A novel 10B-enriched carboranyl-containing phthalocyanine as a radio- and photo-sensitising agent for boron neutron capture therapy and photodynamic therapy of tumours: in vitro and in vivo studies
Received
6th May 2005
, Accepted 22nd September 2005
First published on 19th October 2005
Abstract
The synthesis of a Zn(II)–phthalocyanine derivative bearing four 10B-enriched o-carboranyl units (10B-ZnB4Pc) and its natural isotopic abundance analogue (ZnB4Pc) in the peripheral positions of the tetraazaisoindole macrocycle is presented. The photophysical properties of ZnB4Pc, as tested against model biological systems, were found to be similar with those typical of other photodynamically active porphyrin-type photosensitisers, including a singlet oxygen quantum yield of 0.67. The carboranyl-carrying phthalocyanine was efficiently accumulated by B16F1 melanotic melanoma cells in vitro, appeared to be partitioned in at least some subcellular organelles and, upon red light irradiation, induced extensive cell mortality. Moreover, ZnB4Pc, once i.v.-injected to C57BL/6 mice bearing a subcutaneously transplanted pigmented melanoma, photosensitised an important tumour response, provided that the irradiation at 600–700 nm was performed 3 h after the phthalocyanine administration, when appreciable concentrations of ZnB4Pc were still present in the serum. Analogously, irradiation of the 10B-ZnB4Pc-loaded pigmented melanoma with thermal neutrons 24 h after injection led to a 4 day delay in tumour growth as compared with control untreated mice. These results open the possibility to use one chemical compound as both a photosensitising and a radiosensitising agent for the treatment of tumours by the combined application of photodynamic therapy and boron neutron capture therapy.
Introduction
Boron neutron capture therapy (BNCT) and photodynamic therapy (PDT) represent two emerging therapeutic modalities for tumour treatment1,2 which are currently under active investigation in view of palliative or curative applications. Both modalities involve the systemic injection of an intrinsically non-toxic radio- or photo-sensitising agent to the patient, followed by the specific irradiation of the diseased area with thermal neutrons in the case of BNCT3 or selected visible light wavelengths for PDT.4 In BNCT the cytotoxic effects are promoted by 4He2+ and 7Li3+ particles, that are produced by fission of the 11B isotope consequent to the (10B + n) reaction,1,3 whereas PDT acts via oxidative attack on cell constituents largely by singlet oxygen generated through energy transfer from the electronically excited photosensitizer to ground state molecular oxygen.5
At present, regulatory approval is limited to borono-phenylalanine and sodium borocaptate6 for BNCT, as well as to Photofrin, a complex mixture of haematoporphyrin derivatives,7 for PDT. A large number of patients have been treated by either technique worldwide with objectively positive results. However, for both techniques, severe limitations exist owing to the relatively low selectivity of tumour targeting by the above mentioned radio- or photo-sensitising agents coupled with the small efficiency of their interaction with the incident radiation.3,4 Recently, new perspectives have been opened in this field by the synthesis of chemically pure boron-labelled porphyrins8 or phthalocyanines,9 where the tetrapyrrolic derivative can act as a vehicle for the transport of significant amounts of boron to the neoplastic lesion; at the same time, many newly developed porphyrins and phthalocyanines have been shown to possess tumour-photosensitising properties which are markedly superior to those typical of Photofrin.10 In principle, such boron-loaded porphyrins/phthalocyanines could allow the development of combination therapies based on the sequential application of BNCT and PDT, given the different mechanisms of action of the two therapeutic approaches. Preliminary investigations from our laboratory11 pointed out that the attachment of one carborane moiety at the periphery of the phthalocyanine tetraazaisoindole macrocycle does not impair the photophysical and photosensitising properties of the phthalocyanine in model biological systems.
In this paper, the synthesis of a 10B-enriched tetra-carboranylmethylphenoxy-substituted Zn(II)–phthalocyanine is reported for the first time (see patent WO 2004/081014A1). The description of the properties of such phthalocyanine derivative carrying 40 boron atoms per molecule, which should thus exhibit a greater cross section for interaction with neutrons, represents an extension of our previous studies. Our investigations were also aimed at a more detailed characterization of the photosensitization pathways involved in cell inactivation by the boron-labelled Zn(II)–phthalocyanine, as well as at an exploratory test of its PDT and BNCT activity in mice bearing a tumour which is known to be poorly responsive to conventional PDT, such as malignant melanotic melanoma.
Materials and methods
Chemicals
All the starting materials and solvents used (Merck and Sigma-Aldrich) were high grade commercial products, and were used as supplied or purified according to literature procedures;1211B-1,2-closo-carborane was supplied by Katchem (Prague, Czech Republic), while 10B-1,2-closo-carborane was kindly supplied by Dr Karel Base (Duke University Medical Centre, Durham, North Carolina); both compounds were used without further purification. Natural isotope abundance 3-{4-[(o-carboran-1-yl)methyl]phenoxy}phthalonitrile 2 and 1,8(11),15(18),22(25)-tetrakis-{4-[(o-carboran-1-yl)methyl]phenoxy}-phthalocyaninate zinc(II)
4 were synthesized as described elsewhere.13 Deuterated solvents were obtained from Spectra 2000. Foetal calf serum (FCS) was a product of Gibco Invitrogen. The Dulbecco's modified Eagle medium (DMEM) used for cell growth and trypan blue were supplied by Sigma. Sodium dodecylsulfate (SDS) was obtained from Merck. N-acetyl-L-tryptophanamide (NATA) and 9, 10-dimethylanthracene (DMA) were obtained from Sigma (St. Louis, Missouri).
Analytical procedures
Melting points (uncorrected) were determined using an Electrothermal Digital Mel-Temp 3.0 melting point apparatus. UV-visible spectra were obtained using a HP8453 spectrophotometer. 1H and 13C NMR spectra were recorded on a Varian VXR-300 S instrument. Chemical shifts were expressed in parts per million (δ), using the 2.47 ppm line (d6-DMSO) for 1H NMR spectra and the 40.17 line (d6-DMSO) for 13C NMR spectra as the references. ESI-MS spectra were recorded on a API 365 PESCIEX (5 µL min−1) spectrometer and FAB-MS spectra on a VG70 250 S. For flash chromatography and MPLC Merck silica gel 60 (40–63 µm) was used.
Synthesis of 3-{4-[(10B-o-carboran-1-yl)methyl]phenoxy}phthalonitrile (3).
n-Butyl lithium (1.40 ml, 1.6 M in hexane, 2.2 mmol) was added dropwise to a solution of 10B-1,2-closo-carborane (235 mg, 1.7 mmol) in anhydrous tetrahydrofuran (10 ml) kept in an inert atmosphere at −78 °C. The solution was stirred at −78 °C for 10 min, then it was kept at room temperature for 40 min and cooled again at −78 °C, and 3-[4-(bromomethyl)phenoxy]phthalonitrile (500 mg, 1.6 mmol)
(1)13 was added. The mixture was stirred for 1 h while being warmed to room temperature, then it was quenched with water, and the mixture was extracted with ethyl acetate. The organic layer was washed with brine (30 ml × 2), then it was dried on anhydrous Na2SO4, and the solvent was evaporated. The crude product was purified by flash chromatography (eluent: petroleum spirit/ethyl acetate = 3/1, v/v) to yield 300 mg (47%) of the title compound. 1H NMR (300 MHz, CDCl3): 7.63 (1H, dd, J1
=
J2
= 7.8 Hz), 7.51 (1H, d, J
= 7.8 Hz), 7.26–7.22 (2H, m), 7.14–7.08 (3H, m), 3.54 (2H, s), 3.38 (1H, bs), 2.50–1.82 (10H, bm)
δ; 13C NMR (75 MHz, CDCl3): 160.38, 154.37, 134.84, 132.54, 132.24, 127.84, 121.33, 120.84, 117.75, 115.22, 112.71, 106.92, 74.32, 59.98, 43.11 δ; ESI−-MS: m/z 367 [C17H20N2OB10]−. Melting point: 179–181 °C.
Synthesis of 1,8(11),15(18),22(25)-tetrakis-{[4-(10B-o-carboran-1-yl)methyl]phenoxy}-phthalocyaninate zinc(II).
A mixture of 3-{4-[(10B-o-carboran-1-yl)methyl]phenoxy}phthalonitrile 3
(200 mg, 0.5 mmol) and Zn(II)–acetate (100 mg, 0.5 mmol) was finely ground and heated to 210 °C in an inert atmosphere for 4.5 h. The dark solid was then allowed to cool to room temperature, and was taken up in ethyl acetate. The suspension was filtered through Celite and the solvent was evaporated. From the crude mixture the title compound was isolated by flash chromatography (eluent: petroleum spirit/THF = 3/1 to 1/1); 83 mg (yield = 40%). 1H NMR (300 MHz, d6-DMSO): 9.13 (d, J
= 7.2 Hz), 9.01–8.98 (m), 8.68–8.56 (m), 8.75 (d, J
= 7.2 Hz), 8.65 (dd, J1
=
J2
= 7.2 Hz), 8.52 (d, J
= 7.2 Hz), 8.15–7.82 (m), 7.73 (d, J
= 7.5 Hz), 7.47–7.22 (m), 5.24–5.18 (m), 4.92 (bs), 3.68–3.64 (m), 3.50 (bs), 2.71–1.18 (bm)
δ; 13C NMR (75 MHz, d6-DMSO): 159.81, 159.51, 159.39, 157.46, 157.39, 157.08, 154.46, 154.09, 153.94, 153.55, 153.07, 152.81, 150.38, 141.46, 141.39, 136.86, 132.95, 132.29, 132.06, 131.86, 131.67, 131.49, 129.95, 129.11, 128.78, 127.62, 127.50, 127.27, 121.07, 120.68, 120.52, 120.19, 116.65, 116.50, 77.50, 77.38, 63.65, 63.14, 42.17, 41.93 δ; ESI+-MS: m/z 1540 [C68H80N8 O4B40Zn]+; UV-vis (DMF): 690 nm (100), 622 nm (16), 326 nm (17)
ε690 nm
= 250
000 M−1 cm−1.
Preparation of liposomes
The preparation was performed by using 1,2 dioleoyl-sn-glycero-3-phosphocholine (DOPC) provided as a 20 mg ml−1 chloroform solution by Avanti Polar Lipids, Inc. The handling of the lipid was always performed in a nitrogen atmosphere. Typically, a chloroform solution containing both the phospholipid and the boronated phthalocyanine in a 200 : 1 molar ratio was dried by rotary evaporation under reduced pressure at room temperature. The phospholipid–photosensitiser film was resuspended in phosphate-buffered saline (PBS), pH 7.4 (4 ml per 80 mg lipid). This ice-cooled solution was sonicated (RAPIDIS 300, Paul Funke and Co., Milan, Italy) for 30 min under a N2 gas flow. At the end the suspension was filtered using a disc-filter (0.2 µm) in order to isolate a uniform population of small unilamellar phospholipid vesicles.
Determination of the photosensitising efficiency
The photosensitising efficiency of ZnB4Pc was evaluated by following the photooxidative modification of a well known photosensitive amino acid derivative, i.e.N-acetyl-L-tryptophanamide (NATA).14 In a typical experiment, a 20 µM solution of NATA in N,N-dimethylformamide (DMF) was mixed (1 : 1, v/v) with a DMF solution of ZnB4Pc having an absorbance of 0.4 at the maximum peak and was irradiated at 25 °C in a 1 cm quartz cuvette under gentle magnetic stirring for different times with 600–700 nm light from a halogen lamp (Waldmann, Schweningen, Germany). The fluence-rate was 100 mW cm−2. The time-dependent decrease in the NATA concentration was followed by measuring the fluorescence emission in the 300–450 nm wavelength range upon excitation at 290 nm. Previous studies showed14 that under these experimental conditions there is a linear relationship between fluorescence intensity and NATA concentration. The first-order photooxidation rate constant (kv) was obtained by plotting lnF0/F
(F0 and F are the fluorescence intensity at time 0 and time t) as a function of the irradiation time. All fluorescence measurements were carried out by means of a Cary Eclipse Varian spectrophotofluorimeter.
Determination of singlet oxygen quantum yield
The quantum yield (ΦΔ) of singlet oxygen generation by ZnB4Pc was measured by following the decrease in the fluorescence emission of 9,10-dimethyl-anthracene (DMA) upon its photosensitised conversion into the corresponding non-fluorescent 9,10-endoperoxide.15 A 20 µM DMA and 1.7 µM ZnB4Pc solution in DMF were placed in a quartz cuvette with a 1 cm optical path and irradiated at 20 ± 2 °C under gentle magnetic stirring at 600–700 nm (Waldmann light source, 100 mW cm−2) for different periods of time. The DMA fluorescence emission was recorded in the 380–550 nm wavelength range with excitation at 360 nm. The first-order rate constant of the photoprocess was obtained by plotting lnF0/F as a function of the irradiation time. The rate constant was then converted into 1O2 quantum yield by comparison with the rate constant for DMA photooxidation sensitized by unsubstituted Zn(II)–phthalocyanine (ZnPc); the ΦΔ of this phthalocyanine was shown to be 0.55.16
Fluorescence quantum yield
The fluorescence quantum yield for ZnB4Pc was measured in a Perkin Elmer LS 50 spectrophotofluorimeter, using a ZnB4Pc solution in DMF and an ethanol solution of unsubstituted ZnPc, which acted as a reference standard having an absorbance of 0.05 at 680 nm. The fluorescence emission was recorded between 620 and 800 nm, exciting at 600 nm. The quantum yield was obtained by the following formula:
ΦS
=
ΦR(FSARη2)/(ASFRη02) |
where FS and FR are the areas of the emission spectra of the investigated substance and the reference, respectively; AS and AR are the absorbances at 600 nm; η and η0 represent the refractive indexes of the solvent; ΦR is fluorescence quantum yield of the reference (ZnPc in ethanol = 0.2).17
Photostability studies
The stability of the phthalocyanine to red light irradiation was determined by preparing a solution of ZnB4Pc in DMF with an absorbance of 0.2 at the maximum peak. This solution was placed in a quartz cuvette with a 1 cm optical path and irradiated at 25 °C for different periods of time under gentle magnetic stirring by using 600–700 nm light (Waldmann light source, 100 mW cm−2). After each irradiation, the absorbance spectrum was recorded by a Cary Eclipse 50 scan spectrophotometer and the value of the absorbance at the maximum peak at time 0 was compared with those recorded at the various irradiation times.
Cell accumulation and photosensitization studies
The cell line used was the melanotic murine melanoma B16F1. The cells were grown in Dulbecco's modified Eagle medium (DMEM) containing 10% foetal bovine serum (FBS). The culture medium was added with 100 units ml−1 of penicillin and 100 µg ml−1 of streptomycin, as well as with 2.5 µg ml−1 of amphotericin. The cells were then kept in a dark humid atmosphere containing 5% CO2
(95% humidity) at a constant temperature of 37 °C. The cells were seeded approximately 1 : 3 every two or three days. Typically, the culture medium was removed and the cells were washed twice with PBS without Ca2+ and Mg2+ ions, and incubated for 3 min with a 0.05% trypsin–0.02% EDTA solution. The action of trypsin was stopped by the addition of serum. The cells were finally resuspended in DMEM and seeded.
To investigate the phthalocyanine uptake, 3 × 105 cells were seeded in 25 cm2 flasks and approximately 24 h later the cells were washed twice with PBS. Then the cells were incubated with ZnB4Pc-containing liposomes. During incubation the cells were kept in a humid atmosphere containing 5% CO2
(95% humidity) at a constant temperature of 37 °C. At the end, the cells were washed twice with PBS without Ca2+ and Mg2+. Then, 1 ml of a 2% SDS solution per flask was added to the cells and incubated for 60 min under gentle magnetic stirring. An aliquot was mixed with a methanol–chloroform solution (2 : 1, v/v) and the fluorescence emission was measured. A second aliquot was used to measure the protein concentration by the bicinchoninic acid test.18 The phthalocyanine recovery was expressed as nanomoles of ZnB4Pc per mg of cell protein.
For phototoxicity experiments, the cells were seeded in Petri dishes (35 mm diameter) at a density of 105 cells cm−2 and grown in DMEM with 10% FCS. After 24 h the growth medium was removed, replaced with DMEM containing 3% FCS and the desired concentration of ZnB4Pc incorporated in DOPC liposomes and the cells were incubated at 37 °C for 24 h. At the end, the ZnB4Pc-containing medium was removed, the cells were washed twice with 2 ml of PBS containing Ca2+ and Mg2+, and irradiated in the same buffer for various periods of time with 600–700 nm light (Waldmann lamp). The light fluence-rate at the level of the cell monolayer was 50 mW cm−2. At 24 h after irradiation the cells were washed twice and trypsinized. Then a standard trypan blue staining was performed19 and the surviving cells were counted by using a Burker chamber. The percent survival was calculated by comparing the dye-incubated and -irradiated counts to those obtained for cells irradiated in the absence of phthalocyanine. Irradiation alone or incubation with phthalocyanine in the dark had no detectable effect on cell survival.
Fluorescence microscopy
The cells were seeded on a cover glass in Petri dishes as described for the phototoxicity experiments. The intracellular localization of ZnB4Pc after 24 h incubation of unirradiated, as well as of photosensitised cells (600–700 nm, 50 mW cm−2, 2 min exposure to light) was determined by an Olympus IMT-2 fluorescence microscope equipped with a refrigerated CCD camera (Micromax; Princeton Instruments). A 75 W xenon lamp was used as the excitation source. Fluorescence images obtained with a 60 × 1.4 NA oil immersion objective (Olympus) were acquired with the imaging software Metamorph (Universal Imaging). The cellular distribution of ZnB4Pc was compared with that obtained for the fluorescent probe Lucifer Yellow CH (Molecular Probes), used as a marker for endosomal membranes. Appropriate excitation and emission filters (Omega Optical Filter) were used: 655/710 nm for ZnB4Pc and 440/550 nm for Lucifer Yellow.
Measurements of caspase-3 activity
The activity of caspase-3 was measured in the phthalocyanine-photosensitised cells (incubation with 7 µM ZnB4Pc for 24 h, irradiation for 15 min at 50 mW cm−2) at 2 h and 6 h after the end of photosensitization. The ApoAlert CPP32 kit (Clontech, Palo Alto, CA) was used. According to the manufacturer recommended procedure, 106 cells were collected by centrifugation, suspended in 50 µl of lysis buffer, and held for 10 min on ice. Then, 50 µl of reaction buffer containing dithiothreitol and 5 µl of the fluorogenic caspase-3 substrate Asp-Glu-Val-Asp-7-amino-4-trifluoromethyl-coumarin were added to the cell lysate, and the fluorescence emitted at 505 nm (λex
= 400 nm) was measured with a Perkin-Elmer LS50 spectrophotofluorimeter. The caspase-3 activity in the treated cells was expressed as x-fold increase of the emitted fluorescence, taking the fluorescence from untreated cells as a reference.
Annexin-V-FLUOS assay
After photosensitization of cells seeded on a cover slip (incubation with 7 µM ZnB4Pc for 24 h, irradiation for 15 min at 50 mW cm−2), the dishes were incubated for 15 min at 25 °C with 250 µl of an aqueous solution containing 140 mM NaCl, 5 mM CaCl2, 10 mM Hepes–NaOH (pH 7.4), 2 µM propidium iodide and annexin-V-FLUOS (Roche Molecular Biochemicals) to a final dilution of 1 : 25 (v/v). Cells were washed twice with PBS without Ca2+ and Mg2+ before analysis. Cellular fluorescence images were acquired with an Olympus IMT-2 fluorescence microscope equipped with the refrigerated CCD camera using a 75 W xenon lamp as the excitation source. Fluorescence images obtained with a 60 × 1.4 NA oil immersion objective (Olympus) were acquired with the imaging software Metamorph (Universal Imaging). From each cover slip stained with annexin-V-FLUOS and propidium iodide some fields were randomly chosen and excited with light at 488 nm and 568 nm, respectively. The images were acquired using a fluorescence emission of 525 nm for annexin-V-FLUOS (green) and 585 nm for propidium iodide (red). The two images were overlaid using an appropriate function of the Metamorph software, and the cells were counted and classified like positive for annexin-V-FLUOS if they showed green fluorescence and positive for propidium iodide if they showed red fluorescence.
Pharmacokinetic studies with tumour-bearing mice
The experimental models used for in vivo studies were female C57BL/6 mice (20–22 g body weight) obtained from Charles River Laboratories (Como, Italy). The B16F1 pigmented melanoma was subcutaneously transplanted into the upper flank of the mice by injecting 20 µl (106 cells) of a sterile cell suspension in PBS without Ca2+ and Mg2+. After transplantation (8 days), when the tumour diameter was about 0.6 cm, the B16F1 tumour-bearing mice were intravenously injected with the DOPC liposome-embedded ZnB4Pc (3 mg kg−1). At predetermined times after injection, the mice (5 animals at each time point) were sacrificed by cervical dislocation and the ZnB4Pc content in the serum and selected tissues was determined independently for each sample by a spectrophotofluorimetric procedure.20 The blood was centrifuged (15 min at 3000 rev min−1) to remove the erythrocytes and appropriately diluted with 2% aqueous SDS. The tissue specimens were homogenized in 2% aqueous SDS, the homogenate was diluted with a chloroform–methanol mixture (1 : 2, v/v) and centrifuged for 10 min at 3000 rev min−1; the supernatant was collected and analysed for its ZnB4Pc content by fluorescence spectroscopy (excitation at 620 nm, emission at 630–800 nm), using a calibration plot built with known concentrations of ZnB4Pc in the same solvent mixture.
Experimental phototherapeutic studies
The PDT studies in vivo were performed at different time intervals (3, 24, 48 h) after the i.v. injection of a ZnB4Pc (3 mg kg−1) suspension in DOPC liposomes into the caudal vein of B16F1 tumour-bearing mice (five mice per experimental group); in all cases, the neoplastic lesion was irradiated at 670 nm by means of a diode laser equipped with an optical fiber (100 mW cm−2, 150 J cm−2). After irradiation the tumour size was measured daily by means of a calliper. Individual tumour volumes (V) were calculated by assuming a hemiellipsoidal structure for the tumour nodule and measuring the two perpendicular axes (a and b) and the height (c). Application of the relationship:
provided the tumour volume. The number of days for the tumour volume to grow from its initial value of 0.02 ± 0.005 cm3 to a value of 0.4 cm3 was calculated for the individual neoplastic lesions. The mice were treated according to the rules established by the University of Padova ethical committee for humane treatment of experimental animals and sacrificed by euthanasia before the tumour development became too large in order to minimize the risk of undue suffering and spreading of the malignancy across the body through lung or liver metastases. The effectiveness of the treatment was evaluated by comparing the rate of tumour growth of the irradiated mice with that observed for control mice transplanted simultaneously but not injected with ZnB4Pc and unirradiated.
Experimental BNCT studies
The BNCT studies were performed at the TAPIRO, a low power (5 kW nominal) fast flux research reactor, which is located at the ENEA Casaccia Research Center near Rome, and foreseen to be implemented as the national facility devoted to supply an epithermal neutron beam required for malignant brain tumour treatment.21 The feasibility to provide both thermal and epithermal suitable neutron beams in the main experimental cave of the TAPIRO reactor had already been ascertained.22
Reactor irradiations.
A spectrum shifter device prototype, aimed at slowing the fast neutrons emerging from the reactor core down to the thermal energy range, i.e.E < 0.4 eV, was designed and installed, devoted to basic trials on small laboratory animals. The thermal beam shaping assembly is an irradiation cavity of 18 × 18 × 18 cm3 box, located in the middle of a RG-Graphite structure, close to the reactor core in order to provide intense thermal fluxes. The reactor operating power level (limited at 4.7 kW) multiplied by the exposure time (given in units of MW min), which is proportional to the thermal neutron fluence, has been assumed as a reference parameter for all the invivo trials. Notwithstanding the very limited power provided, 1 MW min yields ∼5.6 × 1013 nth cm−2 inside the irradiation cavity with a uniform spatial distribution, that is in no way driven by wall effects, which is about 60 times higher than the thermal irradiation facility provided at the Brookhaven Medical Research Reactor (BMRR).23 A series of gold-foil activation technique was used to assess the thermal neutron flux level at different points inside the irradiation cavity which confirmed the calculation results within 10% uncertainties.
Tumour model.
In order to simulate the mouse, a simple cylindrical geometry model, reported in Fig. 1, has been implemented in all simulations. The model has the same mean length of the C57/BL6 mouse (9.5 cm) and the same mean total mass (21 g). The “muscle” ICRU 46 standard material,24 with a mass density of 1.07 g ml−1, has been used thus yielding a 0.85 cm cylinder radius. Two additional spherical regions, one simulating the liver mass (∼1 g), located along the cylinder axis, and the other one simulating the tumour bulk mass (∼0.1 g), have been created just below the cylinder side surface. Then, a 1 mm thick, 4 cm long, 10B enriched, boric acid (H310BO3) cap was added to the computational model in order to simulate the coating adopted to partially cover the body of the irradiated mice, in order to minimize the thermal neutron flux in the liver (see Results).
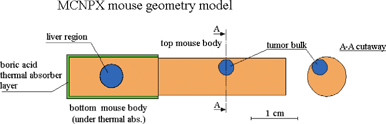 |
| Fig. 1 Geometrical model of the irradiated mouse used in Monte Carlo absorbed dose calculations. | |
Dosimetry.
The mixed radiation field results in energy deposition contributions of different ionizing radiation components. Three fundamental parameters have been calculated in the simulation trials: (a) the dose delivered by neutrons Dn inside hydrogenated materials, due to both the thermal neutron capture reactions by nitrogen nuclei 14N(n,p)
14C and the proton recoil ones by fast neutrons 1H(n,n′)
1H; (b) the gamma component Dγ, due to the direct background contribution generated by neutron slowing down process, as well as the induced radiative capture reactions 1H(n,γ)
2H; (c)
DBNC, that is the selective contribution by heavy charged particles from boron neutron capture reactions 10B(n,α)
7Li. An extensive computational investigation of all reactor facilities has been performed using the latest MCNPX-2.5e Monte Carlo code version25 in order to assess the macroscopic dose estimation inside the main body parts of the irradiated mouse. Dose rate calculations have been carried out either assuming charged particle equilibrium process, using the last updated ICRU 63 flux-to-kerma conversion factors26 or directly by the MCNPX tool. Dose-rate measurements from both gamma and fast neutron components have also been assessed with a special tissue equivalent proportional counter (TEPC) prototype designed and developed at LNL for monitoring the future therapeutic neutron beams.27
Experimental design.
The neutron irradiation experiments were carried out 24 h after injection of ZnB4Pc (3 mg kg−1) to B16F1 tumour-bearing mice. Typically, groups of four animals were placed inside the cavity and irradiated to 2.4 × 10−2, 4.7 × 10−2 and 9.4 × 10−2 MW min, respectively, at the maximum operative reactor power. A total of 4 animals were used for each time point. The tumour size was measured daily and the effectiveness of the treatment was evaluated by comparing the rate of tumour growth of the irradiated and injected mice with that observed for mice uninjected but irradiated and control mice uninjected and unirradiated.
Statistical analysis
All results are reported as mean ± standard deviation. Results were submitted to one-way analysis of variance (ANOVA). The Student–Newman–Keuls multiple comparison of means test was used to assess the significance of the in vivo PDT and BNCT data (p < 0.05).
Results
The synthesis of phthalocyanines was accomplished as reported in Scheme 1. Phthalonitriles 2–3 were synthesized by reaction of the lithium salt of o-carborane28 with the bromide 1 in anhydrous THF. Compound 2 was obtained by using natural isotopic abundance o-carborane (55% yield), whilst for the synthesis of the phthalonitrile 3, bromide 1 was allowed to react with isotopically enriched o-carborane (47% yield). Condensations of the phthalonitriles 2–3 to the corresponding Zn(II)–phthalocyanines 4–5
(1,8(11),15(18),22(25)- tetrasubstituted with 11B- and isotopically 10B-enriched carboranylmethylphenoxy units respectively) were carried out by heating a finely ground mixture of 2–3, respectively, and zinc acetate at 220 °C for several hours. Compounds 4 and 5 were obtained as a statistical mixture of regioisomers, in 40% yield; such results are in agreement with the typical yields reported for this kind of reaction,29 showing that the presence of the carborane cage does not impair the formation of the phthalocyanine. All the compounds obtained were characterized by means of 1H and 13C NMR spectroscopy, UV-visible spectroscopy and mass spectrometry.
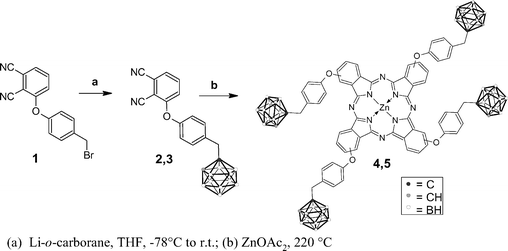 |
| Scheme 1 Scheme of the chemical synthesis of ZnB4Pc. Note that boron atoms are 11B in structures 2 and 4, and 10B in structures 3 and 5. | |
Physico-chemical properties of ZnB4Pc in the ground and electronically excited states
The natural isotopic abundance ZnB4Pc was used in all of our experiments except those involving the irradiations with thermal neutrons, where the much more expensive 10B compound was necessarily used. Spectroscopic and chromatographic analyses, as detailed in the experimental section, showed that the boronated phthalocyanine was stable for at least one week in both a liposomal aqueous suspension and DMF solution, as well as when incubated overnight in the presence of 50% human serum. Particular attention was focused on the lack of hydrolytic cleavage of the chemical bonds connecting the tetraazaisoindole macrocycle to the peripheral substituents bearing the carborane clusters, which would guarantee that the phthalocyanine can properly act as a vehicle of the boron atoms to the tumour lesion in experimental animals. The presence of the four p-carboranyl-substituted phenoxy moieties in the α or β positions of the outer benzene rings brings about a bathochromic shift (from 677 to 690 nm) of the lowest energy absorption maximum as compared with the unsubstituted Zn(II)–phthalocyanine (ZnPc). On the other hand, the fluorescence emission quantum yield of ZnB4Pc was found to be 0.31, i.e. somewhat higher than the 0.20 quantum yield found for ZnPc under identical experimental conditions:17 even so, one can safely expect that the boronated derivative is characterized by a high triplet quantum yield, since phthalocyanines generally exhibit an inefficient non-radiative decay from the first excited singlet state owing to the low vibrational component of their 0–0 transition.5 In actual fact, the DMA photooxidation studies (Fig. 2a) indicate that ΦΔ for this phtahlocyanine is 0.67, as compared with 0.55 found for ZnPc.17 Therefore, the triplet quantum yield of ZnB4Pc cannot be smaller than 0.67. At the same time, ZnB4Pc appeared to be an efficient photosensitiser for NATA, i.e. a substrate which can be photooxidized via both a type I (radical-involving) and a type II (singlet oxygen-involving) pathway:10 as shown in Fig. 2b, the first-order rate constant for the photooxidative modification of the tryptophyl residue (kv
= 4.06 × 10−4 s−1) is of the same order of magnitude as that typical of other photodynamically active photosensitising agents.5,10 The main photophysical properties of ZnB4Pc are summarized in Table 1. Since the photophysical and photosensitising properties for the α- and β-substituted derivatives were essentially identical, only the α-substituted ZnB4Pc was used in the subsequent studies with cells and animals.
Table 1 Selected photophysical properties of ZnB4Pc
k
v NATAa photooxidation/s−1 |
Φ
Δ
b
|
Φ
F
c
|
k
v photobleaching/s−1 |
N-acetyl-L-tryptophanamide; kv
= first-order rate constant.
Singlet oxygen quantum yield.
Fluorescence emission quantum yield.
|
4.06 × 10−4 |
1.23 |
0.31 |
0.16 |
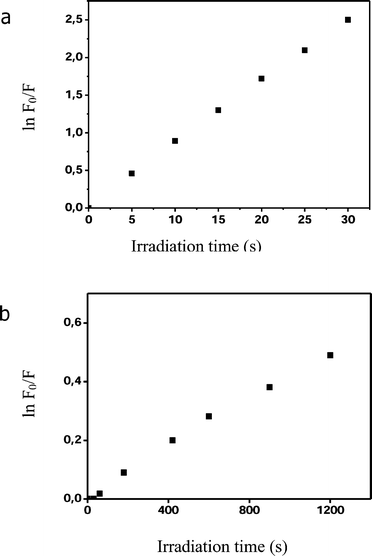 |
| Fig. 2 Determination of the photosensitising efficiency of 1.7 µM ZnB4Pc against (a) 20 µM 9, 10-dimethyl-anthracene and (b) 20 µM N-acetyl-L-tryptophanamide. All irradiations were performed in N,N-dimethylformamide by using 600–700 nm light at a fluence-rate of 100 mW cm−2. | |
As it is typical of most phthalocyanines,16 ZnB4Pc underwent a clearly detectable photobleaching when exposed to red light under our irradiation conditions. Typically, the intensity of the absorption bands peaking at 622 and 690 nm steadily decreased upon prolonging the irradiation time with no apparent formation of new bands throughout the visible and near-UV wavelength range. This indicates that the photoprocess brings about an irreversible modification of the phthalocyanine aromatic skeleton. Under our irradiation conditions, the photobleaching was characterized by a first-order kinetics at least during the initial phase (0–7 min) with a rate constant of 0.16 s−1.
ZnB4Pc-photosensitised inactivation of melanotic melanoma cells
The incubation of the melanotic melanoma cells with 7 µM ZnB4Pc incorporated in DOPC liposomes led to a time-dependent accumulation of the phthalocyanine. A plateau value corresponding with the recovery of about 0.6 nmol of phthalocyanine per mg of cell proteins was reached after 18 h incubation (Fig. 3); no additional increase in the amount of cell-bound ZnB4Pc was induced by extending the incubation time to 24 h. Under our experimental conditions, no appreciable cytotoxic effect due to the binding of the boronated derivative with the melanotic melanoma cells was detected.
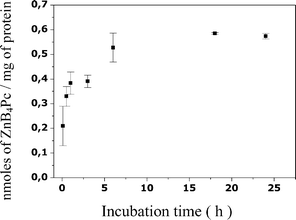 |
| Fig. 3 Recovery of ZnB4Pc from B16F1 melanotic melanoma cells as a function of the incubation time. Incubations were performed with 3 × 105 cells and 7 µM phthalocyanine and the recoveries (mean ± standard deviation of 3 separate experiments, each performed in triplicate) are expressed as nmol of ZnB4Pc per mg of cell protein. | |
The amount of cell-associated ZnB4Pc markedly influenced the degree of photosensitivity. Thus, red light irradiation of B16F1 cells, which had been incubated for 5 or 30 min with the 7 µM phthalocyanine, resulted in a limited decrease in survival even after cell exposure to light for time intervals as long as 15 min (Fig. 4). On the other hand, an almost complete (>95%) cell mortality was obtained when the irradiations were performed after 1 h incubation, and no further enhancement of the efficiency of the overall photoprocess was induced if the melanotic melanoma cells were incubated with the phthalocyanine for 24 h. Apparently, an endocellular phthalocyanine concentration of 0.38 nmol per mg of cell proteins, as reached after 1 h incubation, corresponds with maximum values for the photokinetic parameters controlling melanoma cell survival under our experimental conditions, so that an about 1.3-fold increment in the amount of cell-bound ZnB4Pc, as induced by prolonging the pre-irradiation incubation time, was not accompanied by any appreciable additional cell death at all the irradiation times examined by us (Fig. 4). The chromatographic behaviour and absorption/emission properties of the phthalocyanine extracted from extensively irradiated cells were identical with those measured for the unirradiated compound; this suggests that the boron clusters remain stably associated with the B16F1 cells even at the end of the photoprocess.
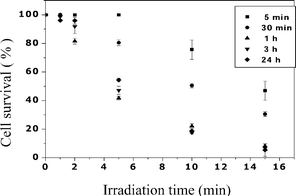 |
| Fig. 4 Effect of the irradiation time on the survival of B16F1 melanotic melanoma cells which had been incubated for different periods of time (see legend) with 7 µM ZnB4Pc. Irradiations were performed by using 600–700 nm light at a fluence-rate of 50 mW cm−2. Values represent mean ± standard deviation of 3 independently performed experiments. | |
In all cases, the irradiation of the B16F1 cells with red light but in the absence of the boronated phthalocyanine caused no detectable drop in survival of the B16F1 cells.
Mechanistic studies of melanoma cell photoinactivation by ZnB4Pc
Observation of the ZnB4Pc-loaded cells at the fluorescence microscope showed a discrete distribution of the photosensitising agent inside the cytoplasmic space (see Fig. 5b). In actual fact, the phthalocyanine fluorescence emission was found to yield an excellent overlap (Fig. 5d) with that observed for Lucifer Yellow, namely a typical endosomal probe (Fig. 5c). This strongly suggests that the two substances were localized in the same subcellular compartments. No major change in the above described fluorescence pattern was observed after the cells had been photoinactivated to a residual survival of about 7–10%, hence the irradiation should bring about no important relocalization of the ZnB4Pc from its original binding sites (data not shown).
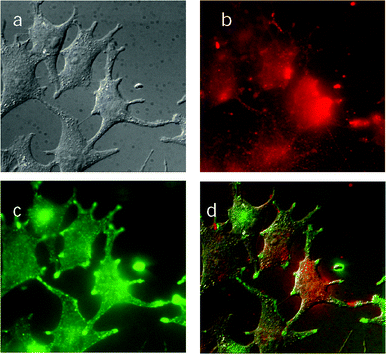 |
| Fig. 5 Fluorescence microscope images of B16F1 melanotic melanoma cells which had been incubated with 7 µM ZnB4Pc for 24 h. (a) Observations in a bright field; (b) fluorescence emission by the phthalocyanine (excitation at 655 nm); (c) fluorescence emission by Lucifer Yellow (excitation at 440 nm); (d) overlap of the three previous images. | |
The caspase test was carried out under irradiation conditions yielding an extensive cell death (7 µM ZnB4Pc, 24 h incubation, 15 min exposure to light). As one can see in Fig. 6a, a significant overexpression of caspase-3, i.e. an enzyme involved in the later stages of the cascade of events which are characteristic of cell apoptosis,30 took place 2 h after the end of irradiation; however, the amount of the apoptotic processes was largely reduced 6 h post-irradiation (Fig. 6a). The limited contribution of apoptotic events to cell death as a consequence of phthalocyanine-photosensitization was further confirmed by the results of the annexin test, which probes the initial stages of the apoptotic process, namely the photoinduced alteration in the microenvironment of phosphatidylserine in the cytoplasmic membrane (Fig. 6b): while a few photosensitised cells showed the green fluorescence typical of annexin, most cells became readily permeable to propidium iodide (as demonstrated by the red fluorescence spread throughout the cell volume), which is indicative of random necrosis.
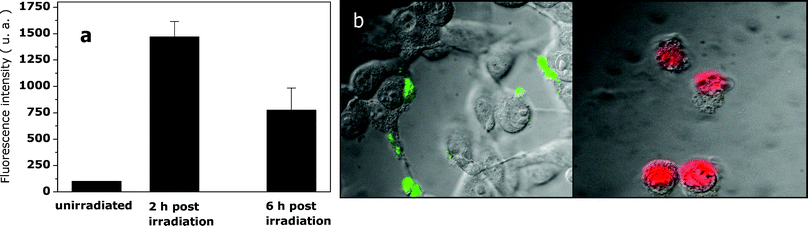 |
| Fig. 6 (a) Caspase-3 activity measured for control and photosensitised (15 min irradiation, 50 mW cm−2) B16F1 melanotic melanoma cells; measurements were made 2 h and 6 h after the end of the irradiation. (b) Annexin-V fluorescence assay for photosensitised cells: the images show the green fluorescence typical of annexin taken immediately after the end of irradiation, as well as the red fluorescence typical of propidium iodide taken 15 min after the end of irradiation. | |
Pharmacokinetic studies of ZnB4Pc in mice bearing a subcutaneously implanted melanotic melanoma
The recovery of ZnB4Pc was measured at different times after i.v. injection of the phthalocyanine to the tumour-bearing mice. The tissues selected for the dosage of the ZnB4Pc concentration included the melanotic lesion, the skin (peritumoural tissue), lung (which can frequently entrap colloidal particles), kidney and liver (to identify the main pathway of phthalocyanine elimination from the body), and spleen (since liposome-delivered drugs are known to be efficiently taken up by the components of the reticuloendothelial system).31,32
The data shown in Fig. 7a point out that the maximal accumulation of ZnB4Pc in the tumour tissue (1.64 nmol of phthalocyanine per g of tissue) occurred 24 h after injection. At this time, the selectivity of tumour targeting by the phthalocyanine was fairly good, since a 4 : 1 ratio of ZnB4Pc concentration in the tumour to skin was observed. The clearance of the photosensitising agent from the neoplastic lesion was at least biphasic: about 70% of the phthalocyanine was released by the tumour in the 24–28 h post-injection time interval, after which a slower phase started which was not completed one week after ZnB4Pc administration (Fig. 7a). No residual phthalocyanine was found to be present in the skin 96 h post-injection, which would strongly reduce the risk of persistent cutaneous photosensitivity consequent to the systemic injection of this derivative. Interestingly, the clearance of the phthalocyanine from the serum occurred rapidly, since only 3 h after injection was a reliably measurable amount of ZnB4Pc detected in the serum (Fig. 7b). The largest amount of the boronated derivative was found in the liver at all the time points analysed by us, as it is typical of hydrophobic compounds, which are predominantly eliminated from the organism via the bile–gut pathway.32
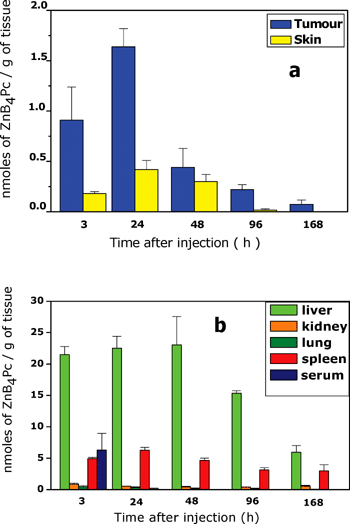 |
| Fig. 7 Time-dependence of ZnB4Pc distribution in (a) tumour and skin, and (b) selected normal tissues of C57BL/6 mice bearing a subcutaneously transplanted B16F1 melanotic melanoma. The mice were i.v.-injected with 3 mg kg−1 phthalocyanine and the histograms represent mean ± standard deviation of recoveries from at least 5 mice at each time point. 7 µM ZnB4Pc. | |
Experimental PDT studies
The 670 nm laser irradiation of the phthalocyanine-loaded melanotic melanoma 24 h and 48 h after the i.v-injection of the photosensitising agent caused no appreciable difference in the rate of tumour growth as compared with control untreated mice (see Fig. 8a). A clear response of the neoplastic lesion to the phototherapeutic protocol was observed only when the irradiation was performed 3 h post-injection: within about 24 h after the end of irradiation, the tumour developed a slight oedema followed by a flattening with no detectable regrowth for several days. The delay in tumour growth, as measured by comparing the time elapsed before the tumour volume reached 0.4 cm3 in control and phototreated mice, was 3.5 days (p < 0.05).
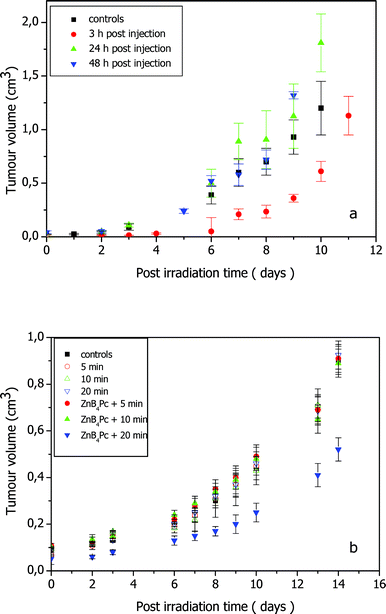 |
| Fig. 8 Tumour growth rate in C57BL/6 mice bearing a subcutaneously transplanted B16F1 melanotic melanoma that underwent PDT or BNCT treatment after injection of 3 mg kg−1 ZnB4Pc. (a) Irradiation with 600–700 nm light at a fluence-rate of 200 mW cm−2 and 3 h, 24 h and 48 h after photosensitizer injection; the growth rate is compared with that observed for uninjected and unirradiated control mice (five mice per group). (b) Irradiation with thermal neutrons for 5, 10 and 20 min 24 h after phthalocyanine injection; the growth rate is compared with that observed for uninjected and unirradiated control mice and mice irradiated for 5, 10 and 20 min in the absence of ZnB4Pc (four mice per group). | |
Preliminary investigations pointed out that irradiation of the tumour under identical experimental conditions but in the absence of ZnB4Pc caused no appreciable effect. In no case did the melanotic melanoma undergo spontaneous regression or remission in control mice.
Experimental BNCT studies
Our preliminary BNCT experiments were performed 24 h after ZnB4Pc injection, i.e. a time where the pharmacokinetic data (Fig. 7) show a boron intratumoural concentration of 0.66 ppm. The amount of 10B in the liver at the same post-injection time was about 15-fold greater, hence it was necessary to cover the mouse body (with the exception of the melanotic area and the strictly peritumoural districts) with a 10B-based coat, in order to protect the healthy tissues from any neutron-induced damage.
The reactor power history, including the rise-drop stages, was monitored throughout the experiment by a fission chamber inserted inside the reactor core. The absorbed dose rate was assumed to be proportional to the monitor current which, integrated along all the radiation exposure time, provided the total absorbed dose. The calculated mean fluence-rate inside the tumour bulk was ∼9.36 × 1011 n cm−2 s−1 MW−1, 98% of which was due to the thermal component. The dose-rate contribution from protons produced in the 14N(n,p)
14C thermal neutron capture reaction based on the given N nuclei content in tissue was calculated as ∼9.7 Gy (MW min)−1 and that originating from the 10B neutron capture reaction was calculated as ∼3.6 Gy (MW min)−1 for each microgram of 10B per gram of tissue based on the assumption of a uniform boron distribution. The fast neutron and gamma dose rates were ∼1.1 Gy (MW min)−1 and ∼36.2 Gy (MW min)−1. Therefore the average total dose rate in boron-free tumour bulk was ∼47 Gy (MW min)−1. The three dose components have been calculated at the level of the tumour bulk, in the liver and in the whole body. DBNC calculations were based on a measured mean 10B uptake of 0.66 ppm in the tumour tissue and 9.0 ppm in the liver (see above). The calculation uncertainties are standard deviations of the data after 108 Monte Carlo particle histories starting from a neutron generated inside the reactor core.
As shown in Fig. 8b, no influence on the rate of tumour growth was observed in mice that had been exposed to the thermal neutron flux for 2.4 × 10−2 or 4.7 × 10−2 MW min (5 or 10 min). On the other hand, a significant delay in tumour growth (4 days, p < 0.05) was induced by 9.4 × 10−2 MW min (20 min) neutron irradiation. No detectable tumour response was obtained upon irradiation under identical conditions but in the absence of ZnB4Pc. In order to corroborate such observations, the neutron irradiation studies described in Fig. 8b were repeated under identical experimental conditions by using another set of mice bearing the melanotic melanoma. As it often happens for transplanted tumours, the melanoma growth rate for the second group of mice was slightly different from that described in Fig. 8b. Once again, all the groups of irradiated animals showed a time-dependent increase in the tumour volume which closely overlapped that found for the control unirradiated mice, except for the group of phthalocyanine-injected mice that were irradiated for 20 min where a 4.5 day growth delay was obtained.
Since 4 mice have been simultaneously irradiated placing them side by side, the dose has been separately calculated for the two centrally located mice (inner position) and the two mice placed at the external positions (outer position) in order to investigate possible shielding effects. In Table 2 the absorbed dose components are reported for the 20 min irradiation experiments. Comparison among inner-mice and outer-mice data pointed out no significant difference in all the absorbed dose components. DBNC inside the tumour was about 1/4 of the neutron dose and about 1/15 of the gamma dose. In any case, the albeit relatively intense gamma radiation caused no major effect on the tumour, as shown by the coincidence in the tumour growth rate between unirradiated controls and mice irradiated without prior injection of the phthalocyanine.
Table 2 Absorbed dose components, averaged over the two mice in the outer position and over the two mice in the inner position, in tumour bulk, liver and whole mouse for 9.4 × 10−2 MW min (20 min) exposure experiment
|
Inner position (average) |
Outer position (average) |
D
n tot/Gy |
D
γ/Gy |
D
BNC/Gy |
D
n tot/Gy |
D
γ/Gy |
D
BNC/Gy |
Tumor bulk |
1.03 ± 0.03 |
3.40 ± 0.18 |
0.22 ± 0.002 |
1.03 ± 0.03 |
3.28 ± 0.17 |
0.22 ± 0.002 |
Liver |
0.14 ± 0.01 |
5.63 ± 0.07 |
0.23 ± 0.003 |
0.15 ± 0.01 |
5.58 ± 0.08 |
0.24 ± 0.003 |
Whole body |
0.63 ± 0.004 |
4.60 ± 0.04 |
— |
0.65 ± 0.005 |
4.44 ± 0.03 |
— |
Discussion
Several carboranyl–porphyrin derivatives are being considered as sensitisers for a dual, possibly combined, application in BNCT and PDT of specific tumours, including meso-tetra-substituted porphyrins33 and chlorins.34 The tumour-localized tetrapyrrole compound can be sequentially activated by low energy neutrons to generate mainly α and Li particles and by red light wavelengths to produce singlet oxygen and other reactive oxygen species (the most convenient order of application of the two therapeutic modalities is still to be determined). Such hyper-reactive intermediates are characterized by a short (less than one cell diameter) path of travel in a tissue, hence the overall process is often characterized by a high degree of selectivity as regards tumour damage.35,36 The Zn(II)–phthalocyanine with four peripheral carborane cages, bearing 28% boron by weight, synthesized by us appears to represent a promising addition to this class of compounds. This conclusion is based on the following considerations.
(1) The ether bonds connecting the four carborane cages to the main tetraazaisoindole macrocycle were found to be very stable in a variety of biologically relevant media, as well as in cells. Such linkages are not attacked by serum or endocellular esterases and are not readily hydrolysed under physiological conditions. Therefore, this phthalocyanine can represent a safe and reliable vehicle for transport of the boron clusters to malignant tissues in vivo.
(2) The photophysical and photokinetic properties of ZnB4Pc are very similar to those typical of other efficient photodynamic sensitisers. Since phthalocyanines largely carry out their photosensitising action via singlet oxygen,5,14 particular attention deserves the measured 0.67 quantum yield for the generation of this oxygen species by photoexcited ZnB4Pc, which means that a high fraction of the absorbed photons promote a chemical reaction, namely an oxidative attack on a variety of cell/tissue constituents, including unsaturated lipids, aromatic and sulfur-containing amino acid residues and guanosine bases.7 The multi-target nature of the overall photosensitised damage makes it unlikely that cells undergoing a variety of irreversible modifications can develop repair or protective processes.
(3) ZnB4Pc is taken up with a high efficiency by melanotic melanoma cells in spite of the steric hindrance caused by the bulky p-(methyl-carboranyl)phenoxy moieties. Moreover, it induces no detectable cytotoxic effects in the dark at concentrations which allow an extensive cell photoinactivation. These considerations appear to be of general validity since essentially identical results were obtained with quite different cell types, including transformed human fibroblasts and keratinocytes (data not shown).
(4) In actual fact, the melanotic melanoma cells, which usually display a low photosensitivity, are extensively (>95%) killed by using relatively mild irradiation protocols, such as 1 h incubation with a ZnB4Pc dose as low as 7 µM, and 15 min irradiation at a 50 mW cm−2 fluence-rate (see Fig. 4). The high efficiency of the photoprocess can be ascribed at least in part to the partitioning of the photosensitising agent in several subcellular membranes, as suggested by the distribution pattern observed at the fluorescence microscope (Fig. 5); this would result in an early photoinduced impairment of several metabolic functions37,38 and a predominant cell death through random necrotic pathways, as one can deduce from the data presented in Fig. 6.
(5) ZnB4Pc is a highly hydrophobic molecule, hence it can be delivered in in vivo systems only after its embedding in suitable vehicles, such as phospholipid vesicles. This constitutes a favourable feature since the DOPC liposomes used by us are known39 to preferably release the incorporated dye to serum low-density lipoproteins (LDL), which undergo an efficient internalization by many types of neoplastic cells via receptor-mediated endocytosis.31 Thus, as shown by our pharmacokinetic studies, a high selectivity of tumour targeting by ZnB4Pc was achieved (Fig. 7a), at least in the animal model utilized in the present investigations. The fairly rapid clearance of the boronated phthalocyanine by skin and serum (Fig. 7b) would also guarantee against the onset of persistent cutaneous photosensitivity, which represents a frequent undesired side effect of PDT.38
(6) Melanotic melanoma is known to give a poor response to PDT mainly because of the light filtering action brought about by the large melanin pigmentation.40,41 Thus, no effect on the rate of tumour growth was caused by PDT treatment of the melanotic lesion 24 h or 48 h after the administration of the phthalocyanine, when the photosensitiser should be largely located in endocellular compartments since no residual amount of ZnB4Pc can be recovered from the serum. On the contrary, if the irradiation was performed 3 h after injection of ZnB4Pc, a significant damage of the neoplastic tissue took place, in spite of the lower amount of tumour-associated photosensitiser; such a time-dependency of tumour response strongly suggests that the photodamage is largely a consequence of PDT action on the blood vessels rather than a direct effect on malignant cells.
(7) The boron concentration in the melanotic melanoma was enough to induce a statistically significant tumour growth delay upon BNCT treatment 24 h after ZnB4Pc administration, provided a sufficient exposure was used, namely 9.4 × 10−2 MW min or ∼4.6 Gy average tumour dose (∼4.4 Gy reactor radiation + 0.66 µg g(10B contribution)−1). The experiment was carried out at the time point corresponding with the maximal accumulation of ZnB4Pc, since this factor appears to be critical for ensuring an efficient interaction of the 10B isotope with the incident radiation.32,33 It must be underlined that boron concentrations in the tumour of the order of 15–30 ppm are generally assumed to be required for achieving an important therapeutic effect.42 This conclusion is based on the assumption of a homogeneous distribution of the boron in the whole neoplastic tissue. In actual fact, previous findings by Coderre et al.23 on the BNCT treatment of melanotic melanoma in C57 mice upon boron delivery via borono-phenylalanine, as well as of Miura et al.43 on BNCT of EMT-6 mammary carcinoma in Balb/c mice in the presence of a carboranyl-tetraphenylporphyrin, showed that a satisfactory response in terms of the survival of tumour-bearing mice could be obtained by using larger reactor doses and markedly greater boron doses (e.g., 4.7 Gy reactor radiation + 85 µg g(10B)−1 for the porphyrin). The large boron doses in the tumour, which were achieved by multiple intraperitoneal injections of the radiosensitiser, were justified by the likely microheterogeneous distribution of the intrinsically hydrophobic porphyrin molecule. Our present observations that a much lower amount of boron in the subcutaneous melanoma, namely 0.66 ppm, can still induce a modest, yet appreciable response at least of this malignant lesion can be explained by the fact (see Fig. 5) that ZnB4Pc is actually partitioned in specific subcellular compartments; such a distribution pattern is typical of several hydrophobic phthalocyanines which are known to be specifically accumulated by cell membranes.16,32 As a consequence, the local phthalocyanine concentration in selected sites could be sufficiently high to warrant an efficient interaction with thermal neutrons leading to irreversible damage of organelles, such as lysosomes or the Golgi apparatus, whose integrity is critical for cell metabolism and survival. The photoinactivation data obtained with cultured melanotic melanoma cells support this hypothesis. The high cost of the 10B-containing compound and the limited access to the thermal neutron source at this preliminary stage of our investigations precluded us from further extending this specific segment of the work plan. However, studies aimed at the characterization of the neutron-induced cell/tissue damage by optical and electron microscopy are presently in progress. Moreover, a more thorough investigation on the relationship between subcellular distribution of the boronated derivative and the response of tumours to irradiation with different thermal neutron doses is certainly necessary. This information could provide a basis for the prospect of obtaining a synergistic or cumulative action of PDT and BNCT.
In conclusion, ZnB4Pc displays tumour-targeting and PDT properties which are comparable with those observed for other therapeutically efficacious phthalocyanines; moreover, if proper irradiation conditions are adopted, ZnB4Pc can also sensitise tumours to the action of thermal neutrons, in particular a higher dose of phthalocyanines may result in significant tumour palliation. So far, our investigations were focused on a subcutaneous tumour model, the melanotic melanoma, essentially as a proof of principle, taking advantage of its easy accessibility to both types of radiation and the ample possibility to improve the presently unsatisfactory effect of PDT by a combination therapy. However, a number of metastases arising from the primary pigmented melanoma could also be usefully treated by PDT + BNCT.44
Acknowledgements
This research has been financially supported by the Italian Institute for Nuclear Physics (INFN). We are indebted to Dr Karel Base (Duke University Medical Centre, Durham, North Carolina) for providing 10B-labelled carboranes.
References
- A. Z. Diaz, J. A. Coderre, A. D. Chanana and R. Ma, Boron neutron capture therapy for malignant gliomas, Ann. Med., 2000, 32, 81–85 Search PubMed.
- R. Ackroyd, C. Kelty, N. Brown and M. Reed, The history of photodetection and photodynamic therapy, Photochem. Photobiol., 2001, 74, 656–659 CAS.
- R. F. Barth, A. H. Soloway, J. H. Goldman, R. A. Gadbauer, N. Gupta, T. E. Blue, W. Yang and W. Tjarks, Boron neutron capture therapy of brain tumours: an emerging therapeutic modality, Neurosurgery, 1999, 44, 433–451 Search PubMed.
- B. W. Henderson and T. J. Dougherty, How does photodynamic therapy work?, Photochem. Photobiol., 1999, 55, 145–147 Search PubMed.
- M. Ochsner, Photophysical and photobiological processes in photodynamic therapy of tumours, J. Photochem. Photobiol., B, 1997, 39, 1–18 CrossRef CAS.
- R. F. Barth, W. Yang, J. D. Rotaru, M. L. Moeschberger, C. P. Boesel, A. H. Soloway, D. D. Joel, M. M. Nawrocky, K. Ono and J. H. Goodman, Boron neutron capture therapy of brain tumours: enhanced survival and cure following blood-brain barrier disruption and intracarotid injection of sodium borocaptate and boronphenylalanine, Int. J. Radiat. Oncol. Biol., Phys., 2000, 47, 209–218 CrossRef CAS.
-
R. Bonnett, Chemical Aspects of Photodynamic Therapy, Gordon and Breach Science Publishers, Amsterdam, 2000 Search PubMed.
- M. Miura, P. L. Micca, C. D. Fisher, C. R. Gordon, J. C. Heinrichs and D. N. Slatkin, Evaluation of carborane-containing porphyrins as tumour targeting agents for boron neutron capture therapy, Br. J. Radiol., 1998, 71, 773–781 Search PubMed.
- S. B. Kahl and J. Li, Synthesis and characterization of a boronated metallo–phthalocyanine for boron neutron capture therapy, Inorg. Chem., 1996, 35, 3878–3880 CrossRef CAS.
-
G. Jori, Photodynamic therapy: basic and preclinical aspects, in CRC Handbook of Organic Photochemistry and Photobiology, ed. W. Horspool and F. Lenci, CRC Press, Baton Rouge, 2nd edn, 2004, pp. 146-1–146-10 Search PubMed.
- C. Fabris, G. Jori, F. Giuntini and G. Roncucci, Photosensitising properties of a boronated phthalocyanine: studies at the molecular and cellular level, J. Photochem. Photobiol., B, 2001, 64, 1–7 CrossRef CAS.
-
W. L. F. Armarego and D. D. Perrin, Purification of Laboratory Chemicals, Butterworth-Heinemann, Oxford, 4th edn, 1996 Search PubMed.
- F. Giuntini, Y. Raoul, D. Dei, M. Municchi, G. Chiti, C. Fabris, P. Colautti, G. Jori and G. Roncucci, Synthesis of tetrasubstituted Zn(II)–phthalocyanines carrying four carboranyl-units as potential BNCT and PDT agents, Tetrahedron Lett., 2005, 46, 2979–2982 CrossRef CAS.
- G. Valduga, S. Nonell, E. Reddi, G. Jori and S. Braslavsky, The production of singlet molecular oxygen by Zinc(II)–phthalocyanine in ethanol and in unilamellar vesicles. Chemical quenching and phosphorescence studies, Photochem. Photobiol., 1988, 48, 1–5 CAS.
- E. Gross, B. Ehrenberg and F. Johnson, Singlet oxygen generation by porphyrins and the kinetics of 9,10-dimethyl-anthracene photosensitisation in liposomes, Photochem. Photobiol., 1993, 57, 808–813 CrossRef CAS.
-
J. E. van Lier and J. D. Spikes, The chemistry, photophysics and photosensitising properties of phthalocyanines, in Photosensitising Compounds: their Chemistry, Biology and Clinical Use, ed. G. Bock and S. Harnett, Ciba Foundation Symposium 146, London, 1989, pp. 17–26 Search PubMed.
- G. Valduga, E. Reddi and G. Jori, Spectroscopic studies on Zn(II)–phthalocyanine in homogeneous and microheterogeneous systems, J. Inorg. Chem., 1987, 29, 59–65 Search PubMed.
- P. K. Smith, R. I. Krohn, G. T. Hermanson, A. K. Mallia, F. H. Gartner and M. D. Provenzano, Measurement of protein using bicinchoninic acid, Anal. Biochem., 1985, 150, 76–85 CrossRef CAS.
- S. Rockwell,
In vivo-in vitro tumour cell lines: characteristics and model for human cancer, Br. J. Cancer, 1980, 41, 118–126 CAS.
- C. Fabris, G. Valduga, G. Miotto, G. Jori, S. Garbisa and E. Reddi, Photosensitisation with Zn(II)–phthalocyanine as a switch in the decision between apoptosis and necrosis, Cancer Res., 2001, 61, 7495–7500 CAS.
- K. W. Burn, L. Casalini, S. Martini, M. Mazzini, E. Nava, C. Petrovich, G. Rosi, M. Sarotto and R. Tinti, An epithermal facility for treating brain gliomas at the TAPIRO reactor, Appl. Radiat. Isot., 2004, 61, 987–991 CrossRef CAS.
- G. Rosi, G. Gambarini, V. Colli, S. Gay, L. Scolari, O. Fiorani, A. Perrone, E. Nava, E. Fasola, L. Visca and A. Zanini, Characterisation of TAPIRO BNCT thermal facility, Radiat. Prot. Dosim., 2004, 110, 651–654 CrossRef CAS.
- J. A. Coderre, D. N. Slatkin, P. L. Micca and J. R. Cialella, Boron Neutron Capture therapy of a Murine Melanoma with p-Boronophenylanine: Dose-Response Analysis using a Morbidity Index, Radiat. Res., 1991, 128, 177–185 CrossRef CAS.
-
ICRU Report 46: Photon, Proton, Electron and Neutron Interaction data for body tissues, International Commission on Radiation Units and Measurements, 1992 Search PubMed.
-
J. S. Hendricks, MCNPX (Version 2.5e), Los Alamos National Laboratory Report, LA-UR-04-0569, February 2004 Search PubMed.
-
ICRU 63 Report: Nuclear Data for Neutron and Proton Radiotherapy and for Radiation Protection, International Commission on Radiation Units and Measurements, 2000 Search PubMed.
- L. De Nardo, E. Seravalli, G. Rosi, J. Esposito, P. Colautti, V. Conte and G. Tornielli, BNCT microdosimetry at the Tapiro reactor thermal column, Radiat. Prot. Dosim., 2004, 110, 579–586 CrossRef CAS.
- J. L. Maurer, F. Berchier, A. J. Serino, C. B. Knobler and M. F. Hawthorne, Preparation and properties of metallo–phthalocyanines, J. Org. Chem., 1990, 55, 838–843 CrossRef CAS.
-
C. C. Leznoff, Synthetic strategies for peripherally substituted phthalocyanines, in Phthalocyanines, Properties and Applications, ed. C. C. Leznoff and A. B. P. Lever, VCH Publishers Inc., New York, 1989, vol. 1, pp. 159–169 Search PubMed.
- N. Oleinick, R. L. Morris and I. Belichenko, The role of apoptosis in response to photodynamic therapy: what, where, why and how, Photochem. Photobiol. Sci., 2002, 1, 1–21 RSC.
-
G. Jori, Low-density lipoproteins-liposome delivery systems for tumour photosensitisers in vivo, in Photodynamic Therapy, ed. B. W. Henderson and T. J. Dougherty, Marcel Dekker Inc., New York, 1992, pp. 173–176 Search PubMed.
-
G. Jori, In vivo transport and pharmacokinetic behaviour of tumour photosensitisers, in Photosensitising Compounds: their Chemistry, Biology and Clinical Use, ed. G. Bock and S. Harnett, Ciba Foundation Symposium 146, London, 1989, pp. 173–186 Search PubMed.
- V. Gottumukkala, R. Luguya, F. R. Fronczek and M. G. H. Vicente, Synthesis and cellular studies of an octa-anionic 5,10,15,20-tetra[3,5-(nido-carboranylmethyl)phenyl]porphyrin (H2OCP) for application in BNCT, Biorg. Med. Chem., 2005, 13, 1633–1640 CrossRef CAS.
- R. Luguya, F. R. Fronczek, K. M. Smith and M. G. H. Vicente, Synthesis of novel carboranylchlorins with dual application in boron neutron capture therapy (BNCT) and photodynamic therapy (PDT), Appl. Radiat. Isot., 2004, 61, 1117–1123 CrossRef CAS.
- M. G. H. Vicente, Porphyrin-based sensitisers in the detection and treatment of cancer: recent progress, Curr. Med. Chem.:Anti-Cancer Agents, 2001, 1, 175–194 Search PubMed.
-
K. Berg, Mechanisms of cell damage in photodynamic therapy, in The Fundamental Basis of Phototherapy, ed. H. Hönigsmann, G. Jori and A. R. Young, OEMF, Milano, 1996, pp. 181–207 Search PubMed.
-
A. C. E. Moor, B. Ortel and T. Hasan, Mechanisms of photodynamic therapy, in Photodynamic
Therapy, ed. T. Patrice, Royal Society of Chemistry, Cambridge, 2003, pp. 19–57 Search PubMed.
- T. J. Dougherty, C. J. Gomer, B. W. Henderson, G. Jori, D. Kessel, M. Korbelik, J. Moan and Q. Peng, Photodynamic therapy, J. Natl. Cancer Inst., 1998, 90, 889–905 CrossRef CAS.
- G. Jori and E. Reddi, The role of lipoproteins in the delivery of tumour-targeting photosensitisers, Int. J. Biochem., 1993, 25, 1369–1375 Search PubMed.
- C. Fitsch, G. Goerz and T. Ruzicka, Photodynamic therapy in dermatology: a review, Arch. Dermatol., 1998, 134, 207–214 CAS.
-
P. G. Calzavara-Pinton, R. M. Szeimies and B. Ortel, Photodynamic Therapy and Fluorescence Diagnosis in Dermatology, ed. D. P. Häder and G. Jori, Elsevier Publishing Co., Amsterdam, 2001 Search PubMed.
- R. G. Fairchild and V. P. Bond, Current status of 10B boron neutron capture therapy: enhancement of the tumour dose via beam filtration and dose rate, and the effects of these parameters on minimum boron content; a theoretical evaluation, Int. J. Radiat. Oncol. Biol., Phys., 1985, 11, 831–840 CAS.
- M. Miura, G. M. Morris, P. L. Micca, D. T. Lombardo, K. M. Youngs, J. A. Kalef-Ezra, D. A. Hoch, D. N. Slatkin, R. Ma and J. A. Coderre, Boron neutron capture therapy of a murine mammary carcinoma using a lipophilic carboranyltetraphenylporphyrin, Radiat. Res., 2001, 155, 603–610 CAS.
- M. A. Rosenthal, B. Kavar, J. S. Hill, D. J. Morgan, R. L. Nation, S. S. Stylli, R. L. Basser, S. Uren, H. Geldard, M. D. Green, S. B. Kahl and A. K. Kaye, Phase I and pharmacokinetic study of photodynamic therapy for high-grade gliomas using a novel boronated porphyrin, J. Clin. Oncol., 2001, 19, 519–524 CAS.
|
This journal is © The Royal Society of Chemistry and Owner Societies 2006 |
Click here to see how this site uses Cookies. View our privacy policy here.