Received
7th July 2003
, Accepted 17th September 2003
First published on 17th October 2003
Abstract
The absorption of two photons by a concentrated assembly of identical chromophores can lead to formation of a biexciton. This work concerns the transfer of biexcitonic energy from a ring to a central donor, with a particular focus on rates and geometric effects. Quantum amplitudes are expressed in terms of orientation factors with a clear physical significance and detailed calculations are performed on an idealised, three-fold symmetric photosystem—a structural motif common in a variety of natural and artificial light-harvesting systems.
Introduction
Concentrated assemblies of chromophore centres in macromolecular superstructures offer ideal media for the absorption of light—a principle that applies to both solar and laser radiation. In most natural photosynthetic systems, arrays of chlorophyll (or a similar light-sensitive organic molecule) adopt this role, supplemented by ancillary pigments such as carotenoids. It is known that the organisation of harvesting chromophores differs markedly in the chloroplasts of plants and bacteria. Specifically, bacterial peripheral light-harvesting complexes (LH2)1–3 and core antenna (LH1)4,5 form a highly symmetrical, ordered chromophore environment; conversely, the plant light-harvesting complex (LHCII)6 and Photosystem I7 are more disordered in character. Another chromophore-rich environment is offered by man-made dendrimers. Within these systems, self-assembled chromophore sub-units assume highly symmetrical configurations, operationally comparable to those observed in LH1 and LH2. Many different forms of light-sensitive building blocks have been used in dendrimer construction, yielding macromolecules with interesting and diverse photophysical properties;8–15 in fact, traits of natural light-harvesting antenna systems themselves have been identified and studied.16–18 Finally, multichromophore arrays, usually comprising photoactive porphyrin building blocks coupled to a quenching core, have been specifically engineered for the study of novel photophysical processes achievable with high powered laser sources.19–21 In this area, numerous biomimetic analogues of light-harvesting centres have been synthesised and scrutinised.22–25
Following initial photoabsorption by any one chromophore in any of the above systems, subsequent migration of the excitation is governed by the photophysics of resonance energy transfer (RET).26 This phenomenon is manifested in so-called ‘energy hopping’ and has itself been the focus of many studies in the photosynthetic unit,27–31 dendrimers15,32–34 and multichromophore arrays.35–38 Although the quantum mechanical description of RET is well documented,39–45 some aspects of the theory are still under development.46 The type of energy migration under study in this work occurs between a ring of pre-excited donor species and an amenable acceptor situated beyond wavefunction overlap; the overall structure is not conducive to any electron exchange mechanism which might otherwise dominate over shorter transfer distances.47,48
New features arise in simple transfer descriptions when the absorbers are part of a highly symmetrical environment. Here, excitations delocalised within the set of electronically coupled donor chromophores are characterised in an exciton.49–51 Correspondingly, the excitation can no longer be thought of as localised on a single donor. The energy manifested in the exciton can be transferred to a suitable acceptor, as in RET, and this migration remains an interaction essentially described by the equations of RET. Here though, the single-donor excitation is replaced by an excitonic formulation. Rudimentary excitonic behaviour—arising when photons are absorbed singly—is well documented and significant in both photosynthetic systems52–55 and dendrimers.56–58
The purpose of this paper is an investigation into the nature of double-photon excitation within such arrays. When two excitations are present at different donor centres, the conditions are favourable for the formation of a biexciton. Theory describing the physics of conventional resonance energy transfer is inadequate, as we now have, within the exciton-forming group, two excited donor species instead of one. In non-symmetrical environments, where exciton formation is forbidden because of a lack of degeneracy, investigations into the photophysics of energy transfer from dual donors to a single acceptor have already proved fruitful.59–61 A quantum electrodynamic (QED) investigation into this phenomenon—termed three-centre energy transfer (3CET) or energy pooling—has identified three mechanisms by which transfer is achieved, one so-called cooperative and two accretive pathways (vide infra). The preliminary work was adapted to study the effect of a third body upon RET, essentially offering an explanation for the behaviour of spacer units in bi- and multichromophore arrays, and also in dendrimers.62 Here, we use fundamental molecular QED theory to describe energy transfer from a biexciton, highlighting features which distinguish its particular physics from those of the one-photon analogue.
Biexciton formation and decay
As in the simpler, single-photon exciton case examined previously,63 our model light-harvesting system is envisaged as a dendrimer or other multichromophore array, comprising three identical donor chromophores, A, B and C, potentially coupled to an acceptor species, D. The acceptor may or may not be chemically identical to the donors; however, a chemically distinct acceptor is advantageous when discounting competing processes. The overall system is assumed to exhibit three-fold symmetry—a symmetry element present in bacterial LH22 and various dendrimeric molecules. As such, we assume that each donor sits at the corner of an equilateral triangle with the acceptor in the middle (see the following section for the architecture). All system components are in the electronic ground state prior to photoexcitation. Two photons of approximately sufficient energy (hν) to excite one of the donor species are then absorbed at different sites, forming the biexciton. The probability of achieving the necessary condition for biexciton formation is determined by the laser flux and both the absorption cross-section, σ, and the excited-state lifetime of the donor, τ. Generally, with N donors and a laser delivering an irradiance I, the relative efficiency of biexciton formation is quantified by the ratio NIστ/hν. With a very modest pulsed laser irradiance of 1012 W cm−2, an absorption cross-section of 10−18 cm2 and a lifetime of 100 fs, a biexciton formation efficiency of about 10% can be expected; obviously, the exact figure is sensitive to the details of the particular system.
The features of the biexcitonic quasi-particle are consistent with the system symmetry and relate to the uncertainty in excitation localisation. The excitation energy is essentially encompassed by a superposition of chromophore states. It is this which constitutes the initial matter state for subsequent energy transfer, as compared to the localised excited donor state in conventional RET. We may express the overall photophysics of biexciton formation and transfer through the non-chemical equation
|  | (1) |
where superscripts indicate the state in which species reside (0, ground state; *, group excited state;
u, single-species excited state). In
eqn. 1, the biexciton is represented by (A + B + C)
2* and the 2* indicates that two, indeterminate donors are excited (results for single-
photon excitation processes have been reported elsewhere
64,65). Note that the process of exciton formation need not be immediate; it may be associated with intramolecular relaxation following photoabsorption.
Fig. 1 schematically illustrates a simplified energy level scheme for biexciton formation and
resonance energy transfer. Note that a manifold of vibrational levels (not shown) is associated with each electronic state.
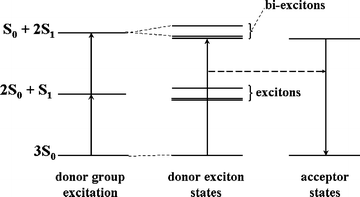 |
| Fig. 1 Schematic energy level diagram for biexciton energy transfer. On the left are combined energy levels for the group of three donors. Two undergo an S0
→ S1 transition, resulting in formation of a biexciton state (which is the initial state for energy transfer) followed by transfer of the net energy to an acceptor. For simplicity, sharp levels without linewidth are depicted. | |
In many photosystems, biexcitonic energy transfer can compete directly with other possible relaxation processes.66 Those manifested at the exciton creation stage of eqn. 1 jeopardise the formation of (A + B + C)2* by offering other absorption locations for the two input photons, electronic structure permitting. These alternatives could also serve as precursors to the desired acceptor excited state. For example, direct two-photon absorption (TPA) at the acceptor needs to be discounted. Furthermore, if the excitations were not absorbed by two donors, but instead by one donor and the acceptor, singlet–singlet (S–S) annihilation between the two chromophores would also result in the observed fluorescence; however, this option is precluded when acceptor electronic states are not amenable to single-photon absorption of hν. This highlights the advantage of having donor and acceptor chromophores which are either chemically different or are rendered electronically so by fields in their local environment. Finally, TPA at a donor followed by two-centre energy transfer to the acceptor might also account for the requisite energetics. This pathway can be discounted by ensuring that donor chromophores exhibit no absorption band at approximately 2hν. Loss of excitation at the energy transfer stage is limited to S–S annihilation between two donors. Clearly, under such circumstances, the acceptor would not exhibit the required excitation as the S–S annihilation precludes it. For the analysis in this paper, we assume that these criteria which discount S–S annihilation and TPA effects are satisfied. In systems where S–S annihilation and TPA are observed, biexciton energy transfer represents a competing process which must be accommodated into any rate analyses.
Theory
Permissible wavefunctions for the biexciton |Ψi〉 can be written as linear combinations of the chromophore orbital states, each component describing alternative positionings of the twin excitations. Such local positionings are quantum mechanically realisable through wavepacket collapse on any suitable act of measurement. Explicitly, these wavefunctions are |  | (2a) |
|  | (2b) |
|  | (2c) |
with overbars indicating complex conjugates. Here, matter states are represented with a shorthand for the product of interacting donor species wavefunctions, for example, | |AuBuC0〉
≡
|ψuA〉|ψuB〉|ψ0C〉 | (3) |
where ψu/0ξ is the excited-state/ground-state wavefunction of species ξ. In eqn. 2b and c, the degenerate states |Ψ2〉 and |Ψ3〉 contain the complex coefficient |  | (4) |
and exhibit Davydov splitting of 3〈ξu|V|ξ′u〉
= 3ν, where V is the energy of interaction between ξ and ξ′. The multiplicative coefficients of eqn. 2, as in the single-photon exciton case, form the character table for the cyclic group C3.65 The coefficients of |Ψ1〉 are those of the irreducible representation A, and |Ψ2〉 and |Ψ3〉 those of the degenerate representations E.
The quantum electrodynamic calculations for biexcitonic energy transfer are here based on fourth-order, time-dependent perturbation theory.65,67 The resulting quantum amplitude for biexciton resonance energy transfer, M3;2fi, is expressible as
| M3;2fi
=
MABfi
+
c±MCAfi
+
c∓MBCfi | (5) |
where contributions are tempered by the pre-multipliers
c± and
c∓, which prove to offer a convenient way of generalising the different biexcitonic states in the following calculation. Explicitly,
| 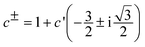 | (6) |
when
c′
= 1, the upper signs in
eqn. 6 generate |
Ψ2〉 and the lower, |
Ψ3〉. The state |
Ψ1〉 results from
eqn. 6 when
c′
= 0. In
eqn. 5, each contributor is denoted by the two excited species involved,
ξ and
ξ′. In general,
| Mξξ′fi
=
coopMξξ′fi
+
acc1Mξξ′fi
+
acc2Mξξ′fi | (7) |
where the superscripts coop, acc1 and acc2 denote mechanistic pathways by which exciton relaxation may occur (see
Fig. 2). In 3CET studies, these pathways are designated either cooperative or accretive in nature. Each type of relaxation encompasses a species at which a two-
photon interaction is observed. In the cooperative (coop) mechanism, it is the acceptor itself which exhibits TPA characteristics; conversely, in the accretive mechanisms (acc1 and acc2), an acceptor adopts a role similar to the bridging species in some multichromophore systems and fleetingly accrues the net excitation from its fellow excited species, which leads to it displaying inhomogeneous two-
photon scattering features. In full,
| coopMξξ′FI
=
μFI(ξ)iVij(nK,RDξ)αu0(D)jk(−cK,−cK)Vkl(nK,RDξ′)μFI(ξ′)I | (8a) |
| acc1Mξξ′FI
=
μFI(ξ)iVij(nK,Rξξ)αFI(ξ′)jk(2cK,−cK)Vkl(2n′K,RDξ′)μu0(D)I | (8b) |
| acc2Mξξ′FI
=
μFI(ξ′)iVij(nK,Rξ′ξ)αFI(ξ)jk(2cK,−cK)Vkl(2n′K,RDξ)μu0(D)I | (8c) |
Here we introduce the inter-
chromophore vector displacements
Rξ′ξ
=
Rξ′
−
Rξ and refractive indices
n
(for the medium through which the energy is transferred) and
n′
(at double the transferral frequency, 2
cK). Also introduced in
eqn. 8 is the transition dipole moment of a species
ξ from the initial,
I, to the final system state,
F:
μFI(ξ)
=
〈
ξF|
μ(
ξ)|
ξI〉,
μ(
ξ) being the electric dipole moment operator for
ξ. Naturally, the transfer energy
ħcK includes the appropriate Davydov splitting energies, here given by
ε. Specifically, for |
Ψ1〉,
ε
= 2
ν, and for both |
Ψ2〉 and |
Ψ3〉,
ε
=
−
ν. Thus,
| 2ħcK
= 2Eu0ξ
+
ε
=
Eu0D | (9) |
where
ESS′ξ
=
ESξ
−
ES′ξ and
ESξ is the energy of
ξ in state |
ξS〉. The quantity
Eu0ξ in
eqn. 9 relates to the electronic excitation energy of a donor,
ξ, and is equal to the input
photon energy
ħν, subject to dissipative losses.
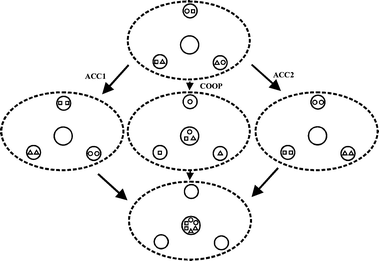 |
| Fig. 2 Quantum pathways coop, acc1 and acc2 for biexciton energy transfer in a system with three donors surrounding a central acceptor. In the initial biexciton state (top), positions of excitations for the three biexciton states are depicted by △, □, and ○. In the acc pathways, the net excitation accrues in any one donor and transfers to the acceptor to give the final state (bottom). In the coop pathway (centre), one unit of excitation passes to the acceptor, leaving a single-photon excitation residue which separately transfers to the acceptor. | |
The quantum amplitudes describing the three 3CET pathways (eqn. 8) display two types of second-rank tensor, one governing matter interaction, the other radiation. First, the well-known two-photon interaction tensor is given, in general, by
|  | (10) |
In order to avoid singularities the finite lifetime of the virtual states of
ξ,
ζ, are fully recognised in phenomenological damping corrections to the state energy:
where the virtual state takes on a Lorentzian profile
Γζ.
68 The correct signing of the damping,
i.e. with the same sign in both denominators of
eqn. 10, is the subject of a proof recently given elsewhere.
69 It is in
eqn. 11 that the aforementioned two-
photon behaviour associated with one of the
chromophores is manifest. Depending on the type of mechanism, the two-
photon interaction tensor assumes either two-
photon absorption (coop) or scattering (acc1/acc2) characteristics.
The other type of second-rank tensor present in eqn. 8 is the well-studied, index-symmetric coupling tensor V(nk,r). Each occurrence signifies an energy transfer-type interaction between two chromophores via media-corrected electric dipole–electric dipole coupling.70 For example, in the first term of eqn. 5, the first instance resulting from eqn. 8a descibes transfer between species A and D. Here, the transfer tensor is explicitly
|  | (12) |
with intervening media effects evident in both the Lorentz pre-multiplier and other terms containing the refractive index. In real dispersive media, the imaginary part of the refractive index imposes an exponential decay from within the phase factor; thus, in
eqn. 12, the transfer tensor reduces to the vacuum case as
n
→ 1.
Application to the model photosystem
In the previous section, we presented the form and structure of a biphoton exciton created in the model photosystem defined by inter-chromophore vectors shown in Fig. 3. Continuing, we adapt the results encapsulated in eqn. 5 to facilitate calculations that elicit geometric dependence. First, identifying the usual condition 2n′KR
≈
nKR
≪ 1 signifying transfer-pair distances substantially less than the input optical wavelength, we reduce each electric dipole–electric dipole tensor to its short-range (SR) form. For example, eqn. 12 becomes |  | (13) |
Furthermore, for calculational simplicity, we assume both that all transition moments associated with individual species (either real or virtual) are collinear and n
≈
n′.
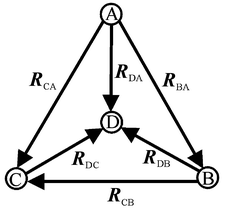 |
| Fig. 3 Inter-chromophore vectors in the model photosystem. | |
Using techniques analogous to those in ref. 61, we can re-write the nine contributions to eqn. 5, viaeqn. 8, in terms of angles rather than tensors/vectors:
| 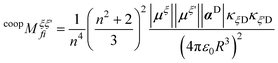 | (14a) |
| 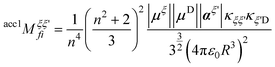 | (14b) |
| 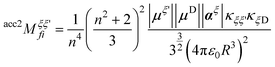 | (14c) |
where we employ the intermolecular lengths of
Fig. 2 and introduce the short-hand notation, and
μFI(ξ)
=
μ(ξ) and
αFI(ξ)
=
α(ξ). Most importantly, variations of the well-known orientation factor
κ are present in
eqn. 14.
71,72 Obviously, due to the added complexity of the system herein, a single
κ is inadequate to describe the necessary behaviour. Explictly, we define
| κAD
= cos ΘAD
− 3cos ϕAcos ϕD | (15a) |
| κBD
= cos ΘBD
− 3cos θBcos θD | (15b) |
| κCD
= cos ΘCD
− 3cos γCcos γD | (15c) |
| κAB
= cos ΘAB
− 3cos ΛAcos ΛB | (15d) |
| κAC
= cos ΘAC
− 3cos ΦAcos ΦC | (15e) |
| κBC
= cos ΘBC
− 3cos ΞBcos ΞC | (15f) |
where
| μξμξ′
=
|μξ‖μξ′|cos Θξξ′ | (16a) |
| μξRDA
=
|μξ‖RDA|cos ϕξ | (16b) |
| μξRDB
=
|μξ‖RDB|cos θξ | (16c) |
| μξRDC
=
|μξ‖RDC|cos γξ | (16d) |
| μξRBA
=
|μξ‖RBA|cos Λξ | (16e) |
| μξRCA
=
|μξ‖RCA|cos Φξ | (16f) |
| μξRCB
=
|μξ‖RCB|cos Ξξ | (16g) |
Angles subtended by donor transition moments and respective inter-
chromophore vectors are illustrated in
Fig. 4; those involving the acceptor moment, both with other moments and inter-
chromophore vectors, are given in
Fig. 5.
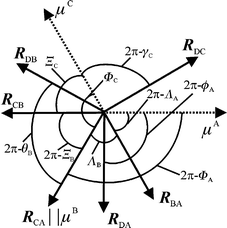 |
| Fig. 4 Angles subtended by donor transition moments and respective inter-chromophore vectors. | |
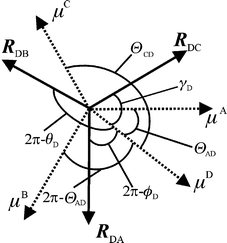 |
| Fig. 5 Angles subtended by the acceptor moment with both inter-chromophore vectors and donor transition moments. | |
Analysis of the above equations must accommodate the intrinsic symmetry of the equilateral triangle formed by the donors. This property is served by ensuring that the donor transition dipoles, illustrated in Fig. 6, are mutually arranged such that
To proceed further, however, the number of angles involved in
eqn. 7 must be reduced to a more manageable number. To this end, two special cases are considered.
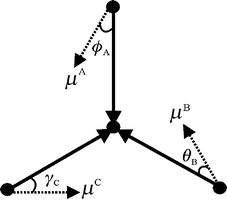 |
| Fig. 6 System geometry illustrating the case of ϕA
=
θB
=
γC. | |
Planar system
When the entire system, including transition dipoles, is co-planar, we may describe the system in terms of only three angles. First, we portray free, in-plane rotation of the acceptor transition moments with respect to the donor–acceptor intermolecular vectors in terms of the angle ϕD. Implementing the criterion of eqn. 17 and noting Fig. 5, we recognise | 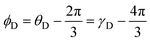 | (18) |
Secondly, the angles between the donor and acceptor transition moments can be expressed in terms of the angle ΘAD alone. Explicitly, | 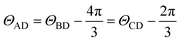 | (19) |
as can also be seen in Fig. 5. Finally, we define all other relevant angles (those between donor transition moments and donor–donor intermolecular vectors) in terms of ϕA: |  | (20) |
Noting that, due to the planar nature of the system, | 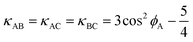 | (21b) |
we may write the total quantum amplitude as |  | (22) |
where the first term exclusively addresses the cooperative contributions, the second describes the accretive. The magnitude of all donor transition moments are equal, as are the donor polarisibilities; these are represented by the short-hand notation |μA| and |αA|, respectively, with a similar abbreviation for acceptor parameters.
In the excitonic state |Ψ1〉, we observe that c±
=
c∓
= 1, and the accretive part of eqn. 22 vanishes. This implies that biexcitonic transfer from the symmetrical state only proceeds via coop-based mechanisms. Substituting in the explicit relationships for the κ factors, eqn. 22 becomes
| 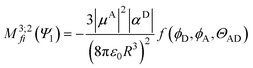 | (23) |
where
| f(ϕD,ϕA,ΘAD)
= 1 − 6cos ϕAcos(ϕD
−
ΘAD)
+ 9cos2ϕA | (24) |
This result mirrors rate results for the degenerate states of the single-
photon exciton states (see expression 24 in
ref. 63). As before,
eqn. 24 is zero when
| 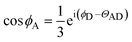 | (25) |
which only yields real solutions when
ϕD
=
ΘAD. The conjunction of the conditions
ϕA
= cos
−1(1/3) and
ϕD
=
ΘAD precludes energy transfer from the cooperative mechanism in biexcitonic energy transfer.
Calculation of the rate expressions using the Fermi golden rule entails modulus squaring the sum of the relevant quantum amplitude contributions. As the results of ref. 63 contain only a single κ factor in the relevant quantum amplitudes, the corresponding rate expressions go as κ2, the behaviour seen in the quantum amplitude of eqn. 23. Even though the number of angles needed to describe the biexcitonic system is greater than those required for the single-photon exciton, the system symmetry dictates a directly comparable geometric dependence. However, in single-photon excitons, energy transfer from the totally symmetric exciton to an in-plane acceptor dipole is forbidden, a condition shown to be valid in previous work.63 This selection rule is broken for the biexciton. Interestingly, transfer emanates from the cooperative mechanism, where symmetry is preserved, and not from the symmetry-breaking accretive pathways.
For the degenerate biexciton states |Ψ2〉 and |Ψ3〉, the quantum amplitude of eqn. 22 takes the form
|  | (26) |
Here,
M3;2fi(
Ψ+) and
M3;2fi(
Ψ−) represent the quantum pathways for energy transfer from |
Ψ2〉 and |
Ψ3〉, respectively. In
eqn. 26, the first term again reports information on cooperative mechanisms and the second on the accretive mechanisms. Explicitly, the observable for these biexcitonic energy transfer processes,
i.e. the rate,
Γ, is derived from the Fermi golden rule:
| 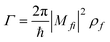 | (27) |
where
ρf is the density of acceptor final states. From
eqn. 26 and
27 we have
|  | (28) |
where the first and third terms represent pure cooperative and accretive contributions respectively, and the second term quantifies their quantum interference. Concentrating on the angular dependences in
eqn. 28, we may ascertain values which equate to optimum transfer probabilities for each contribution. Plotting these maxima against the angle
ϕA
(see
Fig. 7), we observe that it is the cooperative contribution that has the greatest influence, with the cross-term offering a small (but not insubstantial) input and the accretive contribution being effectively negligible. This is to be expected, as a donor separation angle of 2π/3 gives a significant bias toward cooperative transfer.
60 Examples of the angle maps from which these results are extracted are given in
Fig. 8 and
9.
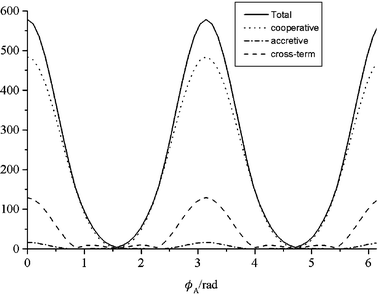 |
| Fig. 7 Maxima for energy transfer rate contributions plotted against ϕA. | |
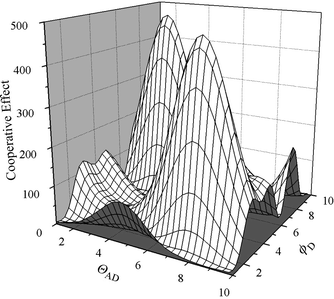 |
| Fig. 8 Contour map showing the cooperative contribution to eqn. 28. Here, ϕA
= 0 | |
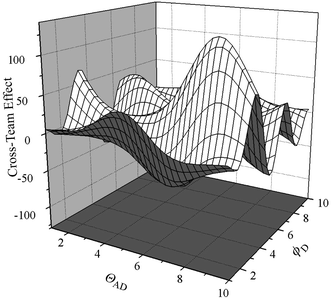 |
| Fig. 9 Contour map showing the cooperative–accretive cross-term contribution to eqn. 28. Here, ϕA
=0 | |
Acceptor transition moment perpendicular to the donor plane
Now consider the case where the acceptor transition moment, and also the salient components of the polarisability tensor, are fixed perpendicular to the plane of the model photosystem, i.e. | 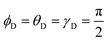 | (29) |
A result of symmetry between the donor transition moments is | cos ΘAD
= cos ΘBD
= cos ΘCD | (30) |
and alsoHence, noting the results of eqn. 20 and 21b still hold true, the quantum amplitude (eqn. 7) may be written as |  | (32) |
with the terms linear and quadratic in |μA| embodying the cooperative and accretive contributions, respectively.
For the degenerate biexcitonic states and |Ψ2〉 and |Ψ3〉, the pre-multiplier 1 +
c±
+
c∓ is zero, obviating any potential transfer. For the symmetric state, eqn. 32 reduces to
|  | (33) |
where, the first term again gives the response for cooperative transfer, which follows a regulation cos
2 regimen in
ΘAD, and the latter gives that of the accretive mechanisms.
Discussion
In this work, we have derived results for model photosystems which display the motif of three-fold symmetry that characterises many natural and artificial light-harvesting materials. The simple addition of one further excitation to an excitonic energy transfer system has been shown to significantly complicate mechanistic issues. However, juxtaposition with the results given by the simpler, single-photon excitation system generates a number of novel and interesting features. In the planar system, symmetry dictates that, in the single-photon excitation case, transfer is forbidden from the totally symmetric exciton state. In the case under study here, the cooperative transfer channel breaks this rule and migration proceeds. Both excitonic forms exhibit transfer from the degenerate basis states. Interestingly, the result governing single-photon excitonic energy transfer from the degenerate states is found within the expressions for the two-photon exciton cooperative behaviour. As no cancellation is observed when considering the degenrate two-photon exciton states, the form of eqn. 28 is complex, but Fig. 7 clearly shows that the cooperative pathway offers a leading term. With regard to the perpendicular system, only the symmetric state offers a viable transfer route. Given sufficient photon flux to generate a biexciton in the chosen array (the relatively modest intensity regime of two-photon absorption processes), it should be possible to observe the novel features we have elicited in the theory; moreover knowledge of their detailed geometric dependence should be of assistance in devising and optimising of biomimetic photosystem analogues.
Concerning further development of the theory, we note that the detailed form of spectral overlap associated with biexciton energy transfer mechanisms should also prove a matter of considerable interest. Elsewhere,70 it has been shown how the QED formulation of conventional single-donor energy transfer elicits a frequency-weighted overlap between the donor fluorescence spectrum and the dispersive absorption cross-section of the acceptor—the detailed form depending on distance, and in the short range, leading to a familiar Förster result. In the present context of twin-donor biexciton transfer, initial excursions into the theory reveal that the corresponding results differ for each mechanism. In the case of cooperative biexciton transfer, the result depends on the inverse square of the donor lifetime. Moreover, the spectral overlap integral entails not the donor fluorescence spectrum, but its autocorrelation function, and the (two-photon) acceptor cross-section is, of course, intensity dependent. For the accretive mechanism the result is still further removed from the familiar form, since it entails a convolution of the donor fluorescence and its anti-Stokes electronic emission lineshape. The complexity of the issue invites further detailed consideration and is the subject of ongoing work.
Acknowledgement
The authors would like to thank the Engineering and Physical Sciences Research Council for funding this project.
References
- G. McDermott, S. M. Prince, A. A. Freer, A. M. Hawthornthwaite-Lawless, M. Z. Papiz, R. J. Cogdell and N. W. Isaacs, Crystal structure of an integral membrane light-harvesting complex from photosynthetic bacteria, Nature, 1995, 374, 517 CrossRef CAS.
- A. A. Freer, S. M. Prince, K. Sauer, M. Z. Papiz, A. M. Hawthornthwaite-Lawless, G. McDermott, R. J. Cogdell and N. W. Isaacs, Pigment-pigment interaction and energy transfer in the antenna complex of the photosynthetic bacterium Rps. acidophila, Structure, 1996, 4, 449 CrossRef CAS.
- J. Koepke, X. Hu, C. Muenke, K. Schulten and H. Michel, The crystal structure of the light-harvesting complex II (B800–850) from Rhodospirillum molischianum, Structure, 1996, 4, 581 CrossRef CAS.
- S. Karrasch, P. A. Bullough and R. Ghosh, The 8.5Å projection map of the light-harvesting complex I from Rhodospirillum rubrum reveals a ring composed of 16 sub-units, EMBO J., 1995, 14, 631 CAS.
- M. Z. Papiz, S. M. Prince, A. M. Hawthornthwaite-Lawless, G. McDermott, A. A. Freer, N. W. Isaacs and R. J. Cogdell, A model for the photosynthetic apparatus of purple bacteria, Trends Plant Sci., 1996, 1, 198 CrossRef.
- W. Kühlbrandt, D. N. Wang and Y. Fujiyoshi, Atomic model of plant light-harvesting complex by electron crystallography, Nature, 1994, 367, 614 CrossRef CAS.
- N. Krauss, W.-D. Schubert, O. Klukas, P. Fromme, H. T. Witt and W. Saenger, Photosystem I at 4 Å resolution represents the first structural model of a joint photosynthetic reaction centre and core antenna system, Nature Struct. Biol., 1996, 3, 965 CAS.
- M. R. Shortreed, S. F. Swallen, Z.-Y. Shi, W. Tan, Z. Xu, C. Devadoss, J. S. Moore and R. Kopelman, Directed energy transfer funnels in dendrimeric antenna supermolecules, J. Phys. Chem. B, 1997, 101, 6318 CrossRef.
- A. Archut and F. Vögtle, Functional cascade molecules, Chem. Soc. Rev., 1998, 27, 233 RSC.
- M. Drobizhev, A. Rebane, C. Sigel, E. H. Elandaloussi and C. W. Spangler, Picosecond dynamics of excitations studied in three generations of new 4,4′-bis(diphenylamino)stilbene-based dendrimers, Chem. Phys. Lett., 2000, 325, 375 CrossRef CAS.
- N. D. McClenaghan, F. Loiseau, F. Puntoriero, S. Serroni and S. Campagna, Light-harvesting metal dendrimers appended with additional organic chromophores: a tetranuclear heterometallic first-generation dendrimer exhibiting unusual absorption features, Chem. Commun., 2001, 2634 RSC.
- M. Drobizhev, A. Karotki, A. Rebane and C. W. Spangler, Dendrimer molecules with record large two-photon absorption cross section, Opt. Lett., 2001, 26, 1081 CrossRef CAS.
- S. Yokoyama, A. Otomo and S. Mashiko, Laser emission from high-gain media of dye-doped dendrimer, Appl. Phys. Lett., 2002, 80, 7 CrossRef CAS.
- V. Vicinelli, P. Ceroni, M. Maestri, V. Balzani, M. Gorka and F. Vögtle, Luminescent lanthanide ions hosted in a fluorescent polylysin dendrimer. Antenna-like sensitisation of visible and near-infrared emission, J. Am. Chem. Soc., 2002, 124, 6461 CrossRef CAS.
- D. M. Guldi, A. Swartz, C. P. Luo, R. Gomez, L. J. Segura and N. Martin, Rigid dendritic donor–acceptor ensembles: Control over energy and electron transduction, J. Am. Chem. Soc., 2002, 124, 10875 CrossRef CAS.
- A. Bar-Haim, J. Klafter and R. Kopelmann, Dendrimers as controlled artificial energy antennae, J. Am. Chem. Soc., 1997, 119, 6197 CrossRef CAS.
- A. Bar-Haim and J. Klafter, Geometric versus energetic competition in light harvesting by dendrimers, J. Phys. Chem. B, 1998, 102, 1662 CrossRef CAS.
- A. Adronov and J. M. J. Fréchet, Light-harvesting dendrimers, Chem. Commun., 2000, 1701 RSC.
- J. L. Sessler, V. L. Capuano and A. Harriman, Electronic migration and trapping in quinone-substituted, phenyl-linked dimeric and trimeric porphyrins, J. Am. Chem. Soc., 1993, 115, 4618 CrossRef CAS.
- P. Brodard, S. Matzinger, E. Vauthey, O. Mongin, C. Papamicaël and A. Gossauer, Investigations of electronic energy transfer dynamics in multiporphyrin arrays, J. Phys. Chem. A, 1999, 103, 5858 CrossRef CAS.
- R. K. Lammi, R. W. Wagner, A. Ambroise, J. R. Diers, D. F. Bocian, D. Holten and J. S. Lindsey, Mechanisms of excited-state energy-transfer gating in linear versus branched multiporphyrin arrays, J. Phys. Chem. B, 2001, 105, 5341 CrossRef CAS.
- P. G. Van Patten, A. P. Shreve, J. S. Lindsey and R. J. Donohoe, Energy-transfer modelling for the rational design of multiporphyrin light-harvesting arrays, J. Phys. Chem. B, 1998, 102, 4029.
- D. Kuciauskas, P. A. Liddell, S. Lin, T. E. Johnson, S. J. Weghorn, J. S. Lindsey, A. L. Moore, T. A. Moore and D. Gust, An artificial photosynthetic antenna-reaction centre complex, J. Am. Chem. Soc., 1999, 121, 8604 CrossRef CAS.
- R. S. Loewe, K.-Y. Tomizaki, W. J. Youngblood, Z. Bo and J. S. Lindsey, Synthesis of perylene–porphyrin building blocks and rod-like oligomers for light-harvesting applications, J. Mater. Chem., 2002, 12, 3438 RSC.
- D. M. Guldi, Fullerene–porphyrin architectures; photosynthetic antenna and reaction centre models, Chem. Soc. Rev., 2002, 31, 22 RSC.
-
Resonance Energy Transfer, ed. D. L. Andrews and A. A. Demidov, John Wiley and Sons, Chichester, 1999 Search PubMed.
- G. D. Scholes, R. D. Harcourt and G. R. Fleming, Electronic interactions in photosynthetic light-harvesting complexes: the role of carotenoids, J. Phys. Chem., 1997, 101, 7302 Search PubMed.
- X. J. Jordanides, G. D. Scholes and G. R. Fleming, The mechanism of energy transfer in the bacterial photosynthetic reaction centre, J. Phys. Chem. B, 2001, 105, 1652 CrossRef CAS.
- T. Renger, V. May and O. Kühn, Ultrafast excitation energy transfer dynamics in photosynthetic pigment-protein complexes, Phys. Rep., 2001, 343, 137 CrossRef CAS.
- S. Matsuzaki, V. Zazubovich, N. J. Fraser, R. J. Cogdell and G. J. Small, Energy transfer dynamics in LH2 complexes of Rhodopseudomonas acidophila containing only one B800 molecule, J. Phys. Chem. B, 2001, 105, 7049 CrossRef CAS.
- T. Ritz, S. Park and K. Schulten, Kinetics of excitation migration and trapping in the photosynthetic unit of purple bacteria, J. Phys. Chem. B, 2001, 105, 8259 CrossRef CAS.
- C. Devadoss, P. Bharathi and J. S. Moore, Energy transfer in dendritic macromolecules: molecular size effects and the role of an energy gradient, J. Am. Chem. Soc., 1996, 118, 9635 CrossRef CAS.
- H.-L. Wang, D. W. McBranch, V. I. Klimov, R. Helgeson and F. Wuhl, Controlled unidirectional energy transport in luminescent self-assembled conjugated polymer superlattices, Chem. Phys. Lett., 1999, 315, 173 CrossRef CAS.
- M. Maus, R. De, M. Lor, T. Weil, S. Mitra, U.-M. Wiesler, A. Herrmann, J. Hofkens, T. Vosch, K. Müllen and F. C. De Schryver, Intramolecular energy hopping and energy trapping in polyphenylene dendrimers with multiple peryleneimide donor chromophores and a terryleneimide acceptor trap chromophores, J. Am. Chem. Soc., 2001, 123, 7668 CrossRef CAS.
- K. P. Ghiggino, E. K. L. Yeow, D. J. Haines, G. D. Scholes and T. A. Smith, Mechanisms of excitation energy transport in macromolecules, J. Photochem. Photobiol., A, 1996, 102, 81 CrossRef CAS.
- J.-S. Hsiao, B. P. Krueger, R. W. Wagner, T. E. Johnson, J. K. Delaney, D. C. Mauzerall, G. R. Fleming, J. S. Lindsey, D. F. Bocian and R. J. Donohoe, Soluble synthetic multiporphyrin arrays. 2. Photodynamics of energy transfer processes, J. Am. Chem. Soc., 1996, 118, 11
181 CrossRef CAS.
- E. K. L. Yeow, D. J. Haines, K. P. Ghiggino and M. N. Padden-Row, Electronic energy transfer in multichromophoric arrays. A sequential and superexchange dynamics study, J. Phys. Chem. A, 1999, 103, 6517 CrossRef CAS.
- E. K. L. Yeow and K. P. Ghiggino, Electronic energy transfer in multichromophoric arrays. The effect of disorder on superexchange coupling and energy transfer rate, J. Phys. Chem. A, 2000, 104, 5825 CrossRef CAS.
- Th. Förster, Zwischenmolekulare energiewanderung und fluoreszenz, Ann. Phys., 1948, 6, 55 , English translation in:
Biological Physics, ed. E. V. Mielczarek, E. Greenbaum and R. S. Knox, American Institute of Physics, New York, 1993 Search PubMed.
- J. S. Avery, Resonance energy transfer and spontaneous photon emission, Proc. Phys. Soc., 1966, 88, 1 Search PubMed.
- L. Gomberoff and E. A. Power, The resonance transfer of excitation, Proc. Phys. Soc., 1966, 88, 281 Search PubMed.
- D. L. Andrews and B. L. Sherborne, Resonance energy transfer: a quantum electrodynamical study, J. Chem. Phys, 1987, 86, 4011 CrossRef CAS.
- D. L. Andrews, A unified theory of radiative and radiationless molecular energy transfer, Chem. Phys., 1989, 135, 195 CrossRef CAS.
- G. D. Scholes, A. H. A. Clayton and K. P. Ghiggino, On the rate of radiationless intermolecular energy transfer, J. Chem. Phys., 1992, 97, 7405 CrossRef CAS.
- G. Juzeliunas and D. L. Andrews, Quantum electrodynamics of resonance energy transfer, Adv. Chem. Phys., 2000, 112, 357 CAS.
- G. J. Daniels, R. D. Jenkins, D. S. Bradshaw and D. L. Andrews, Resonance energy transfer: the unified theory revisited, J. Chem. Phys., 2003, 119, 2264 CrossRef CAS.
- D. L. Dexter, A theory of sensitised luminescence in solids, J. Chem. Phys., 1953, 21, 836 CrossRef CAS.
- G. D. Scholes and K. P. Ghiggino, Rate expressions for excitation transfer IV. Energy migration and superexchange phenomena, J. Chem. Phys., 1995, 103, 8873 CrossRef CAS.
-
A. S. Davydov, Theory of Molecular Excitons, McGraw-Hill, New York, 1962 Search PubMed.
-
D. L. Dexter and R. S. Knox, Excitons, John Wiley and Sons, New York, 1965 Search PubMed.
- M. Kasha, H. R. Rawls and M. A. El-Bayoumi, The exciton model in molecular spectroscopy, Pure Appl. Chem., 1965, 11, 371 CAS.
-
H. van Amerongen, L. Valkunas and R. van Grondelle, Photosynthetic Excitons, World Scientific, Singapore, 2000 Search PubMed.
- T. Ritz, X. Hu, A. Damjanovic and K. Schulten, Excitons and excitation transfer in the photosynthetic unit of purple bacteria, J. Lumin., 1998, 76–77, 310 CrossRef.
- P. Herman, U. Kleinekathöfer, I. Barvík and M. Schreiber, Exciton scattering in light-harvesting systems of purple bacteria, J. Lumin., 2001, 94–95, 447.
- M. A. Palacios, F. L. de Weerd, J. A. Ihalainen, R. van Grondelle and H. van Amerongen, Superradiance and exciton (de)localisation in Light-Harvesting Complex II from green plants?, J. Phys. Chem. B, 2002, 106, 5782 CrossRef CAS.
- R. Kopelman, M. Shortreed, Z.-Y. Shi, W. Tan, Z. Xu and J. S. Moore, Spectroscopic evidence for excitonic localisation in fractal antenna supermolecules, Phys. Rev. Lett., 1997, 78, 1239 CrossRef CAS.
- S. F. Swallen, Z.-Y Shi, W. Tan, Z. Xu, J. S. Moore and R. Kopelman, Exciton localisation hierarchy and directed energy transfer in conjugated linear aromatic chains and dendrimeric supermolecules, J. Lumin., 1998, 76–77, 193 CrossRef.
- P. Reineker, A. Engelmann and V. I. Yudson, Excitons in dendrimers: optical absorption and energy transport, J. Lumin., 2001, 94–95, 203 CrossRef.
- R. D. Jenkins and D. L. Andrews, Three-centre systems for energy pooling: quantum electrodynamical theory, J. Phys. Chem. A, 1998, 102, 10
834 CrossRef CAS.
- R. D. Jenkins and D. L. Andrews, Twin-donor systems for resonance energy transfer, Chem. Phys. Lett., 1999, 301, 235 CrossRef CAS.
- R. D. Jenkins and D. L. Andrews, Orientation factors in three-centre energy pooling, Phys. Chem. Chem. Phys., 2000, 2, 2837 RSC.
- G. J. Daniels and D. L. Andrews, The electronic influence of a third body on resonance energy transfer, J. Chem. Phys., 2002, 116, 6701 CrossRef CAS , Erratum: 2002, 117, 6882.
- R. D. Jenkins and D. L. Andrews, Exciton resonance energy transfer: effects of geometry and transition moment orientation in model photosystems, Photochem. Photobiol. Sci., 2003, 2, 130 RSC.
- P. Herman and I. Barvík, Towards proper parametrisation in the exciton transfer and relaxation problem. II. Trimer, Chem. Phys., 2001, 274, 199 CrossRef CAS.
- R. D. Jenkins and D. L. Andrews, Multichromophore excitons and resonance energy transfer: molecular quantum electrodynamics, J. Chem. Phys., 2003, 118, 3470 CrossRef CAS.
- L. Valkunas and G. Trinkunas, Non-linear exciton annihilation and local heating effects in photosynthetic antenna systems, Photochem. Photobiol., 1997, 66, 628 CAS.
-
D. P. Craig and T. Thirunamachandran, Molecular Quantum Electrodynamics: An Introduction to Radiation Molecule Interactions, Dover, New York, 1998 Search PubMed.
- D. L. Andrews, S. Naguleswaran and G. E. Stedman, Phenomenological damping of nonlinear-optical tensors, Phys. Rev. A, 1998, 57, 4925 CrossRef CAS.
- D. L. Andrews, L. C. Dávila Romero and G. E. Stedman, Polarizability and the resonance scattering of light: damping sign issues, Phys. Rev. A, 2003, 67, 55
801 CrossRef CAS.
- G. Juzeliunas and D. L. Andrews, Quantum electrodynamics of resonance energy transfer, Adv. Chem. Phys., 2000, 112, 357 CAS.
-
B. W. Van der Meer, in Resonance Energy Transfer, ed. D. L. Andrews and A. A. Demidov, John Wiley and Sons, Chichester, 1999 Search PubMed.
- B. W. van der Meer, Kappa-squared: from nuisance to new sense, Rev. Mol. Biotech., 2002, 82, 181 CrossRef CAS.
|
This journal is © The Royal Society of Chemistry and Owner Societies 2004 |