2+ on the surface of the QDs, accompanied by rapid reduction of Cu2+ to Cu+. On the other hand, since the addition of CN− to QDs without the modification of Cu2+ produced no remarkable PL change, the enhanced PL in Fig. 2 could not be attributed to the direct interaction of CN− with the QD surfaces. Thus the observed CN−-induced PL increase should be the result of interaction between copper ions and CN−. Attempts to investigate the sensing mechanism by absorption spectroscopy, however, failed due to the extremely low absorbance of QDs in the present experimental conditions. In order to verify the desorption of copper ions from QDs upon the interaction with CN−, we performed the following experiment. The Cu2+-modified QD solution was firstly dialyzed to remove any free copper ions. Then CN− was added to the above solution and subjected to dialysis against Tris-HCl buffer solution. Finally the Tris-HCl buffer solution was collected and concentrated for X-ray photoelectron spectroscopy (XPS) characterization to confirm whether copper ions were present. The appearance of Cu2p peaks in XPS spectra (see Fig. S2 in the ESI†) suggested the existence of copper in the solution, which confirmed the occurrence of a copper ion-desorption event on the surface of the QDs in the presence of CN−.
While the above experiment presented the direct evidence of our proposed mechanism, indirect support came from the shift on the position of the emission maximum of the QDs before and after the treatment with CN−. As shown in Fig. 1, a red-shift on the emission maximum of QDs from 610 to 615 nm was observed upon the interaction with Cu2+. After the addition of CN−, the emission maximum was found to move back (or blue-shift) gradually. For instance, at a concentration of 6.0 × 10−4 M CN−, the emission maximum was found to shift from 615 to 611 nm (curve d in Fig. 2). This phenomenon accorded well with our proposed sensing mechanism that the bound copper ions were desorbed from the QD surface upon the interaction with CN−, thus the Cu2+-shifted emission maximum was restored correspondingly.
Furthermore, a quantitative support to our proposed mechanism can be obtained from the copper quenching study in the absence and presence of CN− (see Fig. S3 in the ESI†). While the PL of the QDs was quenched by 96% upon the addition of 3.0 × 10−7 M Cu2+, adding the same amount of Cu2+ into the QD solution containing 1.2 × 10−5 M CN− resulted in only an 8.1% decrease. This suggested that the binding of copper to CN− was much stronger than to the QDs. Therefore, in the mixture solution of CN− and CdTe QDs, copper would prefer to bind with CN− rather than the CdTe QDs which led to less quenching. Accordingly, upon the addition of CN− to the copper ion-modified QDs, the quencher Cu2+ would move away from the QD surfaces to form more stable complexes with CN−, which then recovered the PL of the QDs.
Conclusions
In this work, we have reported the development of a new cyanide sensor based on copper ion-modified CdTe QDs in aqueous media. The sensor functions by the ability of CN− to bind and remove copper ions from the surface of QDs which recovers the PL that has been quenched by Cu2+. The enhanced fluorescence nature, strong fluorescence quenching ability of Cu2+, much higher stability constant of the complex of CN− and copper ions, and high quantum yields of QDs ensure a sensitive and selective response of the present sensor system to CN−. Besides the advantage of a low detection limit (lower than 1.9 μM), the long-wavelength emission nature (above 600 nm) and negligible influence of possible interferences (such as other anions, albumin, or blood constituents) make the present sensor system potentially applicable to monitor cyanide concentration in wastewater or in fire victims. On the other hand, given the wide interests of semiconductor QDs in many research fields recently, we expect that the present study may also serve as a foundation to the further understanding and application of semiconductor QDs.
Acknowledgements
This work was supported by the National Natural Science Foundation of China (Nos. 20575064 and 20675076).
References
- M. M. G. Antonisse and D. N. Reinhoudt, Chem. Commun., 1998, 443 RSC.
- T. Gunnlaugsson, M. Glynn, G. M. Tocci, P. E. Kruger and F. M. Pfeffer, Coord. Chem. Rev., 2006, 250, 3094 CrossRef CAS.
- J. W. Steed, Chem. Commun., 2006, 2637 RSC.
- C. Suksai and T. Tuntulani, Top. Curr. Chem., 2005, 255, 163 CAS.
- R. Martinez-Manez and F. Sancenon, Chem. Rev., 2003, 103, 4419 CrossRef CAS.
- P. D. Beer and P. A. Gale, Angew. Chem., Int. Ed., 2001, 40, 486 CrossRef.
- N. Gimeno, X. Li, J. R. Durrant and R. Vilar, Chem.–Eur. J., 2008, 14, 3006 CrossRef CAS.
-
K. W. Kulig, Cyanide Toxicity, U.S. Department of Health and Human Services, Atlanta, GA, 1991 Search PubMed.
-
B. Vennesland, E. E. Comm, C. J. Knownles, J. Westly and F. Wissing, Cyanide in Biology, Academic Press, London, 1981 Search PubMed.
-
Ullmann's Encyclopedia of Industrial Chemistry, Wiley-VCH, New York, 1999 Search PubMed.
-
C. Young, L. Tidwell and C. Anderson, Cyanide: Social, Industrial, and Economic Aspects, Minerals, Metals, and Materials Society, Warrendale, 2001 Search PubMed.
- A. Ishii, H. Seno, K. Watanabe-Suzuki, O. Suzuki and T. Kumazawa, Anal. Chem., 1998, 70, 4873 CrossRef CAS.
- F. Moriva and Y. Hashimoto, J. Forensic Sci., 2001, 46, 1421.
- A. E. Lindsay, A. R. Greenbaum and D. O'Hare, Anal. Chim. Acta, 2004, 511, 185 CrossRef.
- F. H. Zelder, Inorg. Chem., 2008, 47, 1264 CrossRef CAS.
- J. Ren, W. Zhu and H. Tian, Talanta, 2008, 75, 760 CrossRef CAS.
- D. Cacace, H. Ashbaugh, N. Kouri, S. Bledsoe, S. Lancaster and S. Chalk, Anal. Chim. Acta, 2007, 589, 137 CrossRef CAS.
- M. Aguilar, A. Farran and V. Marti, J. Chromatogr., A, 1997, 778, 397 CrossRef CAS.
- T. T. Christison and J. S. Rohrer, J. Chromatogr., A, 2007, 1155, 31 CrossRef CAS.
- K. S. Lee, H. J. Kim, G. H. Kim, I. Shin and J. I. Hong, Org. Lett., 2008, 10, 48.
- Y. M. Chung, B. Raman, D. S. Kim and K. H. Ahn, Chem. Commun., 2006, 186 RSC.
- R. Badugu, J. R. Lakowicz and C. D. Geddes, Anal. Chim. Acta, 2004, 522, 9 CrossRef CAS.
- R. Badugu, J. R. Lakowicz and C. D. Geddes, J. Am. Chem. Soc., 2005, 127, 3635 CrossRef CAS.
- Z. Ekmekci, M. Deniz, Yilmaz and E. U. Akkaya, Org. Lett., 2008, 10, 461 CrossRef CAS.
- P. J. Anzenbacher, D. S. Tyson, K. Jursikova and F. N. Castellano, J. Am. Chem. Soc., 2002, 124, 6232 CrossRef.
- D. L. Recalde-Ruiz, E. Andres-Garcia and M. E. Diaz-Garcia, Quim. Anal., 1999, 18, 111 Search PubMed.
- A. R. Surleva, V. D. Nikolova and M. T. Neshkova, Anal. Chim. Acta, 2007, 583, 174 CrossRef CAS.
- D. Shan, C. Mousty and S. Cosnier, Anal. Chem., 2004, 76, 178 CrossRef CAS.
- A. E. Lindsay and D. O'Hare, Anal. Chim. Acta, 2006, 558, 158 CrossRef CAS.
- A. P. Demchenko, Anal. Biochem., 2005, 343, 1 CrossRef CAS.
- L. Shang, H. Chen and S. Dong, J. Phys. Chem. C, 2007, 111, 10780 CrossRef CAS.
- J. M. Costa-Fernandez, R. Pereiro and A. Sanz-Medel, TrAC, Trends Anal. Chem., 2006, 25, 207 CrossRef.
- Y. Lin, H. Xie, Z. Zhang, Z. Tian and D. Pang, Progr. Chem., 2007, 19, 1861 Search PubMed.
- Z. Lu, C. Li, H. Bao, Y. Qiao, Y. Toh and X. Yang, Langmuir, 2008, 24, 5445 CrossRef CAS.
- W. J. Jin, M. T. Fernandez-Arguelles, J. M. Costa-Fernandez, R. Pereiro and A. Sanz-Medel, Chem. Commun., 2005, 883 RSC.
- W. J. Jin, J. M. Costa-Fernandez, R. Pereiro and A. Sanz-Medel, Anal. Chim. Acta, 2004, 522, 1 CrossRef CAS.
- A. Touceda-Varela, E. I. Stevenson, J. A. Galve-Gasion, D. T. F. Dryden and J. C. Mareque-Rivas, Chem. Commun., 2008, 1998 RSC.
- Q. Zeng, P. Cai, Z. Li, J. Qin and B. Tang, Chem. Commun., 2008, 1094 RSC.
- H. Bao, E. Wang and S. Dong, Small, 2006, 2, 476 CrossRef CAS.
- W. W. Yu, L. Qu, W. Guo and X. Peng, Chem. Mater., 2003, 15, 2854 CrossRef CAS.
-
J. R. Lakowicz, Principles of Fluorescence Spectroscopy, Kluwer Academic/Plenum Press, New York, 1999 Search PubMed.
- Y. Chen and Z. Rosenzweig, Anal. Chem., 2002, 74, 5132 CrossRef CAS.
- M. T. Fernandez-Arguelles, W. J. Jin, J. M. Costa-Fernandez, R. Pereiro and A. Sanz-Medel, Anal. Chim. Acta, 2005, 549, 20 CrossRef.
- B. Tang, J. Niu, C. Yu, L. Zhuo and J. Ge, Chem. Commun., 2005, 4184 RSC.
- Y. Xia, C. Cao and C. Zhu, Chin. J. Chem., 2007, 25, 1836 CrossRef CAS.
- K. Kurmia, D. E. Giles, P. M. May, P. Singh and G. T. Hefter, Talanta, 1996, 43, 2045 CrossRef CAS.
- N. Fujiwara, Y. L. Liu, M. Takabashi and H. Kobayashi, J. Electrochem. Soc., 2006, 153, G394 CrossRef CAS.
-
Guidelines for Drinking-Water Quality, World Health Organization, Geneva, 1996 Search PubMed.
- A. V. Isarov and J. Chrysochoos, Langmuir, 1997, 13, 3142 CrossRef CAS.
Footnote |
† Electronic supplementary information (ESI) available: relative fluorescence intensity of CdTe QDs in the presence of various metal ions, XPS data of Cu2p in the concentrated residual solution after dialyzing the mixture of Cu2+-QDs and KCN, and fluorescence spectra of the copper quenching in the absence and presence of KCN. See DOI: 10.1039/b812458b |
|
This journal is © The Royal Society of Chemistry 2009 |