Photobleaching kinetics, photoproduct formation, and dose estimation during ALA induced PpIX PDT of MLL cells under well oxygenated and hypoxic conditions
Received
25th August 2005
, Accepted 14th October 2005
First published on 15th November 2005
Abstract
Fluorescence photobleaching and photoproduct formation were investigated during δ-aminolevulinic acid (ALA) induced protoporphyrin IX (PpIX) PDT of MLL cells in vitro. Cells were incubated in either 0.1 or 1.0 mM ALA for 4 h and were treated with 532 nm or 635 nm light under well oxygenated or hypoxic conditions. Fluorescence spectra were acquired during treatment. Photobleaching and photoproduct formation were quantified using singular value decomposition fitting of fluorescence spectra to experimentally determined basis spectra for PpIX, photoprotoporphyrin (Ppp), product II (peak at 655 nm), and product III (peak at 618 nm). PpIX photobleaching occurred under both normal and hypoxic conditions. The photobleaching kinetics could not be explained by purely first- or second-order photobleaching kinetics, and were attributed to differences in PpIX binding at the two ALA incubation concentrations. Ppp was the main photoproduct and accumulated in higher levels in the absence of oxygen, likely a result of reduced Ppp photobleaching under hypoxia. Increases in product II fluorescence occurred mainly in the presence of oxygen. To assess potential fluorescence based PDT dose metrics, cell viability was measured at select times during treatment using a colony formation assay. Cell survival correlated well to changes in product II fluorescence, independent of oxygenation, sensitizer concentration, and treatment wavelength, suggesting that this product is primarily a result of singlet oxygen mediated reactions and may potentially be useful to quantify singlet oxygen dose during PDT.
Introduction
In the past decade there has been tremendous interest in δ-aminolevulinic acid (ALA) based PDT.1–3 ALA itself is not a photosensitizer, but a naturally occurring precursor in the biosynthetic pathway for heme production. The last step in heme production involves conversion of protoporphyrin IX (PpIX), a photosensitizing species, to heme. Under physiologic conditions, free heme acts by negative feedback to control ALA synthesis. When exogenous ALA is administered, the control mechanism is bypassed, and heme precursors are synthesized in excess. Under these conditions, the rate limiting step is the insertion of iron into PpIX by ferrochelatase. Since ferrochelatase is unable to compensate for the excess PpIX that is formed, high levels of PpIX accumulate in cells, rendering them photosensitive.
The synthesis of PpIX from ALA is affected by many factors, including pH,2,4,5 hypoxia,4,6 temperature,7 glucose,8 cell cycle and cell type.9 Unfortunately, the variation in synthesis makes PDT dosimetry based solely on administered ALA and delivered light fluence inaccurate. Much work has been done to measure accumulated PpIX in tissue before treatment, but this measure alone may be insufficient to predict therapeutic outcome, since PDT dose also depends on the amount of oxygen available in the tissue.10 As with other photosensitizers, ALA-PpIX induced biological damage depends on the production of singlet oxygen and other oxygen radicals, and therefore depends on the availability of ground state oxygen and the irradiance of treatment light. Incorporating these measures into an explicit dose metric is difficult since PpIX concentration, oxygen, and light fluence are difficult to measure in vivo at all times during treatment. Alternatively, PDT dose estimates may be based on either a direct or indirect measure of singlet oxygen.11 We have shown previously that photobleaching kinetics can be used to calculate singlet oxygen dose during in vitro PDT of MLL cells with mTHPC and Photofrin for a wide range of treatment conditions.12–14 In this work we have investigated the photobleaching of PpIX during PDT under a variety of treatment conditions, and assessed whether photosensitizer fluorescence photobleaching or photoproduct formation are appropriate candidates for estimating PDT dose during ALA based PDT.
Methods
Chemicals
A 10 mg ml−1 stock solution of δ-aminolevulinic acid (ALA) (Sigma-Aldrich, St. Louis MO, USA) was prepared by dissolving the sensitizer in phosphate buffered saline (PBS). The solution was kept frozen until needed. For PDT experiments, further dilutions were made in cell medium supplemented with 20 mM Hepes buffer (Gibco-BRL, Grand Island, NY USA), but without fetal bovine serum (FBS) (Gibco-BRL).
Cell culture
MAT-LyLu (MLL) rat prostate adenocarcinoma cells were grown in RPMI medium 1640 (Gibco-BRL) supplemented with 10% FBS (Gibco-BRL) and 2% antibiotic-antimycotic (Gibco-BRL) and 10 mM Hepes buffer (Gibco-BRL). For PDT, 6 × 106 cells were plated in a 150 mm cell culture dish and allowed to attach. Medium was removed and cells were washed once with PBS. Serum free medium prepared with a known concentration of ALA was added and cells were incubated in the dark. After 4 h, the medium was removed and 5 ml of 0.1% trypsin was added to detach the cells from the cell plate. After 5 min, 20 ml of serum free medium was added to the plate and the resulting suspension was centrifuged. The supernatant was removed and the cells were re-suspended in PBS to a concentration of 2 × 106 ml−1.
Irradiation apparatus
The light irradiation apparatus has been described previously.12 All handling of cells and PDT irradiations were performed in a sterile laminar flow hood. 1.5 ml of cell suspension were put into a sterile 4 ml cuvette. The cuvette was held in place with a cuvette holder and the cells were kept in suspension with a magnetic stir bar. The mechanical action of the stir bar had no effect on cell viability of photosensitized cells when measured after 1 h of stirring in the absence of light. On one face of the cuvette was a lens coupled fiber that delivered light to the sample.
Cell survival assay
At selected times during PDT, a 50 µl cell sample was withdrawn from the cuvette and diluted in 10 ml of medium. A volume was plated in triplicate in a six well tissue culture dish so that the total number of cell colonies would not exceed 400. Additional medium was added to make the final volume 3 ml. After 4 d of dark incubation, the medium was removed and the colonies were fixed and stained with 0.05% methylene blue dye. Colonies were counted on a light microscope with a translational stage.
Light source
The treatment and fluorescence excitation light were produced by a diode-pumped solid state frequency-doubled single mode laser (Alphalas GmbH, Gottingen, Germany) with a continuous wave output of 60 mW at 532 nm. The fluence rate was determined at the incident face of the cuvette with a hand held optical power meter (Newport Corporation, Fountain Valley, CA USA) with calibration traceable to the National Institutes of Standards and Technology (NIST, Gaithersburg, MD USA). The average fluence rate was 18.5 ± 0.5 mW cm−2. For treatments at 635 nm, a fiber coupled 635 nm diode laser with a maximum output of 200 mW was used for the treatment light. The average fluence rate of the 635 nm source was 13 mW cm−2. In these experiments the treatment light was interrupted every 30 s for approximately 1 s to use 532 nm light for excitation and measurement of the fluorescence spectra.
Fluorescence analysis
Perpendicular to the fluorescence excitation light was a lens coupled fiber used to collect fluorescent light. Fluorescence spectra from the sample were acquired with a USB2000 Ocean Optics spectrometer (Ocean Optics Inc., Dunedin, FL USA) with a spectral range of 600 to 890 nm. The measured fluorescence spectra were analyzed as a linear combination of basis spectra using a singular value decomposition (SVD) fitting routine implemented in MATLAB (V 7.04). The basis spectra used in the fitting, shown in Fig. 1, were those for PpIX, photoprotoporphyrin (Ppp), product II (peak at 655 nm), product III (peak at 618 nm), MLL cellular autofluorescence, and a spectrum equal to the first derivative of the PpIX basis spectrum to correct for small wavelength shifts of the PpIX spectrum. An additional basis spectrum was used to account for instrument background due to fluorescence of the optical components (not shown in Fig. 1 for clarity). The spectra were not corrected for instrument response.
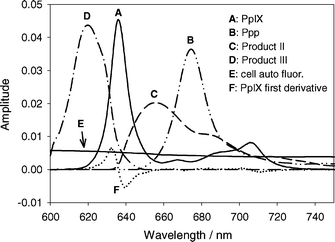 |
| Fig. 1 Basis spectra used in SVD fitting of acquired spectra. Spectra are identified in the legend. All the spectra have amplitudes equal to one. Spectra A–E have an area under the curve between 610 nm and 750 nm equal to one. The amplitude of F is such that its addition to spectrum A will shift A by 1 nm. Spectra are not corrected for instrument response. | |
The cellular and instrument autofluorescence basis spectra were constructed from an average of ten fluorescence measurements of unsensitized MLL cells suspended in PBS and a cuvette containing only PBS respectively. The PpIX basis spectrum was constructed from an average of six fluorescence measurements of MLL cells incubated in 1.0 mM ALA for 4 h and suspended in PBS before PDT. The autofluorescence spectra were subtracted from each acquired spectrum. To account for small wavelength shifts in the PpIX spectrum, an additional basis spectrum equal to the first derivative of the PpIX spectrum was included in the SVD fitting. For small wavelength shifts, it can be shown that a linear combination of the PpIX basis spectrum and its first derivative is equivalent to a shifted version of the basis spectrum. The amplitude of this spectrum could be used to estimate the shift in each spectrum and the largest wavelength shift observed in any experiment was 0.3 nm.
The Ppp and product III basis spectra were determined by subtracting fluorescence spectra acquired during PDT under hypoxic conditions (at fluences less than 10 J cm−2) from initial fluorescence spectra. Autofluorescence was subtracted and the acquired spectra were scaled so that the PpIX amplitude matched that of the initial spectrum (pre-PDT). The resulting difference spectra showed two distinct peaks, one centered at ∼618 nm (product III), and one centered at 674 nm (Ppp). The spectra were scaled so that the peak amplitudes matched for each acquired spectrum. The basis spectra were determined from the average of 12 fluorescence spectra acquired from three separate experiments.
The product II basis spectrum was determined in a similar fashion to Ppp and product III, except the fluorescence spectra were acquired under well oxygenated conditions. The scaled Ppp, PpIX, product III and autofluorescence basis spectra were subtracted from the acquired spectra. The basis spectrum was determined from the average of 10 spectra acquired from two independent experiments.
The measured basis spectra were smoothed using SigmaPlot and the amplitudes were normalized so that the area beneath each basis spectrum between 610 nm and 750 nm was unity. The PpIX first derivative spectrum was scaled so that an amplitude of one would shift the PpIX basis spectrum by 1 nm.
Experiments under hypoxic conditions
Oxygen pressure was reduced by flowing 100% nitrogen gas into the cell sample. Oxygen concentration was measured with a Clark style oxygen electrode (product number 730, Diamond General Corp., Ann Arbor, MI). The electrode was controlled by a chemical sensor (Diamond General Corp), which was used to set the polarization voltage of the sensor and to adjust the oxygen calibration points. Oxygen concentration was below measurable levels (< 0.5%) after 20 min of nitrogen flow, and it was monitored to insure stability. When hypoxia was maintained for 30 min (longer than any PDT treatment) it had no effect on the viability of photosensitized cells in the absence of light.
Results
Spectral fitting and analysis
Fig. 2a shows a typical acquired spectrum (curve 1) and the fit basis spectra for MLL cells incubated in 1.0 mM ALA under hypoxic conditions before PDT. The main component of the acquired fluorescence spectrum is PpIX (curve A). The initial amplitude of Ppp (curve B) is 1.7% of the amplitude of PpIX. The amplitudes of product II and product III were less than 1% of the PpIX amplitude, and the amplitude of the cell autofluorescence basis spectrum was 8% of PpIX. The fit product II, product III, and autofluorescence basis spectra were removed from Fig. 2a for clarity. The PpIX first derivative (curve F) has an amplitude of 10% relative to the PpIX basis spectrum amplitude, corresponding to a shift of 0.10 nm.
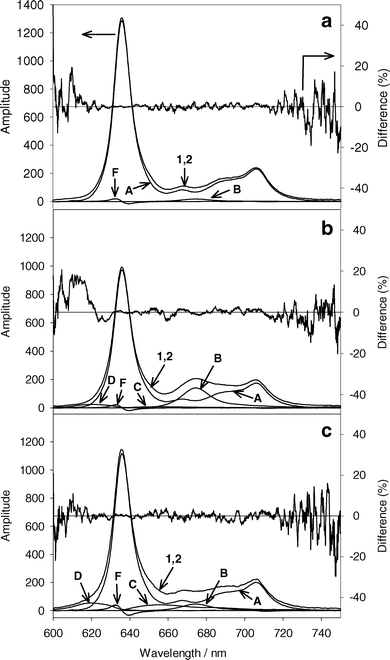 |
| Fig. 2 (a) A representative initial acquired fluorescence spectrum from MLL cells incubated in 1.0 mM ALA for 4 h (curve 1). Curve 2 is the sum of the basis spectra in the best fit to curve 1 using SVD. Cells were made hypoxic before acquiring the spectrum. The percent difference of the best fit from the acquired spectrum is given on the right axis. (b) The same experiment following 13 J cm−2 532 nm light under hypoxic conditions. (c) Acquired spectrum from MLL cells incubated in 1.0 mM ALA and treated with 13 J cm−2 532 nm light under well oxygenated conditions. The contributing basis spectra to the best fits are indicated by A–F, corresponding to the basis spectra in Fig. 1. Basis spectra with small amplitudes are not shown for clarity. | |
Fig. 2b shows the acquired spectrum (curve 1) and fit basis spectra for the same experiment shown in Fig. 2a after 13 J cm−2 of 532 nm light under hypoxic conditions. The amplitude of the PpIX basis spectra (curve A) is reduced to 75% of the initial amplitude. There is a significant increase in Ppp (curve B), and small increases in product II and product III (curves C and D). For a similar experiment performed under well oxygenated conditions (Fig. 2c), there is noticeably less Ppp accumulation for a similar decrease in PpIX, but higher levels of product II and product III are found.
PpIX production
The fit of the PpIX basis spectrum to the fluorescence spectra from sensitized cell samples before PDT showed no change in PpIX concentration as a result of making the cell suspension hypoxic (Fig. 3). The ratio of the PpIX amplitude for ALA incubation concentration of 1.0 mM to that for 0.1 mM was 3.8.
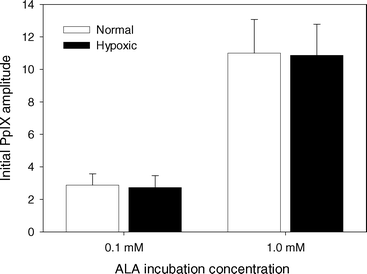 |
| Fig. 3 Initial PpIX basis spectrum amplitude normalized to cell autofluorescence basis spectrum amplitude for MLL cells incubated in 0.1 or 1.0 mM ALA for 4 h. Spectra were acquired either under well oxygenated conditions (white) or after cells were made hypoxic (black). Error bars represent ±1 standard deviation from a minimum of 3 independent experiments. | |
PpIX photobleaching
Fig. 4a shows the change in PpIX amplitude for experiments under both hypoxic and well oxygenated conditions for ALA incubation concentrations of 0.1 and 1.0 mM. The PpIX amplitudes are normalized to initial cell autofluorescence amplitude to account for small variations in the number of cells in the cuvette. PpIX photobleaching occurred during all experiments. At high initial PpIX concentrations, more bleaching occurred for experiments under hypoxic conditions than under well oxygenated conditions. At low initial PpIX concentrations, the opposite effect was observed. Fig. 4b shows the data in Fig. 4a normalized to the initial PpIX amplitude. For experiments under well oxygenated conditions, a greater fraction of PpIX was bleached at low PpIX concentrations for the same fluence. Normalized PpIX photobleaching was independent of initial PpIX concentration under hypoxic conditions. These experiments could be fit by a single exponential decay curve, suggesting that oxygen independent bleaching followed first-order kinetics. The bleaching coefficient was 2.27 ± 0.08 × 10−2 cm2 J−1.
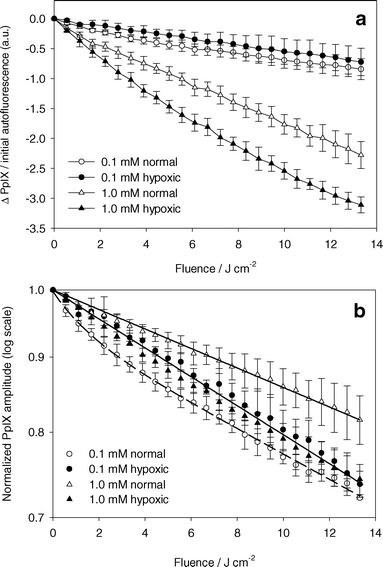 |
| Fig. 4 PpIX photobleaching. (a) Absolute change in PpIX basis spectrum amplitude normalized by the cell autofluorescence basis spectrum before PDT versus light fluence. ALA incubation concentration and oxygenation are indicated in the legend. PDT done with 532 nm light. (b) PpIX basis spectrum amplitude normalized to the initial PpIX basis spectrum amplitude versus light fluence. Solid lines are a single exponential decay curve fit to the data (closed symbols and open triangles). Dashed line is a double exponential decay curve fit to the data (open circles). Error bars represent ±1 standard deviation from a minimum of 3 independent experiments. | |
Photoproduct formation
Fig. 5a shows the change in Ppp amplitude normalized by the initial cell autofluorescence amplitude versus light fluence. For both ALA incubation concentrations, significantly higher levels of Ppp were measured during experiments performed under hypoxic conditions than under well oxygenated conditions.
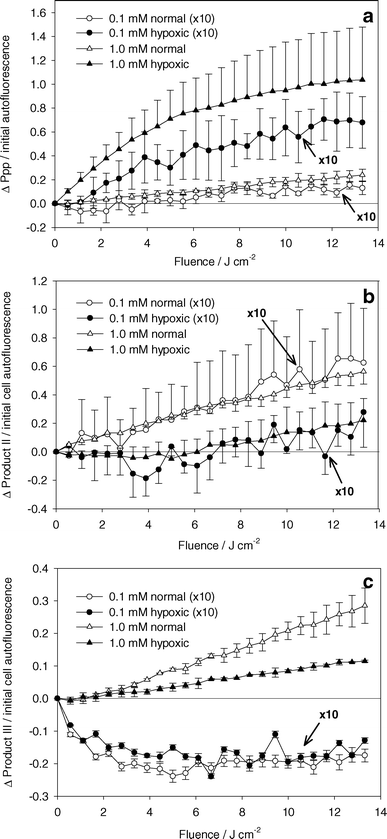 |
| Fig. 5 Absolute change in (a) Ppp, (b) product II, and (c) product III basis spectra amplitude normalized to initial cell autofluorescence basis spectrum amplitude. ALA incubation concentration and oxygenation are indicated in the legend. PDT done with 532 nm light. Data represented by circles (ALA incubation concentration of 0.1 mM) are scaled by a factor of 10. Error bars represent ±1 standard deviation from a minimum of 3 independent experiments. | |
The changes in product II and product III amplitudes normalized to initial cell autofluorescence are shown in Fig. 5b and 5c. Fig. 5b shows higher levels of product II generated under well oxygenated than under hypoxic conditions. The ratio of product II generated under well oxygenated versus hypoxic conditions is the same for both incubation concentrations. Under hypoxia, an increase in product II formation is seen beyond 6 J cm−2. There was a correlation between lack of Ppp fluorescence and increase in product II fluorescence. Although there is significant variability in the data in Fig. 5a and 5b, we observed that experiments with the highest accumulation of Ppp corresponded to those with the lowest accumulation of product II. Fig. 5c shows an increase in product III amplitude for experiments at high PpIX concentrations. At low PpIX concentrations, product III amplitude decreases initially, then shows no change.
Cell survival
Fig. 6 shows surviving cell fraction versus light fluence for ALA incubation concentrations of 0.1 and 1.0 mM for PDT under well oxygenated (open symbols) and hypoxic (closed symbols) conditions. For experiments under well oxygenated conditions, there was increased cell kill at higher incubation concentrations. When the sample was hypoxic, a small but significant decrease in viability was observed at both concentrations. It is unclear whether this cell killing was a result of residual oxygen in the sample enabling the generation of small amounts of singlet oxygen, or other oxygen independent reactions which lead to cell damage.
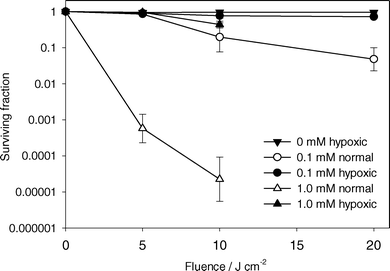 |
| Fig. 6 Surviving fraction of MLL cells determined by colony formation assay versus light fluence. ALA incubation concentration and initial oxygenation status are indicated in the legend. Cells were treated with 532 nm light. Error bars represent ±1 standard deviation from a minimum of 3 independent experiments. | |
Discussion
PpIX photobleaching
PpIX photobleaching under well oxygenated conditions differed from our observations of photobleaching of mTHPC and Photofrin in MLL cells. For these photosensitizers, the photobleaching kinetics could be characterized by a combined first and second order singlet oxygen mediated bleaching model, where a higher rate of photobleaching was found with increasing concentrations of photosensitizer.12,13 For PpIX, there is no simple explanation of the lower rate of bleaching at higher PpIX concentrations as seen in Fig. 4b. No significant oxygen depletion was observed during PDT, even at the higher PpIX concentration. A possible factor is the existence of multiple binding sites for PpIX within the cell.15 Our results are consistent with the idea that the preferred binding sites are readily available at low PpIX concentrations, whereas at high concentrations, they are saturated and the sensitizer localizes in secondary site(s).16 The photophysical properties of porphyrin photosensitizers, like PpIX, have been shown to depend strongly on local environment.17 Therefore, if the secondary binding site provides an environment which either protects PpIX from singlet oxygen damage or renders the sensitizer less photoactive, the result will be less relative bleaching at higher sensitizer concentrations. Photosensitizer aggregation may also be an important factor in the observed kinetics. Porphyrin aggregation can depend on sensitizer concentration and local environment and has been shown to affect photosensitizer fluorescence18 and photobleaching kinetics.19
For experiments done under well oxygenated conditions and high PpIX concentrations (open triangles in Fig. 4b), a single exponential can be fit to the data with a bleaching constant of 0.015 cm2 J−1. For low PpIX concentrations, the data can be fit by a double exponential with decay constants of 0.67 and 0.020 cm2 J−1 with relative weightings of 0.05 and 0.95 respectively. Other groups have shown that PpIX bleaching fits reasonably well to a double exponential decay curve in vitro.16,20,21 This suggests two first-order photobleaching processes, consistent with PpIX contained in two compartments. Since the fits at both PpIX concentrations have a component which decays with a similar rate constant, and the lower concentration has a component which decays at a higher rate, the data support the theory of multiple PpIX binding sites. The rapidly bleached component observed at low PpIX concentrations may be present at high PpIX concentrations, but its contribution to the total fluorescence would be too small to detect. We cannot explicitly state the fraction of sensitizer contained in each site from fluorescence measurements since it is possible that the fluorescence quantum yield may differ depending on the site of localization. This may also contribute to the observation that the ratio of PpIX fluorescence for 1.0 mM ALA incubation is only 3.8 times that for 0.1 mM ALA instead of 10 times (Fig. 3).
Our observation of reduced rate of photobleaching at higher PpIX concentration is consistent with the work of Bagdonas et al.22 for the treatment of WiDr cells in vitro with various concentrations of ALA and 635 nm light. A similar observation can be made in vivo, where Sorensen et al.23 showed reduced PpIX bleaching rate with increasing length of exposure of normal skin of bald/c nude mice to topical ALA. Juzenas et al.24 found a higher rate of PpIX bleaching in normal tissue compared to UV-induced tumors containing higher initial levels of PpIX during the treatment of hairless mice. Although it is difficult to compare our observations to those in vivo, it is clear that PpIX photobleaching in vivo and in vitro does not follow simple first- or second-order photobleaching kinetics. Multiple binding sites with distinct photochemistry is a possible explanation for the observed kinetics.
Under hypoxic conditions, normalized PpIX photobleaching was not dependent on concentration and the decrease could be fit by a single exponential decay (rate constant 0.023 cm2 J−1), suggesting a first-order photobleaching mechanism. This is qualitatively similar to the photobleaching kinetics we observed during the treatment of MLL cells with Photofrin under hypoxic conditions.13 In that study the oxygen independent bleaching mechanism was attributed to interactions between excited triplet-state PS and biological substrates. With PpIX, oxygen independent bleaching may occur through a similar mechanism. Cellular localization appears to be an important factor for oxygen independent bleaching, as evidenced by the lack of bleaching observed for PpIX in solution in the absence of oxygen or in the presence of sodium azide, a known singlet oxygen quencher.25,26 PpIX has been reported to photobleach during nitrogen flushing in vitro.22
At high PpIX concentrations we found the rate of bleaching was greater under hypoxic than under well oxygenated conditions. Comparing this result with available studies is difficult since few studies measure oxygen directly, but our results are consistent with those of Dietel et al.,27 who found increased PpIX bleaching in solid Ehrlich ascites carcinoma in vivo after occlusion of tumor vessels. Several studies have examined PpIX bleaching in vivo at increasing fluence rates, and attributed differences in bleaching to depletion of tissue oxygenation. Sorensen et al.23 found similar bleaching at low and high fluence rates, and Finlay et al.28 and Robinson et al.29 found less (although significant) bleaching at higher fluence rates. The factors contributing to the differences in the results of these studies are not apparent, but our results indicate that sensitizer concentration is an important variable. Since the bleaching kinetics are concentration dependent, it is difficult to compare the results of these studies without quantitative PpIX concentration information.
Photoprotoporphyrin (Ppp) production
There is an abundance of evidence to support the idea that Ppp is a product of the interaction between singlet oxygen and PpIX. This reaction may occur from the cycloaddition of singlet oxygen to one or both the vinyl groups of PpIX, reducing the adjacent double bonds.16,30,31 Increased bleaching of PpIX and initial rate of Ppp formation are seen when PDT is performed in the presence of D2O, demonstrating enhanced singlet oxygen mediated Ppp formation.16,26 This is clearly not the only mechanism of Ppp production, since large amounts of Ppp are produced under hypoxic conditions (Fig. 5a), for which the cell survival data (Fig. 6) suggest that very little singlet oxygen (or other reactive oxygen species) was produced. It is difficult to conclude that more Ppp is produced under hypoxic conditions, as suggested by Fig. 5a, since Ppp is susceptible to photobleaching.32 In fact, the photobleaching quantum yield of Ppp has been shown to be comparable to that of PpIX.33 Ppp bleaching is enhanced in the presence of D2O, showing that this reaction is at least partly mediated by singlet oxygen.16 We cannot say that Ppp photobleaching is exclusively a result of singlet oxygen interactions, but the build-up of Ppp under hypoxia (Fig. 5a) suggests that Ppp bleaching is inhibited under these conditions. Under well oxygenated conditions, we did not observe a decrease in Ppp fluorescence at extended light fluences as reported by other groups, but those studies were done for significantly higher light fluences.16,22,32 The increase in Ppp at low fluences (below 14 J cm−2) is consistent with our results.
Product II
The generation of product II has been identified by other groups. Formation of product II has been demonstrated in cells treated with Ppp and 670 nm light,33 and in the selective bleaching of Ppp using 670 nm light following treatment using ALA induced PpIX and 635 nm light in vitro.22 These findings imply that product II is a photoproduct of Ppp, and not of PpIX. Finlay et al.28 found diminished production of product II in vivo at high compared to low fluence rates. This observation was attributed to oxygenation depletion at high fluence rates, and is consistent with our findings in Fig. 5b, where we found very little product II under hypoxic conditions. Our data do not support the idea that product II is generated exclusively from reactions between singlet oxygen and Ppp, since small, but significant, amounts were generated under hypoxic conditions when the fluence exceeded 6 J cm−2.
Product III
Product III is likely a contribution from uroporphyrin (Up) and/or coproporphyrin (Cp). These porphyrins are produced in the intermediate steps of the biosynthetic heme pathway. Dietel et al.8 attributed a similar fluorescence peak in vitro to Up and Cp with fluorescence peaks at 618 and 613 nm respectively. This is consistent with the results of Finlay et al.28 and Juzenas et al.34 The spectra included a secondary peak centered at ∼678 nm that we did not observe. This wavelength corresponds to the peak of Ppp and this feature may not have been discernible with our method of acquiring the product III basis spectrum. The data in Fig. 5c show a large difference in product III accumulation at the two concentrations. At 0.1 mM ALA incubation concentration, we found a decrease in the amplitude of the fit basis spectrum with increasing light dose up to 4 J cm−2, independent of oxygenation. The initial amplitude of the product III basis spectrum divided by the amplitude of the cell autofluorescence basis spectra was 0.08 ± 0.04 at the low ALA incubation concentration. Therefore, the reduction in product III at this concentration represents a decrease of about 25%. This is in contrast to the results at high incubation concentrations, where product III amplitude increased with increasing light dose. In this case the initial product III amplitude divided by the autofluorescence amplitude was 0.23 ± 0.09, and the amplitude increased by ∼10% and ∼4% per J cm−2 under well oxygenated and hypoxic conditions respectively. The differences in these results can be explained by considering the mechanisms of Up/Cp generation and loss. Clearly, the increase in product III seen during the experiment under hypoxic conditions at 1.0 mM ALA concentration is a result of continual generation of Up/Cp from high concentrations of ALA in the cell. The additional increase in product III in the presence of oxygen could be a result of two processes, either light induced changes in the enzymatic pathway leading to enhanced Up/Cp production,8 or damage to the mitochondria causing an arrest in the synthesis of PpIX and other mitochondrial porphyrins.27 The decrease in Up/Cp observed at low ALA incubation concentrations is likely a result of an initially higher than metabolic concentration of Up/Cp. Once ALA is removed from the medium, the residual ALA is quickly metabolized to Up/Cp and ultimately to PpIX. Therefore, initially high levels of Up/Cp will drop to metabolic levels at a rate determined by the enzymes that convert Up-III to Cp-III to PpIX. As well, there is evidence demonstrating that porphobilinogen deaminase, an enzyme required to produce Up/Cp, can be inhibited by ALA PDT,35 and that Up/Cp are also photosensitizers and are susceptible to photobleaching. We cannot attribute our observed decreases in product III to these mechanisms since we found no differences between experiments under hypoxic and well oxygenated conditions.
PpIX photobleaching, photoproduct formation and singlet oxygen dose estimation
Unlike mTHPC and Photofrin, it appears that PpIX fluorescence photobleaching cannot be used as the basis for a singlet oxygen dose metric for several reasons. (1) Relating the photobleaching kinetics to singlet oxygen production is difficult because of the apparent concentration dependence of PpIX binding, and therefore concentration dependent photobleaching kinetics. (2) The photoproducts (especially Ppp) are photosensitizers and contribute to the photodynamic dose. (3) Singlet oxygen generated by the photoproducts may contribute to the bleaching of PpIX. (4) PpIX photobleaches in the absence of oxygen. In order to determine the singlet oxygen mediated bleaching, the oxygen independent bleaching must be characterized and accounted for, therefore requiring a measurement of oxygenation during treatment. (5) PpIX can re-localize during treatment, affecting the biological targets, sensitizer kinetics, and fluorescence quantum yield.36 (6) PpIX may be continually generated during treatment in vivo, skewing the measured PpIX concentration and photobleaching kinetics.
The photoproducts of PpIX may be more useful as potential singlet oxygen dose metrics, as observed with other porphyrin photosensitizers.37 Ppp is the main photoproduct of PpIX and there is evidence that, under well oxygenated conditions, this product is a result of reactions with singlet oxygen. Unfortunately, Ppp has two limitations as a singlet oxygen reporter: it accumulates in greater quantities in the absence of oxygen, and it can undergo singlet oxygen mediated photobleaching at a relatively high rate. As a result, it is difficult to determine the absolute amount of Ppp generated exclusively from singlet oxygen reactions.
Product II on the other hand appears to correlate well to cell survival, as seen in Fig. 7. The relationship between cell survival and change in product II fluorescence was independent of ALA incubation concentration and medium oxygenation. The only exception was the experiment done under hypoxic conditions and high ALA concentrations. For these treatments, the increase in product II fluorescence beyond 6 J cm−2 did not correspond to a decrease in cell survival (Fig. 5b). It is unclear what the source of this discrepancy might be, but the apparent non-singlet oxygen mediated increase in product II fluorescence under this condition may limit the usefulness of this dose metric. This product was not observed in vivo at high fluence rates (i.e. presumably reduced oxygenation) at fluences up to 20 J cm−2.28
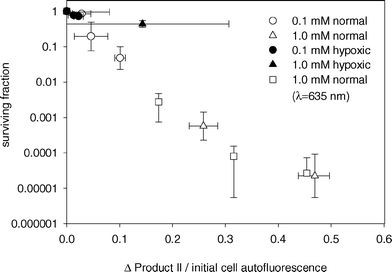 |
| Fig. 7 MLL cell surviving fraction versus change in product II amplitude divided by initial autofluorescence amplitude. Cell survival was determined by colony formation assay. ALA incubation concentration and media oxygenation are given in the legend. Cells were treated with either 532 nm (circles, triangles) or 635 nm light (squares). | |
Below 6 J cm−2, changes in product II fluorescence are consistent with our observations that product II is primarily a result of singlet oxygen mediated reactions. If this is the case, product II should correlate to singlet oxygen production, independent of treatment wavelength and PpIX bleaching kinetics. To confirm this relationship, cells were treated with 635 nm light, a wavelength typically used for ALA PDT. Fig. 8a shows PpIX photobleaching and Ppp accumulation during PDT of MLL cells incubated in 1.0 mM ALA. PpIX bleaching was consistent with our results shown in Fig. 4b, where a higher rate of bleaching was seen for the treatment under hypoxic conditions. The overall bleaching was greater than the corresponding experiments in Fig. 4b, but this difference may be explained by differences in the absorption of PpIX at 635 nm compared to 532 nm. Fig. 8a also shows corresponding Ppp formation during these experiments. The differences in these results from those at 532 nm (Fig. 5a) may be explained by higher Ppp production as a result of increased PpIX bleaching, as well as differences in absorption of Ppp at 532 nm compared to 635 nm. Despite the differences in PpIX and Ppp kinetics at 635 nm versus 532 nm, the relationship between change in product II fluorescence (Fig. 8b) and cell survival was consistent with the treatments done at 532 nm (Fig. 7). This suggests that product II is directly related to singlet oxygen dose, at least for fluences below 6 J cm−2. Beyond 6 J cm−2, the treatment under hypoxic conditions showed an increase in product II fluorescence that could not be related to singlet oxygen production, consistent with the results shown in Fig. 5b. It is unclear whether the change in product II fluorescence is a result of changes in product II fluorescence quantum yield, or increased production resulting from non-singlet oxygen mediated reactions.
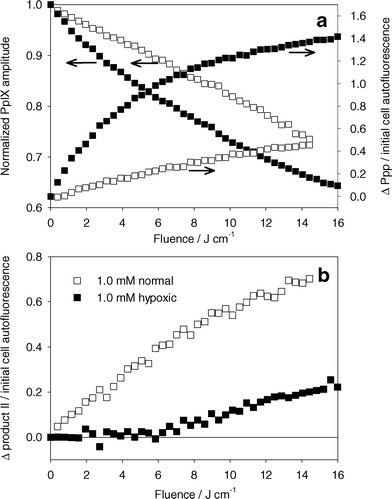 |
| Fig. 8 (a) Left axis: normalized PpIX photobleaching versus light dose for MLL cells incubated in 1.0 mM ALA for 4 h and treated with 635 nm light. Right axis: change in Ppp basis spectrum amplitude normalized to cell autofluorescence amplitude. (b) Change in product II basis spectrum amplitude normalized to initial cell autofluorescence basis spectra amplitude. PDT was done under well oxygenated (open symbols) or hypoxic (closed symbols) conditions. | |
In conclusion, this is the first study to investigate explicitly the dependence of PpIX photobleaching and photoproduct formation on oxygen and sensitizer concentration during PDT in vitro. We used cell survival as a biological end point to assess potential fluorescence-based singlet oxygen dose metrics. Fluorescence photobleaching of ALA induced PpIX exhibited complex photobleaching kinetics in vitro, likely resulting from differential binding of the sensitizer within the cell. PpIX bleaching occurred in the absence of oxygen, making quantification of singlet oxygen dose based on a direct measure of PpIX difficult. The main photoproduct of PpIX photobleaching was Ppp, and it was produced under both hypoxic and well oxygenated conditions. Ppp accumulation did not correlate to singlet oxygen production, since Ppp undergoes bleaching. A second photoproduct with a fluorescence peak at ∼655 nm correlated well to singlet oxygen production for most treatment conditions. The increase in this product seen beyond 6 J cm−2 under hypoxic conditions did not correlate to changes in cell survival, although the significance of this increase is not known in vivo, and it therefore requires further investigation.
Acknowledgements
This work has been supported by National Institutes of Health grant P01-CA43892. The authors wish to thank Dr Gurmit Singh and Dr Andrew Rainbow for helpful discussions.
References
- H. Fukuda, A. Casas and A. Batlle, Aminolevulinic acid: from its unique biological function to its star role in photodynamic therapy, Int. J. Biochem. Cell Biol., 2005, 37, 272–276 Search PubMed.
- B. Krammer and K. Uberriegler,
In-vitro investigation of ALA-induced protoporphyrin IX, J. Photochem. Photobiol., B, 1996, 36, 121–126 CrossRef CAS.
- J. C. Kennedy, R. H. Pottier and D. C. Pross, Photodynamic therapy with endogenous protoporphyrin IX: basic principles and present clinical experience, J. Photochem. Photobiol., B, 1990, 6, 143–148 CrossRef CAS.
- L. Wyld, M. W. Reed and N. J. Brown, The influence of hypoxia and pH on aminolaevulinic acid-induced photodynamic therapy in bladder cancer cells in vitro, Br. J. Cancer, 1998, 77, 1621–1627 CAS.
- P. Uehlinger, M. Zellweger, G. Wagnieres, L. Juillerat-Jeanneret, H. van den Bergh and N. Lange, 5-Aminolevulinic acid and its derivatives: physical chemical properties and protoporphyrin IX formation in cultured cells, J. Photochem. Photobiol., B, 2000, 54, 72–80 CrossRef CAS.
- I. Georgakoudi, P. C. Keng and T. H. Foster, Hypoxia significantly reduces aminolaevulinic acid-induced protoporphyrin IX synthesis in EMT6 cells, Br. J. Cancer, 1999, 79, 1372–7 CrossRef CAS.
- A. Juzeniene, P. Juzenas, O. Kaalhus, V. Iani and J. Moan, Temperature effect on accumulation of protoporphyrin IX after topical application of 5-aminolevulinic acid and its methylester and hexylester derivatives in normal mouse skin, Photochem. Photobiol., 2002, 76, 452–456 CrossRef CAS.
- W. Dietel, K. Bolsen, E. Dickson, C. Fritsch, R. Pottier and R. Wendenburg, Formation of water-soluble porphyrins and protoporphyrin IX in 5-aminolevulinic-acid-incubated carcinoma cells, J. Photochem. Photobiol., B, 1996, 33, 225–231 CrossRef CAS.
- L. Wyld, J. L. Burn, M. W. Reed and N. J. Brown, Factors affecting aminolaevulinic acid-induced generation of protoporphyrin IX, Br. J. Cancer, 1997, 76, 705–712 CAS.
- I. A. Boere, D. J. Robinson, H. S. de Bruijn, J. van den Boogert, H. W. Tilanus, H. J. Sterenborg and R. W. de Bruin, Monitoring in situ dosimetry and protoporphyrin IX fluorescence photobleaching in the normal rat esophagus during 5-aminolevulinic acid photodynamic therapy, Photochem. Photobiol., 2003, 78, 271–277 CrossRef CAS.
- M. J. Niedre, A. J. Secord, M. S. Patterson and B. C. Wilson,
In vitro tests of the validity of singlet oxygen luminescence measurements as a dose metric in photodynamic therapy, Cancer Res., 2003, 63, 7986–7994 CAS.
- J. S. Dysart, G. Singh and M. S. Patterson, Calculation of Singlet Oxygen Dose from Photosensitizer Fluorescence and Photobleaching During mTHPC Photodynamic Therapy of MLL Cells, Photochem. Photobiol., 2005, 81, 196–205 CrossRef CAS.
- J. S. Dysart and M. S. Patterson, Characterization of Photofrin photobleaching for singlet oxygen dose estimation during photodynamic therapy of MLL cells in vitro, Phys. Med. Biol., 2005, 50, 2597–2616 CrossRef CAS.
- J. S. Dysart, M. S. Patterson, T. J. Farrell and G. Singh, Relationship between mTHPC fluorescence photobleaching and cell viability during in vitro photodynamic treatment of DP16 cells, Photochem. Photobiol., 2002, 75, 289–295 CAS.
- L. Brancaleon and H. Moseley, Effects of photoproducts on the binding properties of protoporphyrin IX to proteins, Biophys. Chem., 2002, 96, 77–87 CrossRef CAS.
- J. Moan, G. Streckyte, S. Bagdonas, O. Bech and K. Berg, Photobleaching of protoporphyrin IX in cells incubated with 5-aminolevulinic acid, Int. J. Cancer, 1997, 70, 90–97 CrossRef CAS.
- L. Brancaleon, S. W. Magennis, I. D. Samuel, E. Namdas, A. Lesar and H. Moseley, Characterization of the photoproducts of protoporphyrin IX bound to human serum albumin and immunoglobulin G, Biophys. Chem., 2004, 109, 351–360 CrossRef CAS.
- A. Datta, A. Dube, B. Jain, A. Tiwari and P.
K. Gupta, The effect of pH and surfactant on the aggregation behavior of chlorin p6: a fluorescence spectroscopic study, Photochem. Photobiol., 2002, 75, 488–494 CAS.
- L. Bezdetnaya, N. Zeghari, I. Belitchenko, M. Barberi-Heyob, J. L. Merlin, A. Potapenko and F. Guillemin, Spectroscopic and biological testing of photobleaching of porphyrins in solutions, Photochem. Photobiol., 1996, 64, 382–386 CrossRef CAS.
- C. D. Sudworth, M. R. Stringer, J. E. Cruse-Sawyer and S. B. Brown, Fluorescence microspectroscopy technique for the study of intracellular protoporphyrin IX dynamics, Appl. Spectrosc., 2003, 57, 682–688 CrossRef CAS.
- W. S. Strauss, R. Sailer, M. H. Gschwend, H. Emmert, R. Steiner and H. Schneckenburger, Selective examination of plasma membrane-associated photosensitizers using total internal reflection fluorescence spectroscopy: correlation between photobleaching and photodynamic efficacy of protoporphyrin IX, Photochem. Photobiol., 1998, 67, 363–369 CAS.
- S. Bagdonas, L. W. Ma, V. Iani, R. Rotomskis, P. Juzenas and J. Moan, Phototransformations of 5-aminolevulinic acid-induced protoporphyrin IX in vitro: a spectroscopic study, Photochem. Photobiol., 2000, 72, 186–192 CAS.
- R. Sorensen, V. Iani and J. Moan, Kinetics of photobleaching of protoporphyrin IX in the skin of nude mice exposed to different fluence rates of red light, Photochem. Photobiol., 1998, 68, 835–840 CrossRef CAS.
- P. Juzenas, S. Sharfaei, J. Moan and R. Bissonnette, Protoporphyrin IX fluorescence kinetics in UV-induced tumours and normal skin of hairless mice after topical application of 5-aminolevulinic acid methyl ester, J. Photochem. Photobiol., B, 2002, 67, 11–17 CrossRef CAS.
- M. B. Ericson, S. Grapengiesser, F. Gudmundson, A. M. Wennberg, O. Larko, J. Moan and A. Rosen, A spectroscopic study of the photobleaching of protoporphyrin IX in solution, Lasers Med. Sci., 2004, 18, 56–62 Search PubMed.
- K. Konig, H. Schneckenburger, A. Ruck and R. Konig, Studies on porphyrin photoproducts in solution, cells, and tumor tissue, Proc. SPIE-Int. Soc. Opt. Eng., 1994, 2133, 226–237.
- W. Dietel, C. Fritsch, R. H. Pottier and R. Wendenburg, 5-Aminolaevulinic-acid-induced formation of different porphyrins and their photomodifications, Lasers Med. Sci., 1997, 12, 266–236.
- J. C. Finlay, D. L. Conover, E. L. Hull and T. H. Foster, Porphyrin bleaching and PDT-induced spectral changes are irradiance dependent in ALA-sensitized normal rat skin in vivo, Photochem. Photobiol., 2001, 73, 54–63 CAS.
- D. J. Robinson, H. S. de Bruijn, N. van der Veen, M. R. Stringer, S. B. Brown and W. M. Star, Protoporphyrin IX fluorescence photobleaching during ALA-mediated photodynamic therapy of UVB-induced tumors in hairless mouse skin, Photochem. Photobiol., 1999, 69, 61–70 CrossRef CAS.
- G. S. Cox, C. Bobillier and D. G. Whitten, Photooxidation and singlet oxygen sensitization by protoporphyrin IX and its photooxidation products, Photochem. Photobiol., 1982, 36, 401–407 CrossRef CAS.
- D. E. Gudgin and R. H. Pottier, On the role of protoporphyrin IX photoproducts in photodynamic therapy, J. Photochem. Photobiol., B, 1995, 29, 91–93 CrossRef.
- L. Ma, S. Bagdonas and J. Moan, The photosensitizing effect of the photoproduct of protoporphyrin IX, J. Photochem. Photobiol., B, 2001, 60, 108–113 CrossRef CAS.
- T. Theodossiou and A. J. MacRobert, Comparison of the photodynamic effect of exogenous photoprotoporphyrin and protoporphyrin IX on PAM 212 murine keratinocytes, Photochem. Photobiol., 2002, 76, 530–537 CrossRef CAS.
- P. Juzenas, V. Iani, S. Bagdonas, R. Rotomskis and J. Moan, Fluorescence spectroscopy of normal mouse skin exposed to 5-aminolaevulinic acid and red light, J. Photochem. Photobiol., B, 2001, 61, 78–86 CrossRef CAS.
- S. L. Gibson, J. J. Havens, M. L. Nguyen and R. Hilf, Delta-aminolaevulinic acid-induced photodynamic therapy inhibits protoporphyrin IX biosynthesis and reduces subsequent treatment efficacy in vitro, Br. J. Cancer, 1999, 80, 998–1004 CrossRef CAS.
- D. Kessel, Relocalization of cationic porphyrins during photodynamic therapy, Photochem. Photobiol. Sci., 2002, 1, 837–840 RSC.
- H. Zeng, M. Korbelik, D. I. McLean, C. MacAulay and H. Lui, Monitoring photoproduct formation and photobleaching by fluorescence spectroscopy has the potential to improve PDT dosimetry with a verteporfin-like photosensitizer, Photochem. Photobiol., 2002, 75, 398–405 CAS.
|
This journal is © The Royal Society of Chemistry and Owner Societies 2006 |
Click here to see how this site uses Cookies. View our privacy policy here.