DOI:
10.1039/B803350A
(Paper)
Analyst, 2009,
134, 80-86
Molecularly imprinted nanocomposites for highly sensitive SPR detection of a non-aqueous atrazine sample
Received
29th February 2008
, Accepted 1st September 2008
First published on 20th October 2008
Abstract
A surface plasmon resonance (SPR) sensor highly sensitive and selective for the herbicide atrazine was composed by immobilizing atrazine-imprinted polymer with gold nanoparticles on a gold thin film as a sensor chip. In the detection, the atrazine-imprinted polymer was expected to work as synthetic receptor for selectively capturing atrazine in organic solvent, and the gold nanoparticles were expected to exhibit a coupling effect with the gold thin film to enhance the local electromagnetic field between the nanoparticles and the gold film, making the sensor chip highly sensitive for changes in microenvironmental polarity. Thus, a combination of the atrazine-imprinted polymer and gold nanoparticles enabled us to compose an SPR sensor demonstrating the detection of 5 pM atrazine in acetonitrile.
Introduction
Surface plasmon resonance (SPR) devices are important signal transducers in biosensors because, in principle, SPR sensing requires no sample probing.1 Recent development of SPR sensors focusing upon a reduction in device size,2 imaging applications,3 and multi-channel sensing4 has increased the significance of SPR biosensors. However, in contrast with the progress made in designing and building new devices, little progress has been made on the design and preparation of molecular recognition elements, the other main component in biosensors.5 A major strategy for achieving molecular recognition in SPR sensors is by immobilization of biomolecules such as nucleic acids,6 enzymes,7 and antibodies.8 These biomolecule-immobilized sensor chips are powerful tools for detecting their specific counterparts and are particularly useful for analyzing the thermodynamics and kinetics of intermolecular interactions between biomolecules.9 However, other materials also should be investigated as molecular recognition elements to overcome some limitations of current SPR sensors.
One subject needed to be addressed in current SPR sensing is the detection of a small molecule. Because a small molecule can induce only a small change in dielectric constants on a sensor chip, detection sensitivity is poor.10 Recently, we have developed a solution using substrate-selective hydrogel with immobilized gold nanoparticles as the molecular recognition elements.11 The putative sensing mechanism involves the affinity of a hydrogel–nanoparticle composite immobilized on a sensor chip for the water-soluble analyte dopamine, used as a molecular template for constructing a tailor-made binding site in the composite material. Upon analyte binding, the material swells to decrease the density (proximity) of gold nanoparticles on the sensor chip,12 enhancing the change in dielectric constants compared to the material without the gold nanoparticles. Similar principles have been utilized in SPR sensors using a gold nanoparticle-modified reagent that approaches and departs from the sensor chip surface when triggered by the presence/absence of an analyte molecule.13 However, the incorporation of gold nanoparticles in the recognition elements is a characteristic feature of our earlier sensor chip, requiring no addition of gold nanoparticle-modified reagents for enhanced SPR sensing.
The successful results obtained by the combination of gold nanoparticles and imprinted polymer hydrogel encouraged investigation into the detection of a low-molecular-weight hydrophobic analyte in organic solvents. Hydrophobic small molecules, such as agrochemicals and pharmaceuticals, are often extracted into organic solvents.14 However, their SPR detection in organic solvents is extremely difficult, where a hydrophobic analyte molecule is bound to a site of molecular recognition elements, replacing an organic solvent molecule. This phenomenon induces only a small change in dielectric constant on a sensor chip surface because both the analyte and solvent molecule possess low polarity. In addition, most biomolecules are not capable of selective uptake of a small molecule in organic solvents. To address this problem, molecularly imprinted polymer (MIP) resins are appropriate materials for selectively capturing a given analyte molecule in organic solvents, while imprinted polymer hydrogels used in our previous study are suited for recognizing a water-soluble compound in aqueous solution. Molecularly imprinted polymer resins have been studied extensively, and exhibit strong affinity toward and high selectivity for capturing small molecules in organic solvents utilizing electrostatic interaction, hydrogen bonding and coordination.15 Because imprinted polymer resins do not drastically swell or shrink upon substrate binding, we planned for this study to employ gold nanoparticles in SPR sensing without depending on proximity changes of the nanoparticles. A recent report indicated that an electromagnetic field can be enhanced via coupling effects between gold nanoparticles and a gold substrate on a sensor chip when the nanoparticles are immobilized in close proximity to the substrate surface.16 This phenomenon has been applied to the SPR detection of small molecules in applications such as gas sensing,17oligonucleotide detection,18 and others.19 Because these examples show that use of the enhanced electromagnetic field is promising in the development of sensitive SPR sensors, general methodology to locate specific binding sites between gold nanoparticles and a gold substrate may provide a universal approach to the sensitive and selective detection of a small molecule.
In this study, the combination of gold nanoparticles with molecularly imprinted polymer resins was expected to allow specific binding sites to be located between the gold nanoparticles and a gold substrate on an SPR sensor chip (Fig. 1). Although binding sites and gold nanoparticles would be randomly located in the imprinted polymer resin by this methodology, a certain number of binding sites would be present in the enhanced electromagnetic field and contribute to sensitive small-molecule detection. This report describes the synthesis of molecularly imprinted polymer resins with immobilized gold nanoparticles on a gold substrate-coated slide glass and outlines its usefulness for SPR sensing of the triazine herbicide atrazine, as a model analyte of a low-molecular-weight chemical species.
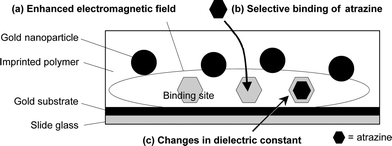 |
| Fig. 1 Schematic representation of the mechanism of angle shift SPR sensing of atrazine using a sensor chip coated with molecularly imprinted polymer containing immobilized gold nanoparticles: (a) some gold nanoparticles near a gold substrate surface enhance the electromagnetic field between the nanoparticles and the substrate; (b) the analyte atrazine is selectively captured by an imprinted polymer membrane; and (c) atrazine molecules accommodated between the nanoparticles and substrate cause sensitive SPR angle shift. | |
Experimental
Reagents and instruments
Atrazine was purchased from Tokyo Kasei Industry (Tokyo, Japan). Acetonitrile (analytical grade), ethylene glycol dimethacrylate, methacrylic acid, 2,2′-azobis(isobutyronitrile) (AIBN), dimethylformamide (DMF), tetraoctylammonium bromide (TOAB), toluene, hydrogen tetrachloroaurate(III) (HAuCl4·4H2O), 11-mercaptoundecanoic acid (MUA), sodium borohydride (NaBH4) and all agrochemicals were purchased from Wako Pure Chemicals (Osaka, Japan). Toluene, DMSO and methacrylic acid were purified by distillation, and AIBN was purified by recrystallization prior to use. All other reagents were used without further purification. The gold-sputtered slide glass (13 × 20 mm) used as a sensor chip was purchased from Nippon Laser & Electronics Lab. (Nagoya, Japan). Transmission electron microscopic (TEM) and scanning electron microscopic (SEM) images were obtained using JEM-2000EX II (JEOL) and JSM-6340F (JEOL) instruments, respectively. An atomic force microscope (JEOL JSPM-4210) was used for measuring the thickness of the polymer nanoparticles composite on the sensor chip.
Into a mixture of a toluene solution (400 mL) of TOAB (3.3 g, 6.0 mmol) and an aqueous solution of HAuCl4·4H2O (15 mM, 200 mL, 3.0 mmol), was gradually added a toluene solution (100 mL) of MUA (655 mg, 3.0 mmol) with vigorous stirring. To the resultant mixture, a freshly prepared aqueous solution of NaBH4 (0.30 M, 100 mL, 30 mmol) was added dropwise. After stirring the mixture for 1 h, the organic phase was separated and washed with distilled water. After removing the solvent by rotary evaporation, the residue was dried in vacuo for one day. The black solid obtained was heat-treated to 155 °C at a rate of 2 °C min−1 for 30 min, and then dissolved in 50 mL of toluene. This solution was added gradually to 800 mL of chloroform to precipitate the gold nanoparticles, removing the excess TOAB and MUA. The nanoparticles were filtered and dried at room temperature.
Preparation of the Au–MIP-coated sensor chip
Atrazine (0.12 mmol), methacrylic acid (1.2 mmol), ethylene glycol dimethacrylate (2.67 mmol), AIBN (10 mg) and the mercaptoundecanoic acid-modified gold nanoparticles (17.4 mg) were added to 1.0 mL of DMF. This pre-polymerization mixture was put between an allyl mercaptan-modified glass slide and a 1-propanethiol-modified glass slide, prepared by immersing gold-sputtered glass slides in ethanolic allyl mercaptan (10 mM) and 1-propanethiol (10 mM), respectively, overnight. A set of glass slides was heated (60 °C, 4 h) under a nitrogen atmosphere with a weight on it. By removing the decanethiol-modified chip, a nanocomposite-coated sensor chip IP17.4 was obtained. Without addition of atrazine, a non-imprinted blank sensor chip BP17.4 was obtained by using the same procedure. The pre-polymerization mixture containing different amounts of gold nanoparticles (0 and 11.6 mg) was also used for preparing the non-imprinted sensor chips BP0 and BP11.6, respectively. All of the sensor chips were immersed in DMF, and washed with acetonitrile after being attached to the SPR sensor.
SPR measurements were conducted using an SPR 670 instrument (Nippon Laser & Electronics Lab) at 24 °C using acetonitrile as a carrier at a flow rate of 15 μL min−1. Samples (20 μL) were prepared in acetonitrile. The average of triplicate measurements was obtained for each sample. The data (shown as a shift in SPR angle) were obtained by subtracting background data of an acetonitrile injection from those obtained from sample injections.
Results and discussion
Preparation of the imprinted polymer nanocomposite-immobilized sensor chip
The sensor chip was designed to locate atrazine-selective binding sites between gold nanoparticles and a gold substrate on a sensor chip, where enhanced electromagnetic fields should increase the sensitivity of an SPR sensor. Cross-linked polymer was utilized as a scaffold for binding sites and gold nanoparticles. A molecular imprinting technique was employed for the formation of atrazine binding sites in the cross-linked polymer.15 A triazine herbicide, atrazine, was chosen as the model analyte for proof-of-principle experiments because of its low molecular weight (FW: 215.69) and hydrophobicity, characteristics that pose difficulties for SPR sensing. Another reason for choosing atrazine is that imprinted polymers used as adsorbents of atrazine have been reported to exhibit high affinity (Kd = 12 μM, in chloroform at room temperature) and selectivity comparable to polyclonal antibodies.20 Typical atrazine analysis involves concentration and purification by liquid–liquid or solid phase extraction, resulting in a sample dissolved in organic solvent.14 Therefore, an atrazine sensor available in organic solvents could be used for detecting atrazine in conventionally prepared sample solutions.
Gold nanoparticles were immobilized within the molecularly imprinted polymer by adding the nanoparticles to the polymerization mixture of the imprinted polymer. The nanoparticle surface had been modified with mercaputoundecanoic acid to increase the solubility of the nanoparticles in DMF during polymerization. Although no reactive functionality for immobilization was introduced, the nanoparticles did not leak out of the imprinted polymer by washing with DMF, methanol, acetonitrile or chloroform, suggesting that the diameter of the nanoparticles is larger than the network size of the imprinted polymer. The mean diameter of the gold nanoparticles was estimated as 4.28 ± 0.98 nm by transmission electron microscopy (TEM) (Fig. 2).
Control of the thickness of the imprinted-polymer nanocomposite membrane is important in this sensor chip design, because the design assumes that analyte molecules diffuse into the nanocomposite membrane to reach the proximity of the gold substrate. For quick response, a thinner nanocomposite membrane is preferable. In addition, a thinner membrane improves sensitivity because a thicker membrane increases insensitive binding sites, located far from the gold substrate, which may capture analyte molecules and reduce the local concentration of the analyte in the sensitive area near the gold substrate. The thickness of the nanocomposite membrane was roughly controlled by a weight placed on the glass slide during polymerization; a heavier weight resulted in a thinner polymer composite film (data not shown). Because an excessively thin nanocomposite membrane was too physically unstable for the SPR measurements, an intermediate thickness was adopted, ca. 300 nm determined by a scanning electron microscope (SEM) study as shown in Fig. 3.
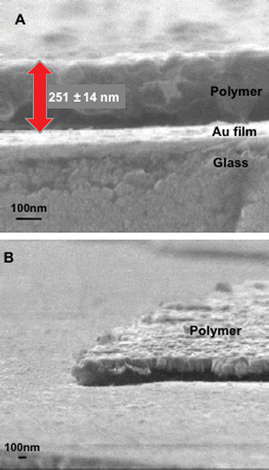 |
| Fig. 3
SEM images of a sensor chip coated with the imprinted polymer–gold nanoparticle composite (IP17.4): (A) side view, (B) slant view. The thickness of the polymer–nanoparticle composite was verified by an AFM measurement. | |
Effect of addition of gold nanoparticles on SPR sensor sensitivity
Polymer–gold nanoparticle composites were prepared with different amounts of gold nanoparticles, and examined as molecular recognition elements of SPR sensor chips to investigate the effect of the gold nanoparticles on the induction of signal transducing ability to the sensor chip. The magnitude of SPR angle shift caused by the presence of atrazine was investigated using non-imprinted blank polymers (BP) prepared without the addition of the molecular template. Non-imprinted blank polymers were used because the atrazine-imprinted polymers were supposed to be unsuitable for simply assessing the effect of the embedded gold nanoparticles on SPR responses; varying amounts of gold nanoparticles, i.e. varying the amount of gold nanoparticle-tethered carboxyl groups, would result in varying efficiency of the molecular imprinting process, which could also affect the sensor sensitivity. Typical sensorgrams obtained upon injection of atrazine (100 μM) are shown in Fig. 4. In the sensorgrams, BP0 exhibited no significant shift in SPR angle, while BP11.6 and BP17.4 produced a maximum increase in SPR angle at ca. 100 s after the sample reached the sensor chip. The increase in SPR angle could be attributed to a change in dielectric constant near the sensor chip surface caused by exchange of the carrier acetonitrile molecules with atrazine. (A decrease in the SPR angle immediately after the sample reached the sensor chip also was observed, although the cause of the decrease is not understood.) The observed response time (ca. 100 s) may be due to the diffusion of analyte molecules to the proximity of the gold substrate.
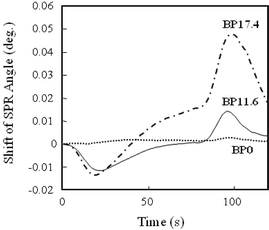 |
| Fig. 4 Typical sensorgrams of non-imprinted blank polymer nanocomposites (BP0, BP11.6, BP17.4) with different densities of embedded gold nanoparticles. The carrier used was acetonitrile at a flow rate of 15 μL min−1. Measurements were conducted at 24 °C on a 20 μL sample of a 100 μM atrazine solution in acetonitrile. | |
A comparison of BP11.6 and BP17.6 suggested that the addition of more gold nanoparticles affords a sensor chip more sensitive to microenvironmental changes on its surface. The trend could be explained by that assuming a greater density of gold nanoparticles increases the number of nanoparticles that interact with the gold substrate to generate an enhanced electromagnetic field, thus increasing the number of binding sites in the enhanced electromagnetic field. Based on this, an attempt was made to prepare a sensor chip with a density of gold nanoparticles greater than 17.4 mg mL−1; however, the resultant sensor chip exhibited an SPR angle too large for the measurable range of the instrument employed.
Effect of molecular imprinting on SPR sensor sensitivity
A molecularly imprinted polymer nanocomposite was compared to the non-imprinted blank nanocomposite as a sensing material. Both sensor chips were prepared using the same density of gold nanoparticles and treated in an identical manner. Upon injection of atrazine acetonitrile solution, the imprinted sensor chip (IP17.4) exhibited a larger shift in SPR angle compared to the non-imprinted blank chip (BP17.4); upon injection of 0.5 nM atrazine, BP17.4 exhibited no distinct increase, while IP17.4 showed a 0.012° increase at 100 s after the sample reached the sensor chip. The response of both sensor chips was dependent on atrazine concentration as shown in Fig. 5, confirming that the response observed was due to the presence of atrazine.
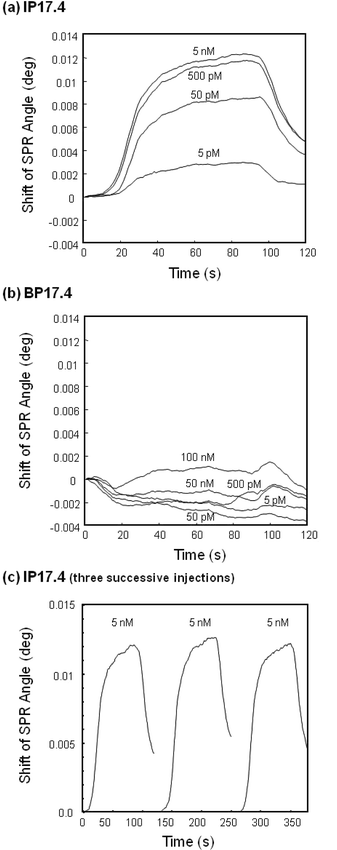 |
| Fig. 5 Typical sensorgrams of various concentrations of atrazine on (a) the atrazine-imprinted polymer nanocomposite (IP17.4) and (b) the non-imprinted blank polymer nanocomposite (BP17.4), and (c) typical sensorgrams of 5 nM atrazine upon three successive injections. The carrier was acetonitrile at a flow rate of 15 μL min−1, and the sample size was 20 μL. Measurements were conducted at 24 °C. | |
The calibration curves for both sensor chips also were compared (Fig. 6), and the results indicated that the imprinted polymer–gold nanoparticle composite can detect atrazine at a concentration of 5 pM. Although the non-imprinted sensor chip also detected atrazine, its sensitivity was significantly lower, resulting in smaller changes in SPR angle with an increase of atrazine concentration. This result strongly suggests that molecular imprinting was effective for enhancing the atrazine-sensitivity of the sensor chip. In the imprinted polymer, monomer molecules (methacrylic acid) assemble around a molecular template and become immobilized by cross-linking, forming a tailor-made binding site for the molecular template. According to previous reports, an atrazine-imprinted polymer prepared with a similar monomer composition exhibits affinity (Kd = 12 μM, at room temperature) in chloroform,20 while a carboxylic acid (e.g.acetic acid) alone displays Kd as 7 mM (although a carboxyl moiety immobilized in BP may exhibit a different affinity from that of acetic acid).21 Because our atrazine-imprinted nanocomposite was prepared in DMF (the previous imprinted polymer was prepared in chloroform20) and atrazine sensing was conducted in acetonitrile, it would exhibit different affinity for atrazine compared with the previously reported polymers. However, the larger response obtained by IP17.4 can be explained by the higher affinity of the imprinted polymer membrane for atrazine, which more effectively concentrates atrazine in the polymer composite membrane, leading to more distinct microenvironmental changes on the sensor chip.
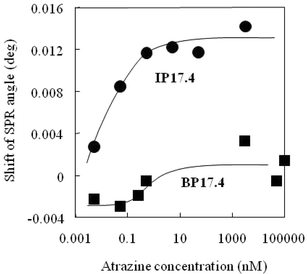 |
| Fig. 6 Calibration curves for the non-imprinted blank polymer nanocomposite (BP17.4) and the imprinted polymer nanocomposite (IP17.4). The carrier was acetonitrile at a flow rate of 15 μL min−1, and the sample size was 20 μL. Measurements were conducted at 24 °C. | |
There have been several reports on atrazine sensors based on other recognition elements and transducers, e.g. bilayer lipid membranes with electrochemical detection (nanomolar level),22 a P450 mRNA-specific oligonucleotide probe with RU-change SPR (4.6 pM),23 and immunoreactions with a waveguide Mach-Zender interferometer (460 pM).24 The sensitivity of our sensor is comparable to these sensors, demonstrating the effectiveness of this atrazine sensor.
Selectivity of the Au–MIP-coated sensor chip
Atrazine-imprinted polymers have been reported independently by several groups, indicating that the imprinted polymers are highly selective for atrazine.20 Also, several selective herbicide sensors based on imprinted polymers have been reported, that employ signal transducers based on amperometry,25conductimetry,26differential-pulse voltammetry,27 and infrared evanescent wave spectroscopy.28 Because our sensor chip is composed of an atrazine-imprinted polymer with a composition similar to those in previous reports, it is likely to exhibit selectivity toward atrazine. Thus, the selectivity of IP17.4 was examined using agrochemicals such as asulam, tetrachloroisophthalonitrile (TPN), 2,6-di-tert-butyl-p-tolyl methylcarbamate (MBPMC), pendimethalin, flutolanil and chlorpyriphos as references (for their structures, see Fig. 7). Fig. 8 summarizes the relative SPR angle shift upon injection of the agrochemicals.
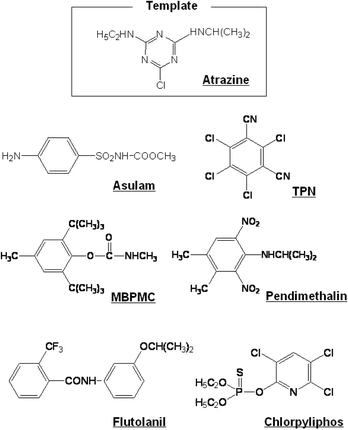 |
| Fig. 7 Structure of atrazine and agrochemicals used for assessing the selectivity of the imprinted polymer-based sensor chip (IP17.4) and the non-imprinted polymer-based one (BP17.4). | |
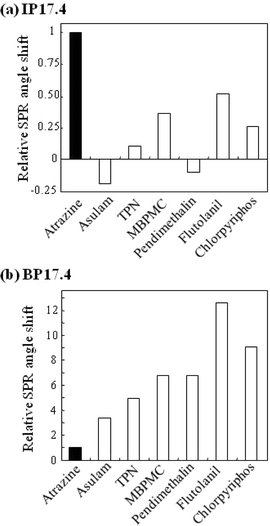 |
| Fig. 8 Selectivity of nanocomposites on (a) the imprinted polymer (IP17.4) and (b) the non-imprinted polymer (BP17.4). The relative SPR angle shift was calculated by dividing the SPR angle shift upon injection of each sample by that upon injection of atrazine. Sample concentration was 50 nM and 12.5 μM for IP17.4 and BP17.4, respectively. | |
As shown in Fig. 8, atrazine resulted in the largest response among the samples tested in IP17.4, while the non-imprinted sensor chip BP17.4 exhibited the smallest response to atrazine. Interestingly, the order of the SPR angle shift in BP17.4 roughly depended on molecular weight: agrochemicals with a higher molecular weight caused a larger response (with the exception of chlorpyriphos that exhibited a smaller response despite a larger molecular weight). The distinctly different preference between IP17.4 and BP17.4 suggests that the atrazine selectivity observed in IP17.4 was induced by molecular imprinting.
Conclusions
This study demonstrated angle-shift SPR sensing of the herbicide atrazine, using a substrate-selective polymer resin with immobilized gold nanoparticles. The gold nanoparticles were effective in inducing angle-shift SPR sensitivity to atrazine bound on a sensor chip. The substrate-selective polymer was synthesized by a molecular imprinting technique, which enhanced sensitivity and selectivity for atrazine. Although a direct comparison in sensitivity cannot be made among unoptimized sensors, our SPR sensor appeared to be much more sensitive compared to previously reported atrazine sensors based on molecularly imprinted polymers.25,26 Because the molecular imprinting technique can be applied to other target species by choosing the targets as templates in the polymer synthesis, highly sensitive and selective SPR sensors can be developed using methodology combining molecularly imprinted polymers with gold nanoparticles.
This study focused on the proof-of-principle by demonstrating the effectiveness of the nanocomposite material for SPR sensing of a small molecule in an organic solvent. However, the significance of an atrazine sensing system available in acetonitrile can be questioned; an ideal herbicide sensor would be one that can directly sense a herbicide in substances such as water, soil, and juice, with sufficient sensitivity and selectivity, requiring no sample concentration or purification or use of organic solvents before analysis. However, it is currently practical to combine a sensitive sensor available in an organic solvent with a conventional sample preparation procedure. Because our sensor can detect atrazine at a low concentration that is believed not to be biologically hazardous, the sample preparation procedure does not require a concentration process for enrichment of the sample. This suggests that our sensor can reduce the amount of organic solvents and adsorbent materials used for sample preparation, and therefore is an environmental friendly (or ‘green’) herbicide analysis process.
References
- P. B. Daniels, J. K. Deacon, M. J. Eddowes and D. G. Pedley, Sens. Actuators, 1988, 15, 11–18 CrossRef CAS.
- E. Fujii, T. Koike, K. Nakamura, S. Sasaki, K. Kurihara, D. Citterio, Y. Iwasaki, O. Niwa and K. Suzuki, Anal. Chem., 2002, 74, 6106–6110 CrossRef CAS.
-
(a) S. Fang, H. J. Lee, A. W. Wark and R. M. Corn, J. Am. Chem. Soc., 2006, 128, 14044–14046 CrossRef CAS;
(b) H. J. Lee, Y. Li, A. Wark and W. R. M. Corn, Anal. Chem., 2005, 77, 5096–5100 CrossRef CAS;
(c) S. D. Evans, H. Allinson, N. Boden, T. M. Flynn and J. R. Henderson, J. Phys. Chem. B, 1997, 101, 2143–2148 CrossRef CAS;
(d) C. E. Jordan and R. M. Corn, Anal. Chem., 1997, 69, 1449–1456 CrossRef CAS.
-
(a) R. Ince and R. Narayanaswamy, Anal. Chim. Acta, 2006, 569, 1–20 CrossRef CAS;
(b) A. N. Naimushin, S. D. Soelberg, D. U. Bartholomew, J. L. Elkind and C. E. Furlong, Sens. Actuators, B, 2003, 96, 253–260 CrossRef;
(c) H. J. Lee, T. T. Goodrich and R. M. Corn, Anal. Chem., 2001, 73, 5525–5531 CrossRef CAS.
-
(a) J. Homola, Anal. Bioanal. Chem., 2003, 377, 528–539 CrossRef CAS;
(b) R. L. Rich and D. G. Myszka, Curr. Opin. Biotechnol., 2000, 11, 54–61 CrossRef CAS.
-
(a) P. Gong, C.-Y. Lee, L. J. Gamble, D. G. Castner and D. W. Grainger, Anal. Chem., 2006, 78, 3326–3334 CrossRef CAS;
(b) R. Georgiadis, K. P. Peterlinz and A. W. Peterson, J. Am. Chem. Soc., 2000, 122, 3166–3173 CrossRef CAS.
-
(a) R. Kurita, Y. Yokota, Y. Sato, F. Mizutani and O. Niwa, Anal. Chem., 2006, 78, 5525–5531 CrossRef CAS;
(b) F. Xu, G. Zhen, F. Yu, E. Kuennemann, M. Textor and W. Knoll, J. Am. Chem. Soc., 2005, 127, 13084–13085 CrossRef CAS.
-
(a) G. Maes, G. Borghs and S. Muyldermans, Anal. Chem., 2005, 77, 7547–7555 CrossRef CAS;
(b) S. Chen, L. Liu, J. Zhou and S. Jiang, Langmuir, 2003, 19, 2859–2864 CrossRef CAS;
(c) W. F. M. Stöcklein, A. Warsinke, B. Micheel, G. Kempter, W. Höhne and F. W. Scheller, Anal. Chim. Acta, 1998, 362, 101–111 CrossRef.
- Y. Tang, R. Mernaugh and X. Zeng, Anal. Chem., 2006, 78, 1841–1848 CrossRef CAS.
-
(a) M. Uttamchandani, D. P. Walsh, S. M. Khersonsky, X. Huang, S. Q. Yao and Y.-T. J. Chang, Comb. Chem., 2004, 6, 862–868 Search PubMed;
(b) A. Kugimiya and T. Takeuchi, Biosens. Bioelectron., 2001, 16, 1059–1062 CrossRef CAS.
- J. Matsui, K. Akamatsu, N. Hara, D. Miyoshi, H. Nawafune, K. Tamaki and N. Sugimoto, Anal. Chem., 2005, 77, 4282–4285 CrossRef CAS.
- J. Matsui, K. Akamatsu, S. Nishiguchi, D. Miyoshi, H. Nawafune, K. Tamaki and N. Sugimoto, Anal. Chem., 2004, 76, 1310–1315 CrossRef CAS.
-
(a) T. Kang, S. Hong, I. Choi, J. J. Sung, Y. Kim, J.-S. Hahn and J. Yi, J. Am. Chem. Soc., 2006, 128, 12870–12878 CrossRef CAS;
(b) L. He, M. D. Musick, S. R. Nicewarner, F. G. Salinas, S. J. Benkovic, M. J. Natan and C. D. Keating, J. Am. Chem. Soc., 2000, 122, 9071–9077 CrossRef CAS.
-
(a) R. D. Harris, B. J. Luff, J. S. Wilkinson, J. Piehler, A. Brecht, G. Gauglitz and R. A. Abuknesha, Biosens. Bioelectron., 1999, 14, 377–386 CrossRef CAS;
(b) C. Sheedy, K. Y. F. Yau, T. Hirama, C. R. MacKenzie and J. C. Hall, J. Agric. Food Chem., 2006, 54, 3668–3678 CrossRef CAS;
(c) T.-K. Lim, M. Oyama, K. Ikebukuro and I. Karube, Anal. Chem., 2000, 72, 2856–2860 CrossRef CAS;
(d) G. Strachan, S. D. Grant, D. Learmonth, M. Longstaff, A. J. Porter and W. J. Harris, Biosens. Bioelectron., 1998, 13, 665–673 CrossRef CAS;
(e) V. I. Chegel, Yu. M. Shirshov, E. V. Piletskaya and S. A. Piletsky, Sens. Actuators, B, 1998, 48, 456–460 CrossRef.
-
(a)
Molecular Imprinting-From Fundamentals to Applications, ed. M. Komiyama, T. Takeuchi, T. Mukawa and H. Asanuma, Wilely-VCH, Weinheim, Germany, 2002 Search PubMed;
(b)
B. Sellergren, Molecularly Imprinted Polymers: Man-Made Mimics of Antibodies and their Application in Analytical Chemistry, Elsevier, Amsterdam, 2001 Search PubMed;
(c) S. A. Piletsky, S. Alcock and A. P. F. Turner, Trends Biotechnol., 2001, 19, 9–12 CrossRef CAS;
(d) K. Haupt and K. Mosbach, Chem. Rev., 2000, 100, 2495–2504 CrossRef CAS;
(e) K. Haupt and K. Mosbach, Trends Biotechnol., 1998, 16, 468–475 CrossRef CAS;
(f) G. Wulff, Angew. Chem., Int. Ed. Engl., 1995, 34, 1812–1832 CrossRef CAS;
(g) K. J. Shea, Trends Polym. Sci., 1994, 2, 166 CAS.
-
(a) L. A. Lyon, D. J. Peña and M. J. Natan, J. Phys. Chem. B, 1999, 103, 5826–5831 CrossRef CAS;
(b) T. Takemori, M. Inoue and K. Ohtaka, J. Phys. Soc. Jpn., 1987, 56, 1587–1602 CrossRef CAS;
(c) W. R. Holland and D. G. Hall, Phys. Rev. B, 1983, 27, 7765–7768 CrossRef CAS;
(d) R. W. Rendell and D. J. Scalapino, Phys. Rev. B, 1981, 24, 3276–3294 CrossRef CAS;
(e) C. R. Evans, T. A. Spurlin and B. L. Frey, Anal. Chem., 2002, 74, 1157–1164 CAS;
(f) C. A. Neugebauer and M. B. Webb, J. Appl. Phys., 1962, 33, 74–79 CAS;
(g) H. Wohltjen and A. W. Snow, Anal. Chem., 1998, 70, 2856–2859 CrossRef CAS;
(h) Y. Joseph, I. Besnard, M. Rosenberger, B. Guse, H.-G. Nothofer, J. M. Wessels, U. Wild and T. Vossmeyer, J. Phys. Chem. B, 2003, 107, 7406–7413 CrossRef CAS;
(i) F. P. Zamborini, M. C. Leopold, J. F. Hicks, P. J. Kulesza, M. A. Malik and R. W. Murray, J. Am. Chem. Soc., 2002, 124, 8958–8964 CrossRef CAS;
(j) H. Ahn, A. Chandekar, B. Kang, C. Sung and J. E. Whitten, Chem. Mater., 2004, 16, 3274–3278 CrossRef CAS;
(k) L. Han, D. R. Daniel, M. M. Maye and C.-J. Zhong, Anal. Chem., 2001, 73, 4441–4449 CrossRef CAS;
(l) T. Okamoto and I. Yamaguchi, J. Phys. Chem. B, 2003, 107, 10321–10324 CrossRef CAS;
(m) E. Hutter, J. H. Fendler and D. Roy, J. Phys. Chem. B, 2001, 105, 11159–11168 CrossRef CAS;
(n) L. He, M. D. Musick, S. R. Nicewarner, F. G. Salinas, S. J. Benkovic, M. J. Natan and C. D. Keating, J. Am. Chem. Soc., 2000, 122, 9071–9077 CrossRef CAS;
(o) J. C. Tsang, J. R. Kirtly and J. A. Bradly, Phys. Rev. Lett., 1979, 43, 772–775 CrossRef CAS;
(p) P. E. Batson, Phys. Rev. Lett., 1982, 49, 936–940 CrossRef CAS.
-
(a) J. M. Rooney and E. A. H. Hall, Anal. Chem., 2004, 76, 6861–6870 CrossRef CAS;
(b) C. K. Chen, A. R. B. Castro and Y. R. Shen, Phys. Rev. Lett., 1981, 46, 143–148;
(c) Y. Y. Teng and E. A. Stern, Phys. Rev. Lett., 1967, 19, 511–514 CrossRef;
(d) R. H. Ritchie, E. T. Arakawa, J. J. Cowan and R. H. Hamm, Phys. Rev. Lett., 1968, 21, 1530–1533 CrossRef CAS.
- A. Yamaguchi, S. Juodkazis, S. Matsuo and H. Misawa, Chem. Lett., 2002, 190–191 CrossRef CAS.
-
(a) X. Li, K. Tamada, A. Baba, W. Knoll and M. Hara, J. Phys. Chem. B, 2006, 110, 15755–15762 CrossRef CAS;
(b) L. A. Lyon, M. D. Musick and M. J. Natan, Anal. Chem., 1998, 70, 5177–5183 CrossRef CAS.
-
(a) J. Matsui, K. Fujiwara and T. Takeuchi, Anal. Chem., 2000, 72, 1810–1813 CrossRef CAS;
(b) M. Siemann, L. I. Andersson and K. Mosbach, J. Agric. Food Chem., 1996, 44, 141–145 CrossRef CAS;
(c) M. T. Muldoon and L. H. Stanker, J. Agric. Food Chem., 1995, 43, 1424–1427 CrossRef CAS;
(d) J. Matsui, Y. Miyoshi, O. Doblhoff-Dier and T. Takeuchi, Anal. Chem., 1995, 67, 4404–4408 CrossRef CAS.
-
(a) G. J. Welhouse and W. F. Bleam, Environ. Sci. Technol., 1993, 27, 494–500 CAS;
(b) G. J. Welhouse and W. F. Bleam, Environ. Sci. Technol., 1993, 27, 500–505 CAS.
- C. G. Siontorou, D. P. Nikolelis, U. J. Krull and K.-L. Chiang, Anal. Chem., 1997, 69, 3109–3114 CrossRef CAS.
- T.-K. Lim, M. Oyama, K. Ikebukuro and I. Karube, Anal. Chem., 2000, 72, 2856–2860 CrossRef CAS.
- E. F. Schipper, S. Rauchalles, R. P. H. Kooyman, B. Hock and J. Greve, Anal. Chem., 1998, 70, 1192–1197 CrossRef CAS.
- R. Shoji, T. Takeuchi and I. Kubo, Anal. Chem., 2003, 75, 4882–4886 CrossRef CAS.
-
(a) S. A. Piletsky, E. V. Piletskaya, A.
V. Elgersma, K. Yano, I. Karube, Yu. P. Parhometz and A. V. El'skaya, Biosens. Bioelectron., 1995, 10, 959–964 CrossRef CAS;
(b) T. A. Sergeyeva, S. A. Piletsky, A. A. Brovko, E. A. Slinchenko, L. M. Sergeeva, T. L. Panasyuk and A. V. El'skaya, Analyst, 1999, 124, 331–334 RSC.
- S. Kroger, A. P. F. Turner, K. Mosbach and K. Haupt, Anal. Chem., 1999, 71, 3698–3702 CrossRef CAS.
- M. Jakusch, M. Janotta, B. Mizaikoff, K. Mosbach and K. Haupt, Anal. Chem., 1999, 71, 4786–4791 CrossRef CAS.
|
This journal is © The Royal Society of Chemistry 2009 |