DOI:
10.1039/B516903H
(Focus)
Lab Chip, 2006,
6, 19-23
Nanotechnology for membranes, filters and sieves
A series of mini-reviews covering new trends in fundamental and applied research, and potential applications of miniaturised technologies
First published on 6th December 2005
In this issue of Lab on a Chip we are introducing a new regular series of mini-reviews that are tightly focused on recent research. These mini-reviews will cover trends in recent research, focus on potential new areas of research or even potential applications. The aim is to inform the reader about such recent trends, bring ‘forgotten’ papers back into the spotlight and perhaps most importantly, to provide a perspective of a particular subject as well as a critical evaluation of it that may help researchers to plan future research. Six mini-reviews will appear during the year.
Introduction
This mini-review is dedicated to the use of nanotechnology for membranes, filters and sieves. With the advent of nanotechnology researchers have acquired an unprecedented freedom to sculpt device geometry almost down to the molecular scale. Such structures can now replace the gels, membranes and sieves of random pore structure that are commonly used in such separations. In this mini-review we want to highlight the impact that this development has had in the area of separation by filtering and sieving, where exciting developments are taking place. To do this we will first present the basic phenomena that determine separation in these devices, together with some historical background. Subsequently we will look at the micro- and nanomachined membranes, filters and sieves that have been manufactured in the past few years, and highlight advances resulting from the use of nanotechnology. In particular, the ability of nanotechnology to produce spatially anisotropic sieving structures suitable for continuous flow operation is seen as a new and exciting development. The invention of structures that sculpt hydrodynamic flow lines in order to perform continuous filtering is another important development. In general continuous flow operation is desirable in nanoscale systems since sampling (either of a detection signal or of the separated substances) can be time-integrated, thus improving the detection limit.
Basic sieving phenomena: steric and entropic effects
Molecules can adopt a certain number of different spatial orientations and conformations. Membranes and pores on the other hand have a certain open space through which molecules can pass. Molecules will not be able to pass through the spaces or pores when there is not a single orientation and/or conformation allowing this passage. If passage is possible, there will be allowed and forbidden (overlapping) molecular conformations (see Fig. 1).1 The decrease in orientational and conformational freedom resulting from these forbidden states causes a decrease in entropy for the molecule inside the membrane, and hence a decrease in the particle distribution coefficient between pore and adjacent solution, decreasing molecular permeation. Theory for this type of sieving was developed for rigid particles by Ogston (Ogston sieving) and Giddings.2,3 Long polymers like DNA can adopt many conformations in a process of self-diffusion, and for such polymers the decrease in entropy when moving into confined spaces can be very large. An added level of complexity is still introduced by applied force fields, which can change the molecular shape. Polyelectrolytes like DNA will be elongated by electrical fields, and all (charged and non-charged) long chain polymers by flow fields.
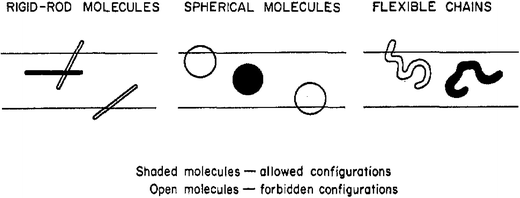 |
| Fig. 1 Illustration of allowed and forbidden configurations in pore space. Reproduced with permission from ref. 3. | |
Basic sieving phenomena: charge related permeation, ion permselectivity
The theory for the influence of the membrane fixed charge on its permeability for charged substances was first developed in the 1930s for membranes with homogeneously distributed fixed charge (the Teorell–Meyer–Sievers or TMS theory).4,5 The membranes will have an equilibrium potential difference with respect to the solution, coions are excluded from it according to Boltzmann's law and consequently show a low permeation flux and counterions are enriched and show a high flux (permselectivity). According to the Boltzmann distribution molecular permeation depends exponentially on the valency of the permeating molecules. Membrane potential and permselectivity increases with decreasing ionic strength, the dependence becoming exponential at high fixed charge concentrations. The charge-based filtering that results is encountered in nature for example in the kidney where the negatively charged albumin is prevented from passing from the blood to the primary urine by the negatively charged pores in the basal membrane.6 The TMS theory applies only to membranes with homogeneously distributed fixed charge, but many membranes derive their permeability from nanopores. Theories for the ion permselectivity of charged capillaries were therefore derived as well, e.g., the space charge model.7,8 The theory for homogeneous membranes and for nanopores yield comparable results when the pore radius is small with respect to the Debye length.8
Permselectivity could be investigated in more detail when materials with well-controlled nanopore size became available. Thus diffusion rates of small molecules through track-etched membranes were investigated as a function of ionic strength.9 Comparable experiments showed that the permeation of charged polystyrene spheres depended strongly on the ionic strength.10 Also for protein transport through artificial membranes electrical effects were shown to be highly important.11 When it was discovered that the pores of track-etch membranes could be plated with gold to leave gold-clad nanopores down to 1 nm diameter, charge-based transport selectivity could be demonstrated in these pores.12,13 The gold nanopore system was later used as the basis to construct pH-dependent water permeating membranes.14 Even more recently micromachined nanochannels became available for experiments, and ionic transport and semi-permeability were demonstrated anew.15 The charge-based transport selectivity can be modulated in a nanochannel by capacitive means to change the surface charge (‘fluidic transistor’),16 a principle first demonstrated in 1992.17 The advantage of measuring with micromachined nanochannels is that geometry and also connectivity can be precisely designed and manufactured and that individual nanochannels can be accessed for experimentation and analysis.18
Sieving/filtering strategies
Fig. 2 shows different strategies that can be used in filters and sieves to employ the forces of electric, steric and entropic origin listed above. These forces together with the hydrodynamic flow field will determine particle movement in sieves. If a particle is excluded from a slower moving region of the liquid, for example from the slowly moving wall region in parabolic flow, this will result in an increased transport rate for the larger particles. This process is called hydrodynamic chromatography (HDC) and is shown in Fig. 2A. If a particle is excluded from areas where the liquid is stagnant, its transport rate in the direction of fluid flow also increases. This occurs in size exclusion chromatography (SEC) and is shown in Fig. 2B. Fig. 2B is based on a paper by Stegeman et al., who showed that hydrodynamic chromatography will often be accompanied by size exclusion chromatography.19 The concept of HDC stems from DiMarzio, who also discussed its relation to SEC.20 Both for HDC and SEC diffusional equilibration of the particles is necessary across the flow structure, which inherently limits the operation speed. This is not the case for the two examples of anisotropic structures shown in Fig. 2C and D. Fig. 2C shows the quite familiar situation of a (membrane) filter. Here some classes of particles are totally excluded from a certain pathway, which not only changes their transport rate but also their transport direction. Finally, Fig. 2D shows a structure that has only become possible with modern machining technology namely a spatially anisotropic sieving structure. In the structure shown particles will have a preferential direction of movement which depends on the main flow lines they follow. We will term this procedure flow line sieving and it will be treated in more detail in the following section.
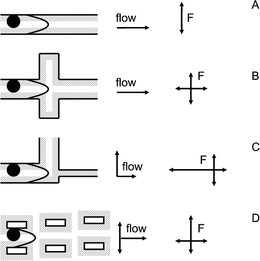 |
| Fig. 2 Four different sieving strategies. A. Hydrodynamic chromatography; B. Size exclusion chromatography; C. Filtering; D. Flow-line sieving. Indicated as shaded areas are the zones from which the particle is excluded by steric or electrical forces F. Also indicated are the flow directions in the sieving structure, and the directions of the forces exerted on the particle. | |
As already mentioned, applied fields can change molecular conformation, complicating sieving behaviour. This has been very well investigated for the electrophoretic separation of DNA in gel matrices. In DNA gel-based sieving at least four regimes are distinguished.21,22 Ogston sieving (see previous section) occurs when the radius of gyration of the DNA molecule is smaller than the average pore size. When the radius of gyration is approximately equal to the average pore size but a large variance exists in pore size, DNA can get separated by entropic trapping in the larger pores. If, finally, the pore size is much smaller than the radius of gyration, the DNA will move in a snake-like conformation through the gel, a movement called reptation. Excellent reviews on DNA separation were given by Viovy and Slater et al.22–24
Nanomachined devices—new strategies
Continuous flow operation is highly desirable especially in nanomachined devices because of the low sample quantities. Operation in continuous flow will enable the operator to integrate the signal over time or to collect the sample over time thus decreasing the detection limit. To perform sieve-type separations in continuous flow in a 2-D matrix, some net force must be generated which is orthogonal to the applied flow field. Fig. 2C and D show two possible approaches. Fig. 2C shows the classical way, namely blocking the passage of certain particles from one flow direction by the filter and transporting them further via a side channel. This principle, however, will provide fractionation in only two fractions. By micro- and nanomachining an anisotropic pore space as shown in Fig. 2D another approach becomes possible which allows a much further fractionation. In classical membranes and gels the pore space is randomly distributed and isotropic. As a result all forces that are at right angles with the flow field are averaged out so that no net orthogonal separating force remains. This limits us to 1-D separations, where a sample plug is separated into different zones along the separation axis. Recently a number of devices have been demonstrated that possess an anisotropic sieving structure and are operated in continuous flow. The first such device was demonstrated by Huang for the separation of polystyrene beads and DNA.25 It employs multiple lines of columns at micrometer spacing, with each column line slightly shifted with respect to the previous one in a direction perpendicular to the flow direction, thus introducing anisotropy (Fig. 3A). It is very feasible that future devices will explore smaller dimensions, separating smaller particles or even ions. Huang et al. termed this effect deterministic lateral displacement and we will group it under flow line sieving. The separation is based on the inhomogeneous particle distribution generated by hydrodynamic effects (e.g., large particles are concentrated in the channel centre). When the channel then downstream is split into branches, each branch will carry the molecules/particles present in its feeding streamlines. A significant advantage of this approach is that diffusion across the flow lines is not necessary for the operation. The devices can therefore be used at the highest flow velocity possible, which will benefit resolution since it will reduce lateral diffusion. Flow line sieving also occurs in the pinched flow device introduced by Yamada et al. and schematically shown in Fig. 3B.26 As in the previous case operation of this sieve improves with flow speed since diffusion will only diminish resolution. Using the same principle of flow-line sieving, Yamada has also manufactured a device that combines particle separation with concentration.27 The deterministic lateral displacement principle has also been applied for preparative purposes, namely for the separation of blood cells.28
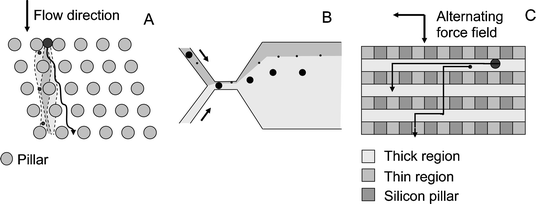 |
| Fig. 3 A. Flow line sieving in the anisotropic filter structures employed by Huang et al.25 Pillar gaps measure 1.6 μm. B. Flow line sieving in the pinched flow fractionation device by Yamada et al.26 C. Sieving in the anisotropic sieve of Fu and Han.29 The channel height above the thick region is 300 nm and above the thin region 55 nm. All three structures allow 2-D continuous flow operation. | |
The benefit of an anisotropic sieving matrix for continuous flow separation was also recently demonstrated by Fu and Han and applied to SDS–protein complexes and DNA.29 Fu and Han use a cleverly designed device (Fig. 3C) since instead of creating the pore space in the horizontal direction as Huang et al., who employed the space between micromachined columns as pores and introduced anisotropy by laterally shifting the column arrays, Fu and Han created the pore space in the vertical direction by etching very shallow parallel nanochannels and introduced anisotropy by providing orthogonal sub-micron channels. The advantage of their approach is that smaller pore sizes can be much more easily machined in the vertical plane, e.g., by etching. It would be possible to combine different separation techniques along the orthogonal axes by varying pore size and wall charge as well as the applied force field. A further possibility is to apply orthogonal fields as in the different types of field flow fractionation.1 Finally the small size of the sieving structures makes electrical effects more important. If the electrical wall potential can be actively controlled as already shown for nanochannels, a new degree of freedom would be introduced.16 We consider the areas of flow-line filtering and of continuous flow sieving with anisotropic structures as some of the most exciting and promising areas for development over the coming years.
Of special interest as a new strategy is also the ionic separation recently demonstrated by Pennathur and Santiago, which can be regarded as a combination of hydrodynamic ion chromatography (or electric field field flow fractionation) and electrophoresis, dubbed EKSIV.30,31 It can be performed in nanochannels when the electrical double layer thickness is in the order of the channel height (or radius), and is based on the parabolic flow profile of electroosmotic flow in nanochannels combined with the different distance from the wall that ions of different charges will adopt due to the Boltzmann distribution.
For the separation of long DNA (40–170 kbp) the new method of entropic recoil separation has been introduced some years ago.32 It employs pillar arrays or long narrow channels that form entropic barriers for DNA permeation. When DNA enters these structures due to an applied E-field and the field is subsequently switched off, it will recoil. The permeation resulting from an alternating applied field proves to be DNA-size dependent.
Nanomachined devices—better control in classical sieving strategies
Micro- and nanomachining has often been used in past years to allow better control over sieve geometry and to tailor the matrix to the separation at hand. This has especially been the case for DNA electrophoresis. As mentioned above, gel-based DNA separation can operate in a number of regimes. Regular micro- and nanochannels with sieve-type structures have been demonstrated for separation in the reptation and entropic trap regime. For the reptation regime Kaji et al. recently pushed the manufacturing limits by constructing an array with 100 nm columns, separating 1–40 kbp DNA using DC applied fields.33 Of special interest for separation by reptation are the magnetic self-assembling sieves, which have been applied to 15–50 kbp samples.34 The entropic trap devices use a sequence of deep and shallow micromachined cavities.35 As an alternative for pulsed field gel electrophoresis Huang et al. designed a chip with regularly spaced microcolumns in which long (60–200 kb) DNA can be separated about 1000 times faster than in conventional pulsed field gel electrophoresis and in continuous flow.36
A micromachined device for HDC (Fig. 2A) has been reported by Chmela et al., employing a 1 μm high and 10 μm wide channel and pressure-driven flow.37 It was applied for the separation of dextran and polystyrene particles. A problem encountered in the separation was peak broadening across the width of the channel. This problem probably is quite generic for channels of large aspect ratio, since it is quite difficult to produce channels of exactly constant height. Another inherent problem of rectangular channels is that the flow profile at the side walls will always be different, causing peak broadening. A solution would be to produce cylindrical nanochannels, but the sample quantity would be exceptionally low and detection problems would almost certainly result. A design employing 2-D continuous flow for HDC separations could possibly solve this problem.
Size exclusion chromatography of DNA fragments has been demonstrated on chip by Sano et al. using a 1 μm high and 80 μm wide channel with porous alumina at the bottom (pore radius 80 nm).38 As in the above case, the separation suffered from band broadening, the cause of which was not analyzed by the authors. Porous silicon has also been investigated for SEC application.39
As shown in Fig. 2C, an average net force can be exerted by the spatial anisotropy offered by a filter. Here continuous flow operation is possible since side channels can continuously carry away excluded matter. The first nanofilter with screen design and 50 nm pores was developed by Stemme and Kittilsland in 1988.40 A micromachined sieve of simpler design (a thin flat plate with nanomachined through-holes) was developed in 1997 by van Rijn et al.41 This design has successfully been commercialized and is in use for filtration in beer production.42 Recently pore sizes down to 10 nm have been produced.43 Advantages of this design are the high flow rate applicable and the low fouling rate. It can be envisaged for the future that stacks of membranes with different pore size will enable continuous-flow fractionation of macromolecules. Instead of flat-plate nanosieves a nanochannel that decreases in height, stepwise,44 could also be used for flow-through size fractionation, if the fractionated particles were transported out of the structure via side channels.
Conclusion
There are exciting developments in the field of filtering and sieving due to the advent of micro- and nanotechnology. Sieving matrices can be machined with spatial anisotropy, enabling continuous flow separation. Continuous flow operation is also possible using the new method of flow line sieving, under which name we grouped together the processes of pinched flow fractionation and deterministic lateral displacement. This continuous flow operation is important for analysis using nanostructures because of the small volumes concerned. It also is a promising technique for preparative separations, e.g., of blood cells. In 2-D matrices sieving can be combined with other separation processes along the orthogonal axis to tune and improve separation. The size limits of the smallest structures are continuously pushed and at present they approach 50 nm for 2-D sieving structures and 10 nm for 1-D nanosieves. In such small structures electrical effects are as important as steric effects, and offer a new source of tunability, especially if the electrical potential can be actively controlled as already demonstrated for single nanochannels.
Jan C. T. Eijkel
Albert van den Berg
MESA+ Research Institute, Twente
References
-
J. C. Giddings, Unified Separation Science, John Wiley and Sons, New York, 1991 Search PubMed.
- A. G. Ogston, Trans. Faraday Soc., 1958, 54, 1754 RSC.
- J. C. Giddings, E. Kucera, C. P. Russell and M. N. Myers, J. Phys. Chem., 1968, 72, 4397 CrossRef CAS.
- T. Teorell, Proc. Soc. Exp. Biol. and Med., 1935, 33, 282 Search PubMed; T. Teorell, Proc. Natl. Acad. Sci., 1935, 21, 152 CAS.
- K. H. Meyer and J.-F. Sievers, Helv. Chim. Acta, 1936, 19, 649 CAS.
- L. Bent-Hansen, B. Feldt-Rasmussen, A. Kverneland and T. Deckert, Diabetologia, 1993, 36, 361 CrossRef CAS.
- R. J. Gross and J. F. Osterle, J. Chem. Phys., 1968, 49, 228 CrossRef CAS.
- H. J. M. Hijnen, J. van Daalen and J. A. M. Smit, J. Colloid Interface Sci., 1985, 107, 525 CrossRef CAS.
- R. E. Beck and J. S. Schultz, Biochim. Biophys. Acta, 1972, 255, 273 CAS.
- D. M. Malone and J. L. Anderson, Chem. Eng. Sci., 1978, 33, 1429 CrossRef CAS.
- N. S. Pujar and A. L. Zydney, Ind. Eng. Chem. Res., 1994, 33, 2473 CrossRef CAS.
- M. Nishizawa, V. P. Menon and C. R. Martin, Science, 1995, 268, 700 CrossRef CAS.
- K. B. Jirage, J. C. Hulteen and C. R. Martin, Science, 1997, 278, 655 CrossRef CAS.
- Y. Ito, Y. S. Park and Y. Imanishi, Langmuir, 2000, 16, 5376 CrossRef CAS.
- Q. S. Pu, J. S. Yun, H. Temkin and S. R. Liu, Nano Lett., 2004, 4, 1099 CrossRef CAS.
- R. Karnik, R. Fan, M. Yue, D. Li, P. Yang and A. Majumdar, Nano Lett., 2005, 5, 943 CrossRef CAS.
- S. A. Gajar and M. W. Geis, J. Electrochem. Soc., 1992, 139, 2833 CAS.
- J. C. T. Eijkel and A. van den Berg, Microfluid Nanofluid, 2005, 1, 249 Search PubMed.
- G. Stegeman, J. C. Kraak and H. Poppe, J. Chromatogr., 1991, 550, 721 CrossRef CAS.
- E. A. DiMarzio and C. M. Guttman, Macromolecules, 1970, 3, 131 CrossRef CAS.
- J. Rousseau, G. Drouin and G. W. Slater, Phys. Rev. Lett., 1997, 79, 1945 CrossRef CAS.
- J.-L. Viovy, Rev. Mod. Phys., 2000, 72, 813 CrossRef CAS.
- G. W. Slater, S. Guillouzic, M. G. Gauthier, J.-F. Mercier, M. Kenward, L. C. McCormick and F. Tessier, Electrophoresis, 2002, 23, 3791 CrossRef CAS.
- G. W. Slater, M. Kenward, L. C. McCormick and M. G. Gauthier, Curr. Opin. Biotechnol., 2003, 14, 58 CrossRef CAS.
- L. R. Huang, E. C. Cox, R. H. Austin and J. C. Sturm, Science, 2004, 304, 987 CrossRef CAS.
- M. Yamada, M. Nakashima and M. Seki, Anal. Chem., 2004, 76, 5465 CrossRef CAS.
-
M. Yamada and M. Seki, in Proceedings of Micro Total Analysis Systems 2005, Kluwer Academic Publishers, Dordrecht, 2005, p. 1528 Search PubMed.
-
S. Zheng, Y.-C. Tai and H. L. Kasdan, in Proceedings Micro Total Analysis Systems 2005, Kluwer Academic Publishers, Dordrecht, 2005, p. 385 Search PubMed.
-
J. Fu and J. Han, in Proceedings of Micro Total Analysis Systems 2005, Kluwer Academic Publishers, Dordrecht, 2005, p. 1531 Search PubMed.
- S. Pennathur and J. G. Santiago, Anal. Chem., 2005, 77, 6782 CrossRef CAS.
- S. Pennathur and J. G. Santiago, Anal. Chem., 2005, 77, 6772 CrossRef CAS.
- M. Cabodi, S. W. P. Turner and H. G. Craighead, Anal. Chem., 2002, 74, 5169 CrossRef CAS.
- N. Kaji, Y. Tezuka, Y. Takamura, M. Ueda, T. Nishimoto, H. Nakanishi, Y. Horiike and Y. Baba, Anal. Chem., 2004, 76, 15 CrossRef CAS.
- P. S. Doyle, J. Bibette, A. Bancaud and J.-L. Viovy, Science, 2002, 295, 2237 CrossRef CAS.
- J. Han, S. W. Turner and H. G. Craighead, Phys. Rev. Lett., 1999, 83, 1688 CrossRef CAS.
- L. R. Huang, J. O. Tegenfeldt, J. J. Kraeft, J. C. Sturm, R. H. Austin and E. C. Cox, Nat. Biotechnol., 2002, 20, 1048 CrossRef CAS.
- E. Chmela, R. Tijssen, M. T. Blom, J. G. E. Gardeniers and A. Van den Berg, Anal. Chem., 2002, 74, 3470 CrossRef.
- T. Sano, N. Iguchi, K. Iida, T. Sakamoto, M. Baba and H. Kawaura, Appl. Phys. Lett., 2003, 83, 4438 CrossRef CAS.
- B. E. Collins, K.-P. S. Dancil, G. Abbi and M. J. Sailor, Adv. Funct. Mater., 2002, 12, 187 CrossRef CAS.
- G. Stemme and G. Kittilsland, Appl. Phys. Lett., 1988, 53, 1566 CrossRef CAS.
- C. van Rijn, M. van der Wekken, W. Nijdam and M. Elwenspoek, J. Microelectromech. Syst., 1997, 6, 48 CrossRef CAS.
- S. Kuiper, C. van Rijn a, W. Nijdam, O. Raspe, H. van Wolferen G. Krijnen and M. Elwenspoek, J. Membr. Sci., 2002, 196, 159 CrossRef CAS.
- H. D. Tong, H. V. Jansen, V. J. Gadgil, C. G. Bostan, E. Berenschot, C. J. M. van Rijn and M. Elwenspoek, Nano Lett., 2004, 4, 283 CrossRef CAS.
- S. Vankrunkelsven, D. Clicq, K. Pappaert, W. Ranson, C. De Tandt, H. Ottevaere, H. Thienpont, G. V. Baron and G. Desmet, Electrophoresis, 2004, 25, 1714 CrossRef CAS.
|
This journal is © The Royal Society of Chemistry 2006 |