Pre-clinical in vitro and in vivo studies to examine the potential use of photodynamic therapy in the treatment of osteomyelitis
Received
19th May 2005
, Accepted 11th October 2005
First published on 8th November 2005
Abstract
Osteomyelitis can lead to severe morbidity and even death resulting from an acute or chronic inflammation of the bone and contiguous structures due to fungal or bacterial infection. Incidence approximates 1 in 1000 neonates and 1 in 5000 children in the United States annually and increases up to 0.36% and 16% in adults with diabetes or sickle cell anaemia, respectively. Current regimens of treatment include antibiotics and/or surgery. However, the increasing number of antibiotic resistant pathogens suggests that alternate strategies are required. We are investigating photodynamic therapy (PDT) as one such alternate treatment for osteomyelitis using a bioluminescent strain of biofilm-producing staphylococcus aureus (S. aureus) grown onto kirschner wires (K-wire). S. aureus-coated K-wires were exposed to methylene blue (MB) or 5-aminolevulinic acid (ALA)-mediated PDT either in vitro or following implant into the tibial medullary cavity of Sprague-Dawley rats. The progression of S. aureus biofilm was monitored non-invasively using bioluminescence and expressed as a percentage of the signal for each sample immediately prior to treatment. S. aureus infections were subject to PDT 10 days post inoculation. Treatment comprised administration of ALA (300 mg kg−1) intraperitoneally followed 4 h later by light (635 ± 10 nm; 75 J cm−2) delivered transcutaneously via an optical fiber placed onto the tibia and resulted in significant delay in bacterial growth. In vitro, MB and ALA displayed similar cell kill with ≥4log10 cell kill. In vivo, ALA-mediated PDT inhibited biofilm implants in bone. These results confirm that MB or ALA-mediated PDT have potential to treat S. aureus cultures grown in vitro or in vivo using an animal model of osteomyelitis.
1. Introduction
Osteomyelitis refers to an acute or chronic inflammation of bone and bone marrow secondary to contamination of pathogenic microorganisms (pyrogenic bacteria, mycobacteria, fungi).1 Whether by etiology or clinical presentation, the predominant factor predicting the progression of the disease and therapeutic outcome, regardless of anatomical location, is vascularity. Trivial traumas involving skin lacerations or bruising are often the culprit leading to blood-borne bacteremia, while avascular focus resulting from peripheral vascular disease, burns or soft tissue disease are usually prone to contiguous infection of neighboring tissues. This is particularly true for diabetics or patients with sickle cell anaemia, for whom the incidence of skeletal infection is increased approximately 20–1000 times, respectively.2,3Staphylococcus aureus (S. aureus) is the pathogen most commonly isolated in this and most other types of osteomyelitis, implicated in 60–90% of childhood osteomyelitis and greater than 55% in adults.4,5S. aureus are known to possess a number of inherent traits that contribute to their high prevalence and virulence6,7 yet, perhaps their most effective barrier to host defenses and antimicrobial agents are the propagation of biofilms. S. aureus produce a glycoprotein slime or glycocalyx biofilm that can adhere very effectively to host tissues and metallic implants, including pins and screws and prosthetic hips and joints, while at the same time providing protection to the embedded S. aureus cells. With more than one million new cases of hip replacement worldwide,8 the implantation of orthopaedic and prosthetic devices is recognized as one of the fastest emerging primary causes leading to acute osteomyelitis. For patients that present with septic prosthetic joints, the prosthesis is usually removed. However, the incidence of morbidity is typically high, as is the economic burden due to lengthy hospital stays and protracted follow-ups.
Current strategies for treatment include the parenteral administration of antibiotics for 4–6 weeks, with or without surgical debridement of necrotic tissues to allow revascularization of bone. Unfortunately, bacteria can develop resistance to antibiotics upon repeated use. In contrast with antibiotics, the chronic administration of photosensitizers for PDT has thus far not been found to confer resistance within mammalian or microbial cultures. This is a very significant distinction. However, photosensitizers, like antibiotics, require an intact vascular supply to access the site of infection, which for osteomyelitis requires rapid intervention within 10–20 days of infection, prior to the manifestation of osteolytic debris.9,10 Rapid diagnosis is, therefore, critical to ensure favorable outcome with either therapy. We hypothesize, that the versatility of PDT, with optimized drug/light regimens, state-of-the-art light sources and interstitial placement of optical fibers for light delivery, may allow for a superior therapy to that proffered by antibiotics, one that is both targeted specifically to the site of infection, thus minimizing the risk of collateral damage to ‘friendly’ host flora as can occur with systemic use of antibiotics,11 and one that is easily customized in real time to the specific stage and severity of infection.
The successful management of osteomyelitis remains challenging and increasingly expensive to treat despite advancements in antibiotics and surgical intervention. This study explores the opportunity of using PDT to treat contaminated implants within bone as a model for the onset of clinical osteomyelitis. Biofilm producing S. aureus. that had been engineered to bioluminesce were treated in vitro either as planktonic cell cultures in growth media or as established biofilms coating a length of titanium wire. Similar biofilm-coated wires were subsequently implanted into the tibia of rats and 10 days later subjected to PDT in vivo. Methylene blue (MB) or 5-aminolevulinic acid (ALA) were used as photosensitizers as both have been shown to have considerable photobactericidal activity against S. aureus.12,13 We were particularly interested in exploiting the endogenous up-regulation of porphyrins mediated by ALA, given the opportunity for oral administration and minimal dark toxicity in vivo. Despite the use of modest light dose and non-optimized light sources, the considerable decrease in bioluminescent signal following PDT, consistent with reduced S. aureus viability found in vitro, are conclusive in supporting the antimicrobial action of ALA-mediated PDT administered against S. aureus-derived biofilms implanted into the tibia of rats.
2. Materials and methods
2.1 Reagents
Methylene blue (MB; certified, ≥97% dye content) and arachidonic acid sodium salt (99%) were purchased from Sigma-Aldrich, Canada Ltd, Oakville, Ontario. 5-Aminolevulinic acid hydrochloride (ALA; >98%) was purchased from Frontier Scientific, Porphyrin Products, Logan, UT, USA.
2.2 Cells
A genetically engineered strain of S. aureus 12600 was purchased from Xenogen corp., Alameda, CA, USA. The modified strain, S. aureus Xen29 (Xen29) possess a stable copy of the Photorhabdus luminescens lux operon integrated at a single location within the bacterial chromosome using Gram-positive lux transposon plasmid pAUL-Atn 4001 luxABCDE kmr14 that allow the cells to be bioluminescent in the absence of exogenous luciferin substrate. Xen29 cells were initially cultured in 5% sheep blood agar (Krackeler Scientific, Inc., Albany, NY, USA) plates (Nalge Nunc International, Rochester, NY, USA) into single colonies and stored at 4 °C. Thereafter, they were grown in broth or cultured onto agar containing trypticase soy broth supplemented with 0.25% glucose (TSBG; Difco, Detroit, MI, USA). Prior to use, an aliquot (100 µL) of S. aureus cells was sub-cultured from an overnight growth culture into 10 mL of TSB for 2.5 h at 37 °C with shaking at 200 rev min−1 to achieve log-phase growth. A standard curve was recorded of colony forming units (CFU) mL−1 as a function of optical density (O.D.) at 600 nm blanked with TSBG.
2.3 Establishing S. aureus biofilm-coated K-wires
1 mL aliquots of 2.5 h Xen29 cells (∼106 CFU mL−1) in exponential growth phase were pipetted into Eppendorfs containing 0.5 mL TSBG and a 0.5–1 cm length of 0.2 mm thick, sterile titanium Kirschner (K)-wire (Small parts Inc., Logansport, IN, USA) and incubated at 37 °C for 6 h. Fresh TSBG was added and the cultures were returned to the incubator and fresh TSBG given at intervals of 12–16 h until S. aureus biofilm was clearly visible as a creamy colored slime along the length of the K-wire, usually after 3 days of culture.
2.4 Osteomyelitis model
Sprague-Dawley CD rats (n = 10, female 250–300 g) were anaesthetized via nose cone (2.5% isoflurane (Abbott Laboratories Ltd., Saint-Laurent, QC)–O2), their flanks shaved, cleansed with Betadyne and placed supine onto a warmed blanket. The knee joint was flexed to outline the proximal anterior margin of the tibial epicondyle. Bacterial inoculation was performed for both the left and right tibia on the same animal. A scalpel was used to open the skin (1 cm incision) and expose the underlying bone. The margins of adhered fascia were cleared using the scalpel and a small bore hole (0.1 cm) was created into the intramedullary space of the tibia using a high speed drill (Multipro™, Dremel, Racine, WI, USA) mounted with a sharp burr (Fissurotomy™, SS White Inc., Lakewood, NJ, USA). A sterile needle (25G) was used to create a small channel through the medullary space, into which was placed a biofilm-coated K-wire (0.5 cm long). 5 µL of arachidonic acid (50 µg mL−1 in 0.9% NaCl solution) was subsequently injected 30 s after the bacterial inoculum. Arachidonic acid acts as a sclerosing agent to facilitate the bacterial infection in bone.15 The burr hole was closed with bone wax (Ethicon® Inc., Johnson & Johnson, Markham, ON, Canada) and the skin sutured closed (4–0, silk, Ethicon®) and disinfected. Animals were given subcutaneous analgesic (buprenorphine; 0.05 mg kg−1) before being returned to their cages. K-wires were imaged 24 h later using a mini C-arm fluoroscopy unit (FluoroScan™ Imaging Systems Inc., Northbrook, IL, USA). Animal protocols were submitted under the animal use protocol number: UHN 04–131 and approved in accordance with the Canadian Council on Animal Care.
2.5 Bioluminescence imaging
The viability of biofilm implants was confirmed upon imaging of bioluminescence using the IVIS bioluminescence imaging system (Xenogen Corp) at 24 h post inoculation and again 10 days later, immediately prior to PDT treatment. The change in S. aureus bioluminescence following PDT in vivo was assessed at 24 and 48 h post PDT. For imaging, the animals were subjected to general anaesthesia using 2.5% isoflurane–O2 (Pharmaceutical Partners of Canada Inc., Richmond Hill, ON, Canada) and placed supine into the Xenogen system. Data were acquired as overlaid luminescent and bright field images and specific regions of interest (ROI) of consistent dimension were applied both in vitro or in vivo, and recorded as the absolute total flux (number of photons emitted s−1) or relative light units (RLU) for a 1 min integration time using the Living image™ software 2.0.1. Background subtraction was performed for all images. It was also confirmed previously that the bioluminescence signal produced by exponential growth phase S. aureus incubated at body temperature (∼37 °C) closely correlates to the number of colony forming units (CFU) over the range of 2 × 102–107 CFU.18 Based on mean values of CFU counts plotted against RLU (correlation, r = 0.985), we estimated that RLU of 5 × 10 5 corresponded to ∼1 × 106 CFU (data not shown).
2.6 PDT treatment of S. aureus (Xen29) cells in solution or as biofilm-coated K-wires
2.6.1
In vitro
.
PDT treatment was performed on S. aureus cells in TSBG broth or 1 cm lengths of S. aureus biofilm-laden K-wire. In the former, 25 µL of 104S. aureus cells mL−1 in broth were placed into 35 mm pertri dishes (Nalge Nunc) containing 1 mL of either 0.1 mM phosphate buffered solution (PBS, pH 7.4), 0.1 mM MB or 1 mM ALA in TSBG and incubated for 30 min or 4 h, respectively prior to a single, acute PDT treatment (aPDT; 0, 1, 2, or 5 J cm−2). Immediately following treatment, cells were plated onto TSBG agar plates and the number of surviving colonies (CFU mL−1) determined after 48 h incubation and plotted as a function of PDT light dose (J cm−2). All data points consist of n = 5 repeats. Similarly, for in vitro protocols, biofilm-coated K-wires were immersed into PBS (1 mL) containing either MB (0.1 or 1 mM) or ALA (1 mM). PDT was administered using one of three dose regimens, (1) aPDT, (2) fractionated (fPDT, once daily for 4 days starting day 0) or (3) metronomic (mPDT, continuous for 4 days). All groups consisted of n = 4–6 repeats. aPDT entailed exposing the Xen29 biofilms to either MB (0.1 or 1 mM) or ALA (1 mM) followed 30 min or 4 h later by exposure to light of varying total fluence (1 or 5 J cm−2) at fixed irradiance (21 mW cm−2). Controls were incubated in the presence of photosensitizer only (ALA, 1 mM and MB, 1 mM) without subsequent irradiation. Bioluminescence was recorded 24 and 48 h post aPDT treatment. To be consistent with mPDT conditions, the extracellular drug was not rinsed out prior to irradiation. Fresh TSBG broth was added immediately following treatment. The emitting light source consisted of 9 fan-cooled halogen bulbs configured into a light box with 600 nm long-pass filters providing a stable spectral transmittance (90%) between 600–800 nm. The same light source and drug doses were used for fPDT. However, biofilms were exposed to 1 or 5 J cm−2 daily for 4 days and bioluminescence was recorded once daily, 24 h after each treatment. Again, controls were exposed to photosensitizer only. Previous studies (data not shown) confirmed that the effects on biofilm survival for light only at these fluences were insignificant. A custom designed light source was used for mPDT comprising 12 LED arrays (each of 25 LEDs; 640 ± 40 nm) allowing simultaneous, continuous irradiation of 12 plates inside the incubator. Following 30 min or 4 h incubation with MB (0.1 mM) or ALA (1 mM) respectively, samples were exposed to light (uniformity within each well ca.
±5%) administered as a metronomic regimen delivering 10 J cm−2 d−1 at fluence rate of 0.116 mW cm−2. Bioluminescence of S. aureus biofilms was imaged 24 h after completion of the mPDT treatment. In order to ensure adequate active drug throughout the treatment, fresh MB or ALA was added into the TSBG broth at intervals of 16 h. The light source was powered by a DC supply (BK Precision; 1735A 30V/3A). Controls for mPDT included photosensitizer only (MB, 0.1 mM or ALA, 1 mM) in the absence of light. Fluence rates for mPDT were selected to be 2log10 less than that for aPDT providing 10 J cm−2 equalized over a period of 24 h while that for aPDT was related to the light source and could not be changed. The RLU for biofilms exposed to PDT was expressed as percentage of the signal immediately prior to treatment and plotted as a function of time in days.
2.6.2
In vivo PDT treatment.
Test animals (n = 12 total) received an intraperitoneal (i.p.) injection of ALA (300 mg kg−1 in saline). Animals were, thereafter, kept in dim light conditions for 4 h then anaesthetized (2.5% isoflurane–oxygen) and placed onto a warming blanket. Laser light (635 ± 10 nm) was delivered transcutaneously via an optical fiber (silicon cladded, 200 µm outer diameter) directed towards the bioluminescent area within the tibia. The fiber was mounted with a collimating lens to illuminate an area of 0.78 cm2. The output of the laser was adjusted to deliver fluence of 75 J cm−2 at 250 mW cm−2. Immediately following PDT treatment, animals received a subcutaneous injection of analgesic (buprenorphine, 0.05 mg kg−1) before being returned to their cages. The RLU of S. aureus biofilms in the left and right tibia at 24 and 48 h post PDT were expressed as percentage of the bioluminescence immediately prior to treatment. Colony forming studies had previously confirmed that 48 h was sufficient time post treatment for cell death to occur.
2.7 Statistical analysis
For in vitro experiments all data are plotted with ±1 standard deviation. Significance between untreated and treated biofilms was inferred with 95% confidence using the Student's t-test involving one-tailed distribution and assuming unequal variance for in vitro studies and equal variance in the case of the in vivo data.
3. Results
This study examines the response of S. aureus cells or biofilm to MB or ALA-mediated PDT using bioluminescence as an indicator of viability. It has previously been reported that the bioluminescent strain of S. aureus, Xen29 provides a valid method of non-invasively monitoring the progression of a S. aureus infection in vivo.14,16,17 Using this same strain we now confirm the potential utility of PDT as treatment for S. aureus infections in bone using a rat model of osteomyelitis, similar to that described previously.15,18 Initial in vitro experimentation confirmed the responsivity of S. aureus cells grown in suspension or propagated as biofilms onto lengths of K-wire.
3.1
In vitro
A plot of the relative number of S. aureus cells surviving after aPDT of varying fluence using MB (0.1 mM) or ALA (1 mM) is shown in Fig. 1. The application of light alone had a noticeable, albeit insignificant, effect on cells survival. With the combination of drug and light together, however, a significant ≥4log10 cell kill was achieved at a fluence of 5 J cm−2. The relative responses of S. aureus to MB and ALA were comparable at these different drug concentrations. Higher concentrations of MB (1 mM) did not significantly enhance cell kill in solution (results not shown).
![Graph showing the survival of S. aureus cells following MB [□] (0.1 mM) or ALA [○] (1 mM)-mediated PDT at light doses of 0, 1, 2 or 5 J cm−2. Controls for MB [■] and ALA [●] groups were exposed to light (5 J cm−2) only.](/image/article/2006/PP/b507082a/b507082a-f1.gif) |
| Fig. 1 Graph showing the survival of S. aureus cells following MB [□] (0.1 mM) or ALA [○] (1 mM)-mediated PDT at light doses of 0, 1, 2 or 5 J cm−2. Controls for MB [■] and ALA [●] groups were exposed to light (5 J cm−2) only. | |
Milky coloured biofilms derived from S. aureus cells were clearly visible on K-wires following incubation at 37 °C in TSBG for 3 or more days. The total integrated bioluminescent intensity from each wire was typically in excess of 1.9 × 105 photon s−1, equivalent to approximately 3.8 × 105 CFU wire−1. Bioluminescence was found to reach a maximum after 3–5 days of incubation and thereafter decrease slightly (∼30–40%) and remain somewhat constant for >15 days with daily replenishment with fresh TSBG. A photograph of bioluminescent K-wires shown in Fig. 2 reveals the comparative homogeneity in bioluminescent intensity following 3 days in culture.
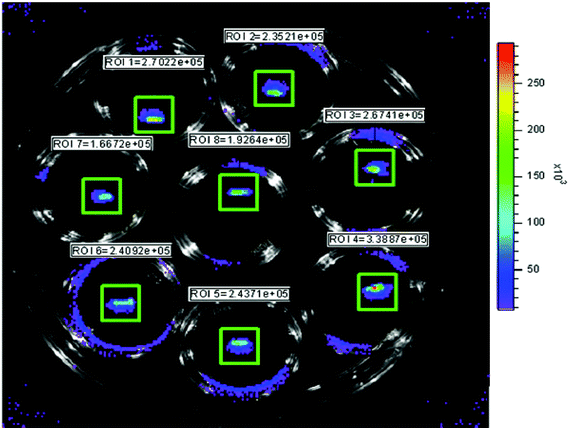 |
| Fig. 2 Photograph of bioluminescent S. aureus-coated K-wires following 3 days in TSBG at 37 °C. The bioluminescence intensity closely correlated to the total CFU wire−1. Upon progression into stationary growth phase, however, bioluminescence decreased and was no longer linear with the number of CFU. | |
Xen29 biofilm-coated K-wires were subjected to 3 distinct regimens of PDT in vitro, namely, aPDT, fPDT or mPDT using MB or ALA. The RLU following PDT or light only (controls) was normalized to a percentage of the initial signal immediately prior to treatment and plotted for each day after (in the case of aPDT and mPDT) or during the treatment (for fPDT). The results are shown in Fig. 3a–c below. A significant decrease in bioluminescence between control and treated samples was evident 1 day after ALA-mediated aPDT with light doses of 1 (p = 0.022) or 5 (p = 0.018) J cm−2 and persisted, albeit less pronounced, at 2 days post treatment (p = 0046). MB appeared to be less effective, with only the combination of 1 mM MB and 5 J cm−2 light dose resulting in a significant decrease in bioluminescence at 1 (p = 0.035) and 2 (p = 0.023) days post treatment. The t-test analysis also revealed that fPDT using either photosensitizer did not result in a significant difference in bioluminescence between control and treated groups, despite the obvious trends in response (see Fig. 3b). The lack of statistical significance is largely a result of the unequal standard deviation between groups, necessitating the use of an unequal variance t-test. The trend in data, however, suggests that the ALA-fPDT provides a sustainable decrease in bioluminescence over the 4 day period. The sustainable decrease in bioluminescence throughout the 4 day regimen suggests that fPDT has potential to provide effective management of S. aureus infections. The mPDT regimen (Fig. 3c) resulted in a significant decrease in bioluminescence for MB (p = 0.026) and ALA-mediated PDT (p = 0.033) after 4 day continuous treatment.
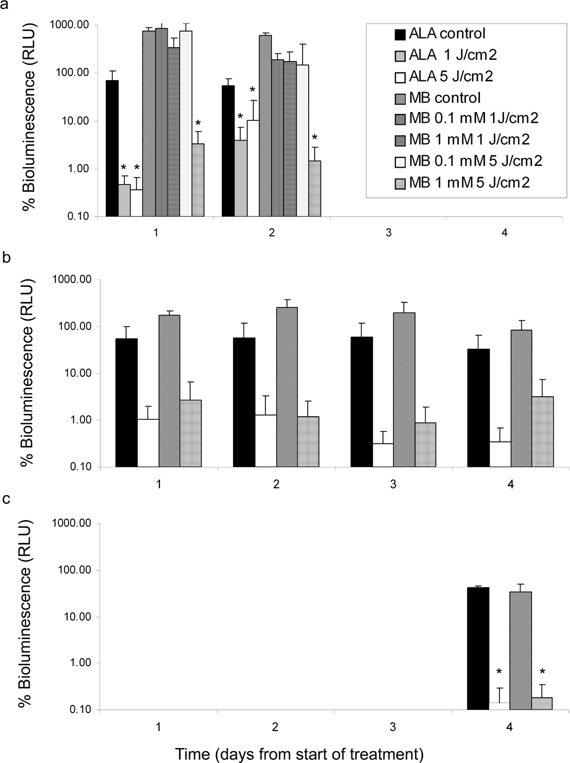 |
| Fig. 3 Histogram plots showing the bioluminescence of S. aureus biofilm-coated K-wires as a function of time in days following 3 distinct treatment regimens; (a) aPDT, (b) fPDT or (c) mPDT. In each instance the RLU following PDT or drug only controls was normalized to the initial signal immediately prior to treatment. Statistical significance was considered when p
≤ 0.05. | |
3.2
In vivo
The successful propagation of viable S. aureus biofilm along lengths of titanium wire and subsequent implantation into the medullary space of rat tibia served as a model to mirror the analogous scenario increasingly found in patients in which titanium implants or prostheses become contaminated with S. aureus, which can result in contiguous spread to the bone and osteomyelitic infection. This study serves to confirm that PDT can be used to treat contaminated implants within bone. 24 h after implantation into the bone, the bioluminescent biofilms coating the wires were clearly evident when imaged using the IVIS (see Fig. 4a). The precise location of wires within the tibia could be visualized using fluoroscopy (Fig. 4b). None of the animals receiving implants displayed signs of discomfort, irregular ambulation or distress. Indeed, animals were typically behaving normally within minutes being taken off anaesthesia. In a number of the animals that had received PDT, the tibia were excised at the end of the experiment and imaged for bioluminescence. The viable bacterial biofilm remnant along the wires could easily be appreciated, an example of which is shown in Fig. 4c. Furthermore, it was consistently observed that the bioluminescence intensity measured from biofilms within the intact living animal was 20–50% less than that measured for the same biofilms upon excision of the tibia, most certainly a consequence of light attenuation by overlying tissues in vivo.
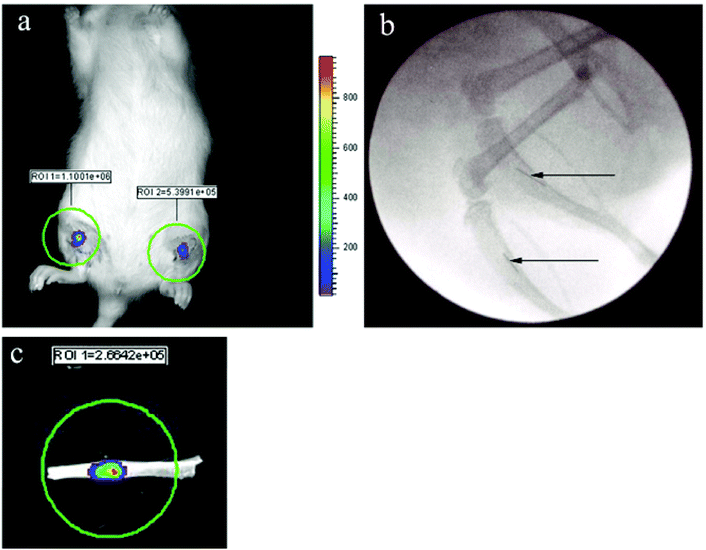 |
| Fig. 4 (a) IVIS image of bioluminescent S. aureus biofilms 24 h post implantation into the right and left tibia of a rat. (b) Fluoroscopy confirmed the precise location of the same biofilm-coated wires within the tibia (see arrows). (c) An excised tibia clearly demonstrates the presence of viable bacterial infection confined to the wire. | |
Fig. 5a and b show the histogram plots of RLU normalized to pre-treatment values (100% values; not shown) for individual implants following different PDT regimens. It is clear that the current PDT doses were not sufficient to eliminate any of the biofilms entirely. In Fig. 5a the right side of treatment animals (n = 5) were exposed to light only (75 J cm−2, in the absence of photosensitizer) while the left sides were not treated. There was no significant difference between the groups at 24 h (p = 0.170) and 48 h (p = 0.405). In order to negate the influence of ALA (300 mg kg−1) alone, a number of animals (n = 7) also received PDT treatment to only their right side implant using 75 J cm−2 light dose (Fig. 5b). A comparison of the relative bioluminescence for ALA only tibia (left side) and the PDT treated tibia revealed a highly significant difference after 24 h (p = 0.0071) however, this difference was not evident after 48 h (p = 0.112). In a separate group of animals (n = 2; data not shown) treatment was applied to both left and right tibia. The recovery of bacteria at 48 h was less evident in this ‘bilateral’ group (see Fig. 6). The significance of this is not clear. These results do, however, indicate that ALA-mediated PDT can decrease the bioluminescence of S. aureus-derived biofilms within bone and since bioluminescence is a reliable indicator of cellular viability, the inference to cell kill can be made. Indeed, the changes in bioluminescence within the treated biofilms are unlikely to reflect changes in cellular metabolism as the same changes would be evident from control biofilms that did not receive PDT. Ultimately, an additional step should be to obtain the number of viable CFU biofilm−1 upon retrieval of biofilms and re-culturing of single cell suspensions.16
![Histograms showing the bioluminescence (% of pre-PDT bioluminescence) of K-wires implanted into the right [■] and left [□] tibia of individual rats (n = 5 group−1) at 24 and 48 h post treatment consisting of either (a) no treatment (left side) and light only (right side; 635 ± 10 nm, 75 J cm−2), (b) PDT treatment using ALA (300 mg kg−1, i.p.) followed 4 h later by light (75 J cm−2) to the right side only.](/image/article/2006/PP/b507082a/b507082a-f5.gif) |
| Fig. 5 Histograms showing the bioluminescence (% of pre-PDT bioluminescence) of K-wires implanted into the right [■] and left [□] tibia of individual rats (n = 5 group−1) at 24 and 48 h post treatment consisting of either (a) no treatment (left side) and light only (right side; 635 ± 10 nm, 75 J cm−2), (b) PDT treatment using ALA (300 mg kg−1, i.p.) followed 4 h later by light (75 J cm−2) to the right side only. | |
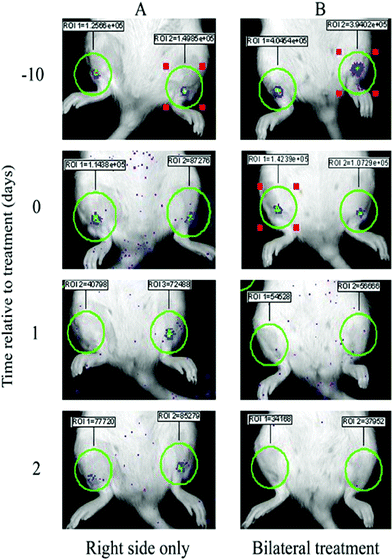 |
| Fig. 6 IVIS images showing examples of bioluminescent S. aureus biofilm-coated K-wires implanted into the intramedullary cavity of rat tibia before (−10 and 0 d) and after (1 and 2 d) ALA-mediated PDT to either the right side only (A) or both sides (B). The bioluminescence intensity (total flux) for each biofilm was measured within ROI of consistent size. | |
IVIS images of implanted biofilms provided clear evidence of the changes in absolute bioluminescent signal before and after PDT. Fig. 6 shows images from 2 rats, one that had received treatment (75 J cm−2 light dose) to the right side only (A) and the other was treated bilaterally again using 75 J cm−2 light dose. A decrease in signal ranging from <9% to >70% is seen when comparing images 24 h post implant and 10 days prior to treatment (day −10), such that on the day of PDT treatment (day 0) biofilms all exhibit similar bioluminescence, all significantly above background levels. It is reasonable to assume that the initial decrease in signal occurs due to the disturbance of the biofilms upon implantation into the tibia. On day 2 a notable recovery in signal is evident from the treated biofilm in rat A (∼70% of day 0 levels) as defined in Fig. 5b.
4. Discussion
This study describes the effects of PDT on S. aureus using two very distinct photosensitizing agents, MB and ALA. S. aureus that had been genetically engineered to bioluminesce were grown either as suspensions in vitro or as adherent biofilms coating pieces of titanium wire and implanted into the tibia of rats. In vitro results confirmed the sensitivity of S. aureus cells to ALA or MB-mediated PDT with ≥4log10 cell kill at 5 J cm−2 light dose and 1 mM and 0.1 mM drug concentrations respectively. No additional increase in cell kill was evident using higher concentrations of MB. Modest light dose regimens were purposely chosen for this study to minimize light only effects which may result due to the fact that some bacterial cells are known to synthesise high levels of endogenous porphyrins, although this appears not to be the case for S. aureus.12 We also wanted to appreciate the extent of cell kill that could be expected when using relatively low level irradiation rather than attempting to define the maximal cell kill attainable using higher doses as described by other investigators.12,13 It is further reasonable to propose that the use of lower fluence opens up the possibility of using light sources other than lasers, such as light emitting diodes (LEDs), an option that will undoubtedly be of use when considering mPDT regimens of light delivery clinically. We have recently introduced mPDT as an alternate dosing regimen using ALA for the treatment of brain tumors with a view to exploiting apoptosis as the primary mode of cell death.19 Using bioluminescence as our primary measure of biofilm vitality the results of this study confirm a significant decrease following mPDT for 4 days. The relative decrease in signal was similar for MB and ALA. The effects using aPDT or fPDT regimens were also substantial although, for the most part not statistically significant, with the exception of ALA which provided significant reduction in bioluminescence using 1 or 5 J cm−2 light dose. The option for oral administration and negligible toxicity identify ALA as an attractive candidate for mPDT and it is conceivable or perhaps highly likely that the application of ALA-mediated mPDT will have an important role to play in the treatment of chronic osteomyeliytis.
Bioluminescence has been described in a number of recent articles as a sensitive, non-invasive indicator of bacterial infections in vivo, whether initiated upon direct inoculation of bacterial suspension18 or as biofilms coating metal implants.14,16,17,20,21 It is important to consider, however, that the precise correlation of RLU as a function of CFU is highly contingent on the growth phase, hence metabolic activity of the cells. Cells of lower metabolic activity display reduced bioluminescence. Temperature must also be optimal ∼37–37.5 °C for the luxABCDE operon. This study describes only the relative changes in bioluminescence without direct inference to CFU, although it is reasonable to assume that, when the changes in treated samples are compared with those of untreated samples, the relative difference is reflective of changes in cell viability. Furthermore, the reduced signal from implanted biofilms in situ as compared to that upon excision of the rat tibia (Fig. 4c) suggest that, despite the comparatively thin layer of muscle and skin covering the anterior tibia, the number of photons exiting the body is diminished. Nevertheless, the results in Fig. 5 and illustrated in Fig. 6 do confirm the action of ALA-mediated PDT delivered transcutaneously to biofilm-coated wires in bone. PDT parameters and modes of delivery for either drug or light have not been optimized in this study and will be the subject of future work. In particular, it is known that S. aureus produce negligible amounts of protoporphyrin IX upon induction by exogenous ALA the predominant metabolite being coproporphyrin with a fluorescence peak of ∼622 nm.13 Fortunately, the spectral range of our light sources, although not specific to coproporphyrin, was sufficient to allow excitation.
The implants described in this study are not intended to instigate osteomyelitic lesions within the rat tibia but serve only to highlight the potential for PDT to treat infection in bone. Planned studies aim to include the treatment of osteomyelitic lesions using ALA-PDT following either aPDT or mPDT regimens. We have previously described the successful treatment of malignant lesions within the vertebrae of rats using interstitial placement of optical fibres,22 an important consideration given the preponderance for vertebral osteomyelitis in adults and the limited options for complete surgical debridement of diffuse infection. The technological advancements in medical imaging, including 3-dimensional cone beam computer tomography,22 will undoubtedly have a major impact on the precision of optical fiber placement and subsequent targeting of PDT to interstitial sites such as bone. Finally, it is of great interest to us to consider that the therapeutic efficacy of PDT as a treatment for osteomyelitis may be further enhanced when administered in conjunction with hyperbaric oxygen therapy23 and this will certainly be addressed in future studies.
5. Conclusions
The increasing number of motor vehicle accidents each year and increasing use of orthopaedic implants as populations age, has resulted in an escalating incidence of osteomyelitis. Unfortunately, the costs associated with long term antibiotic treatments for chronic osteomyelitis are considerable and the need for prolonged hospitalization due to recurrence or protracted follow-ups can be a burden to health-care services. Despite the increasing availability of new antibiotics that can be administered orally in an effort to negate the need for hospitalization, the need for alternate strategies of treatment is paramount, with the ever increasing emergence of antibiotic resistant bacterial strains. Indeed, many of the new antibiotics are more potent but also increase risk of systemic toxicity. The versatility and potency of PDT against many types of microorganism and the lack of resistance associated with repeated use warrant further investigation as an alternative therapy to antibiotics. The results presented here further support the potential use of ALA-mediated PDT to treat microbial infections in bone.
Abbreviations
IVIS, In vivo imaging system; ALA, 5-aminolevulinic acid hydrochloride; PDT, photodynamic therapy; RLU, relative light units; CFU, colony forming units; ROI, region of interest; TSBG, trypticase soy broth supplemented with 0.25% glucose; PBS, phosphate buffered solution; MB, methylene blue; O.D., optical density; S. aureus, Staphylococcus aureus; aPDT, acute PDT; fPDT, fractionated PDT; mPDT, metronomic PDT.
Acknowledgements
The authors wish to acknowledge the invaluable technical assistance of Dr Annie Linn and Dr Cari Whyne for the use of the FluoroScan. This study was supported in part by Photonics Research Ontario. Bioluminescence studies were supported by the National Cancer Institute of Canada.
References
- D. Lew and F. Waldvogel, Osteomyelitis, Lancet, 2004, 364, 369–379 CrossRef CAS.
- C. H. Epps Jr., D. D. Bryant 3rd, M. J. Coles and O. Castro, Osteomyelitis in patients who have sickle-cell disease. Diagnosis and management, J. Bone J. Surg. Am. Vol., 1991, 73, 1281–1294 Search PubMed.
- M. V. Moutsschem, A. J. Scheen and P. J. Lefebvre, Impaired immune responses in diabetes mellitus: analysis of the factors and mechanisms involved. Relevance to the increased susceptibility of diabetic patients to specific infections, Diabete Metab., 1992, 18, 187–201 Search PubMed.
- D. R. Dirschl and L. C. Almekinders, Osteomyelitis. Common causes and treatment recommendations, Drugs, 1993, 45, 29–43 CrossRef CAS.
- K. M. Song and J. F. Sioboda, Acute hematogenous osteomyelitis in children, J. Am. Acad. Orthop. Surg., 2001, 9, 166–175 Search PubMed.
- B. Sinha, P. P. Francois, O. Nusse, M. Foti, O. M. Hartford, P. Vaudaux, T. J. Foster, D. P. Lew, M. Herrmann and K. H. Krause, Fibronectin-binding protein acts as Staphylococcus aureus invasion vis fibronectin bridging to integrin alpha5betral, Cell Microbiol., 1999, 1, 101–117 CrossRef CAS.
- M. C. Hudson, W. K. Ramp, N. C. Nicholson, A. S. Williams and M. T. Nousiainen, Internalization of Staphylococcus aureus by cultured osteoblasts, Microb. Pathol., 1995, 19, 409–419 Search PubMed.
- D. N. Fisman, D. T. Reilly, A. W. Karchmer and S. J. Goldie, Clinical effectiveness and cost-effectiveness of 2 management strategies for infected total hip arthroplasty in the elderly, Clin. Infect. Dis., 2001, 32, 419–430 CrossRef CAS.
- A. H. Kaim, T. Gross and G. K. von Schulthess, Imaging of chronic posttraumatic osteomyelitis, Eur. Radiol., 2002, 12, 1193–1202 CrossRef.
- R. H. Gold, R. A. Hawkins and R. D. Katz, Bacterial osteomyelitis: findings on plain radiography, CT, MR, and scintigraphy, AJR, Am. J. Roentgenol, 1991, 157, 365–370 Search PubMed.
-
S. M. Finegold, Intestinal microbial changes and disease as a result of antimicrobial use, Ped. Infect. Disease J., 1986, 5, Suppl. S88–90 Search PubMed.
- M. Usacheva, M. Teichert and M. Biel, Comparison of the methylene blue and toluidine blue photobactericidal efficacy agains Gram-positive and Gram-negative microorganisms, Lasers Surg. Med., 2001, 29, 165–173 CrossRef CAS.
- N. Yeshayahu, S. Mali, S. Einav and M. Avi, ALA induced photodynamic effects on Gram positive and negative bacteria, Photochem. Photobiol. Sci., 2004, 3, 430–435 RSC.
- K. Francis, D. Joh, C. Bellinger-Kawahara, M. Hawkinson, T. Purchio and P. Contag, Monitoring bioluminescent Staphylococcus aureus infections in living mice using a novel luxABCDE construct, Infect. Immunol., 2000, 68, 3594–3600 Search PubMed.
- J. P. Rissing, T. B. Buxton, J. Fisher, R. Harris and R. K. Shockley, Arachidonic acid facilitates experimental chronic osteomyelitis in rats, Infect. Immunol., 1985, 49, 141–144 Search PubMed.
- J. Kadurugamuwa, L. Sin, J. Yu, K. Francis, R. Kimura, T. Puchio and P. Contag, Rapid direct method for monitoring antibiotics in a mouse model of bacterial biofilm infection, Antimicrob. Agents Chemother., 2003, 47, 3130–3137 CrossRef CAS.
- N. Kuklin, G. Pancari, T. Tobery, L. Cope, J. Jackson, C. Gill, K. Overbye, K. Francis, J. Yu, D. Montgomery, A. Anderson, W. McClements and K. Jansen, Real-time monitoring of bacterial infection in vivo: development of bioluminescent Staphylococcal foreign-body and deep-thigh-wound mouse infection models, Antimicrob. Agents Chemother., 2003, 47, 2740–2748 CrossRef CAS.
- E. Gracia, A. Lacleriga, M. Monzon, J. Leiva, C. Oteiza and B. Amorena, Application of a rat osteomyelitis model to compare in vivo and in vitro the antibiotic efficacy against bacteria with high capacity to form biofilms, J. Surg. Res., 1998, 79, 146–153 CrossRef CAS.
- S. K. Bisland, L. Lilge, A. Lin, R. Rusnov and B. C. Wilson, Metronomic photodynamic therapy as a new paradigm for photodynamic therapy: rationale and preclinical evaluation of technical feasibility for treating malignant brain tumors, Photochem. Photobiol., 2004, 80, 22–30 CrossRef CAS.
- J. Kadurugamuwa, L. Sin, E. Albert, J. Yu, K. Francis, M. Deboer, M. Rubin, C. Bellinger-Kawahara, T. Parr and P. Contag, Direct continuous method for monitoring biofilm infection in a mouse model, Infect. Immunol., 2003, 71, 882–890 Search PubMed.
- E. Gracia, A. Fernandez, P. Conchello, J. Alabart, M. Perez and B. Amorena,
In vitro development of Staphylococcus aureus biofilms using slime-producing variants and ATP-bioluminescence for automated bacterial quantification, Luminescence, 1999, 14, 23–31 CrossRef CAS.
- S. Burch, S. K. Bisland, J. Siewerdsen, A. Bogaards, D. Moseley, A. Yee, J. Finkelstein and B. C. Wilson, Photodynamic therapy for the treatment of metastatic lesions in bone: studies in rat and porcine models, Proc. SPIE-Int. Soc. Opt. Eng., 2004, 5315, 76–87 CAS.
- J. Wang, F. Li, J. H. Calhoun and T. J. Mader, The role and effectiveness of adjunctive hyperbaric oxygen therapy in the management of musculoskeletal disorders, J. Postgr. Med. (Bombay), 2002, 48, 226–231 Search PubMed.
|
This journal is © The Royal Society of Chemistry and Owner Societies 2006 |
Click here to see how this site uses Cookies. View our privacy policy here.