DOI:
10.1039/B303594H
(Paper)
J. Mater. Chem., 2004,
14, 111-115
Magnetic and magnetotransport properties of Fe2P nanocrystallites via a solvothermal route
Received 1st April 2003, Accepted 30th October 2003
First published on 20th November 2003
Abstract
Hexagonal nanocrystalline Fe2P has been synthesized via a solvothermal route at a relatively low temperature of 180 °C. X-Ray diffraction (XRD), X-ray photoelectron spectra (XPS) and transmission electron microscopy (TEM) were used to investigate the phase, composition, and morphology of the final crystallized product. The particles with a uniform hexagonal structure are stable in air and their size is in the range of 100–120 nm. A magnetoresistance effect is observed at comparatively low temperatures, which originates from the spin polarized tunneling effect. The super-paramagnetic state transformation can be found at the blocking temperature in Fe2P nanoparticles, further confirmed by electron spin resonance (ESR) experiments. Metamagnetic behavior is also observed in nanocrystalline Fe2P with variation of field because of the coexistence of ferromagnetic and super-paramagnetic states.
Introduction
Transition metals form a large number of metal phosphide compounds comprising over 125 binary and over 80 ternary substances because of the diversity in metal identity and metal/phosphorus stoichiometry.1 Generally, bulk band gap energies for most of these transition metal phosphides fall into the range of near 0 to over 3 eV similarly to semiconductors, and many metal phosphides display unique physical properties such as phosphorescent, magnetic, electronic and catalytic properties.2–4 Owing to their important properties and potential applications in many fields, such as semiconductors, luminescent devices, electronic components and hydro-processing catalysts,2,5–7 transition metal phosphides have attracted considerable interest in the past decades. Nevertheless, the magnetoresistance (MR) effect of transition metal phosphides has not been reported except for the alloy Fe1.96Ru0.04P.8 Since magnetoresistive materials are the active components of magnetic field sensors and read heads for computer hard drives because of their dependence of electric resistance on magnetic field,9,10 it is worth investigating the origin of magnetoresistance and exploring new transition metal phosphides. As a excellent magnetic material, the dimetal iron phosphide Fe2P is a well-known ferromagnet with a first order para- to ferromagnetic transition at 217 K.11 Considering that the d electrons of Fe are partially polarized,12 when the size of Fe2P is reduced to a few hundred nanometers, the inter-grain spin polarized tunneling is enhanced,13 making the magnetoresistance effect possible. Meanwhile, the magnetic properties of nanocrystalline particles are different compared with bulk Fe2P, stemming from its reduced dimensions.Conventional synthesis of metal phosphides usually involves the direct reaction of the appropriate elements for prolonged periods at high temperatures,14 reaction of phosphine with metals or metal oxides, reduction of metal phosphates by carbon, electrolysis of molten metal phosphate salts,15 metal organic chemical vapor deposition, and the high-temperature self-propagating route.16–18 These procedures typically require high reaction temperatures and long annealing periods to produce crystalline materials. However, most of these methods are only suitable for preparing bulk materials, and the overriding synthetic challenge is still the preparation of nanocrystalline transition metal phosphides with precise metal/phosphorus stoichiometry. Recently, Lukehart et al.19 reported a low-temperature reaction, which affords crystalline nanoclusters of transition metal phosphides in a silica xerogel matrix by the decomposition of molecular precursors. Clark et al.20 also reported a similar route for preparing a hydroprocessing catalyst. Furthermore, solution chemical synthesis techniques have also been developed for synthesizing nanocrystalline Co2P, Ni2P and FeP particles under rather mild temperatures.21–23 Among these techniques, the solvothermal method promises a single step of low-temperature synthesis, superior composition and morphological control.
Though nanocrystalline Fe2P was first reported by Lukehart et al.24 in 1995, the nanocrystallites were dispersed throughout a silica xerogel matrix and formed a nanocomposite, which cannot afford an opportunity to investigate the unique properties of nanocrystalline Fe2P itself. In this paper, through a mild solvothermal method, we have synthesized, for the first time, a nanocrystalline dimetal iron phosphide that is stable in air. The magnetic and magnetotransport properties of Fe2P have also been investigated.
Experimental
In the synthesis of Fe2P nanocrystallites, freshly obtained FeCl2·6H2O microcrystals and analytically pure yellow phosphorus were used as the starting materials in a molar ratio of 1∶5. FeCl2·6H2O crystals were prepared by dissolving appropriate amounts of FeCl3 and Fe in aqueous HCl, subsequently filtering and then cooling in a refrigerator. The typical synthesis procedure is as follows. 1.24 g (10 mmol) of yellow phosphorus, washed free of water by using absolute ethanol and toluene successively, and 0.470 g (2 mmol) FeCl2·6H2O were put into a 100 ml Teflon lined autoclave, which was then filled with ethylenediamine up to 85% of its capacity. The autoclave was maintained at 150–200 °C for 48 h and then cooled to room temperature naturally. The black precipitate was collected and washed with benzene, distilled water, and absolute ethanol several times in sequence. The final product weighed 0.120 g after drying in vacuum at 80 °C for 12 h. The yield was about 84%.The obtained products were characterized by D/max-2000 rotating powder diffractometer (Rigaku, Japan) using Cu-Kα radiation (λ
= 1.5418 Å). X-Ray diffraction (XRD) patterns were obtained at a scanning rate of 8° min−1 over the 2θ range 20–80°. The purity and composition of the sample were determined by X-ray photoelectron spectra (XPS), which were carried out in an ion-pumped chamber (evacuated to 2 × 10−9 Torr) of an Escalab5 (UK) spectrometer, employing Mg-Kα radiation (binding energy (BE)
= 1253.6 eV). Moreover, the morphology and size were both examined by transmission electron microscopy (TEM, H-800, Hitachi, Japan). Resistance was measured in the temperature range of 300–5 K on a MagLab System 2000 (Oxford, UK) using a standard dc four-probe technique with the target products pressed into pellets with diameter of 6 mm. Magnetization measurements were performed on a commercial superconducting quantum interference device (SQUID). Electron spin resonance (ESR) experiments were conducted on an ER-200D spectrometer (Bruker, Germany) at a microwave frequency of 9.5 GHz in the temperature range 110–300 K.
Results and discussion
Fe2P crystallizes in the hexagonal phase with space group P
2m. This structure presented in Fig. 1 shows two different metal sites: tetrahedral Fe (3f) in position (0.2568, 0, 0) with four P atoms as nearest neighbors (NN) and pyramidal Fe (3g) in position (0.5962, 0, ½) with five P atoms as NN.25 In the XRD patterns of Fig. 2, all peaks of Fe2P obtained at 180 °C can be well indexed to a single hexagonal cell with a
= 5.88 Å, c
= 3.43 Å. These cell parameters are in good agreement with the JCPDS card file 33-0670.26 Moreover, the relative intensity of (111) to (002) obtained for the as-prepared nanocrystals is 6.0, which is higher than that of the bulk material, indicating the existence of somewhat preferential orientation in the Fe2P nanocrystals. In the solvothermal process, we have done some related experiments to explore the possible mechanism in the formation of iron phosphide. During the whole reaction process, ethylenediamine not only acted as an N-chelating ligand to chelate with the iron ions and form stable complexes, but also as a basic solvent, which activates the reductive ability of yellow phosphorus. Thus iron ions are easily reduced to active atoms. Meanwhile, yellow phosphorus also disperses homogeneously in ethylenediamine and is rather active under the solvothermal conditions. Once generated, the metallic atoms will immediately combine with elemental phosphorus to form the corresponding metal phosphide. In this solvothermal process, the possible reactions in the formation of iron phosphide can be described as follows:21 | P4
+ 14 en + 16 H2O → 14 H2en2+
+ 4 HPO42−
+ 20 e | (1) |
| Fe2+
+ 3 en → Fe(en)32+ | (2) |
| Fe(en)32+
+ 2 e → Fe + 3 en | (3) |
| 8 Fe + P4
→ 4 Fe2P | (4) |
 |
| Fig. 1 Crystal structure of hexagonal Fe2P. Two inequivalent iron positions, tetrahedral and pyramidal, are distinguished and their nearest atomic neighbors are shown. | |
 |
| Fig. 2 The XRD patterns of Fe2P nanoparticles prepared under different temperatures. | |
During the reaction process, an appropriate amount of water is necessary. It is concluded that the appropriate reaction temperature for synthesizing nanocrystalline Fe2P is about 180 °C, above which the FeP appears, consistent with the XRD peaks below 40°, as observed in Fig. 2.27 If the reaction temperature is below 180 °C, the final products are only amorphous iron and phosphorus. Since the yellow phosphorus is in excess, Fe3P does not form in the reaction process.28
Fig. 3 displays the XPS results of as-prepared Fe2P. The binding energy (BE) of Fe
2p is about 707.4 eV, close to the BE of elemental Fe, which is nearly 707.0 eV. This indicates that the active valence of Fe is comparatively low and almost zero, consistent with the structure of Fe2P. Moreover, the BE peaks of 2s, 2p for phosphorus and 3s, 3p for iron were all observed in Fig. 3 and accordant with the normal values. The BE value of P
2p is only 130.0 eV, close to the BE value of elemental phosphorus (129.1 eV), indicating that the nanocrystalline Fe2P is stable in air and is not oxidized. The BE peaks indexed as O
KLL, O
1s, N
1s and C
1s in Fig. 3 originate from a small amount of CO2 and N2 molecules in the atmosphere. From the integral peak areas of Fe and P in the XPS spectra, the product is rather pure with molar ratio Fe∶P = 1.985∶1, which is close to the chemical formula of Fe2P. No obvious impurities such as chloride or phosphate could be detected in the sample, indicating that the level of impurities is lower than the resolution limit of XPS (1–5 atom%). From the transmission electron microscopy (TEM) images shown in Fig. 4, we can see that the size of Fe2P nanoparticles ranges from about 100 to 120 nm, with a uniform hexagonal shape, coincident with the trend of preferential orientation observed in Fig. 2. The electron diffraction (ED) pattern in the inset reveals that the Fe2P nanoparticles are polycrystalline and externally well crystallized.
 |
| Fig. 3 XPS analysis of as-prepared Fe2P product. | |
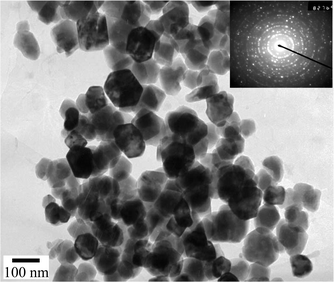 |
| Fig. 4 TEM micrograph for Fe2P nanoparticles. | |
Fig. 5 displays the temperature dependence of resistance and MR ratio (defined as (R0
T
−
R5
T)/R5
T) for nanocrystalline Fe2P particles. It is clear that the resistance values dramatically increase with the temperature, showing a metallic behavior. Compared with the bulk material, the influence of the scattering of carriers at grain boundaries on the temperature dependence of electric conductivity ought to be considered, which may lead to the enhancement of resistivity for nanocrystals. The MR% values are monotonously decreased along with increasing temperature, and then slightly increased above 245 K, which is very close to the ferro–paramagnetic transition temperature confirmed from Fig. 8. As shown in Fig. 6
(MR ratio is redefined as (R0
T
−
RB
T)/RB
T, where B is the external magnetic field), we measured the evolution of MR% with external field H at a temperature of 5 K. The MR ratio is appropriately linearly increased dependent on the external field and reaches about 1.2% in a field of 5 T. This MR effect is mainly caused by the spin polarized tunneling of polarized free electron on the d orbitals of Fe. Moreover, the enhanced inter-grain spin polarized tunneling in nanocrystalline Fe2P also contributes to the MR enhancement.13
 |
| Fig. 5 Temperature dependence of resistance and MR ratio obtained at 5 T for nanocrystalline Fe2P obtained at 180 °C. | |
 |
| Fig. 6 Field dependence of MR values for Fe2P at 5 K. | |
The magnetic characterization was carried out by several experiments. For single crystal Fe2P, the measured atomic magnetic moments of tetrahedral Fe (3f) and pyramidal Fe (3g) sites are 0.59 and 2.22 μB respectively, resulting in a net moment of 2.81 μB.29 From the hysteresis loop at 5 K of Fig. 7, we find that the magnetization of Fe2P is still not saturated even under an external field of 50 kG, and the unsaturated magnetization moment is 32.1 emu g−1
(0.82 μB/f.u.), which is much lower than the saturated moment of bulk Fe2P.29 The rather unusual shape of this hysteresis loop possibly indicates this material is comprised of a combination of paramagnetic and ferromagnetic constituents. Resolution of this complexity will result from more detailed measurements of the temperature dependence of the sample magnetization. Fig. 8 shows the temperature dependence of magnetization in the zero-field-cooled (ZFC) and field-cooled (FC) processes with an applied low field of 100 and 500 Oe. In the ZFC mode, the magnetization increases at first, and then decreases with an increase of temperature. The temperature, at which the maximum ZFC magnetization occurs, is characterized as the blocking temperature (TB
≈ 248 K) for the super-paramagnetic transformation.30 Meanwhile, the magnetization data as a function of temperature taken at 100 and 500 Oe in FC mode were used to determine the Curie temperature (TC). From the maximum value in the dM/dT vs. T curve, the obtained TC is about 250 K,31 different from the value of the bulk material.11 Usually, the blocking temperature is sensitive to the volume of single particles and magnetic anisotropy constant. The magnetic anisotropy constant (K), was deduced from the blocking temperature using the equation K
= 25kbTB/V, where kb is the Boltzman constant and V is the volume of the nanoparticle.32 When the size of nanoparticles increases, TB will approach TC and disappear with further increase of particle size. As for the Curie temperature, it is related to the size and crystallization of particles as well as the magnitude of the applied external field. From Fig. 9, we can judge that nanocrystalline Fe2P is a metamagnetic compound. The value of dM/dH first increases with H, reaches a maximum, and then decreases with a further increase of H. The given field, at which the maximum value is reached, is the critical field related to the magnetic transition. This magnetic transition may be attributed to the transformation from the super-paramagnetic to ferromagnetic state. In order to further understand the complicated magnetic behavior of this compound, we investigated ESR signals of the Fe2P nanoparticles. ESR detects the power P absorbed by the sample from the transverse magnetic microwave field as a function of the static magnetic field H. As shown in Fig. 10(a), the sample shows gradual X-band paramagnetic (PM) line transformations at above 260 K, which is consistent with the TB value in Fig. 8. Within the whole paramagnetic regime above 285 K, the spectrum consists of a broad, exchange narrowed resonance line, which is well fitted by a Dysonian line shape with a g factor of 1.995.33 As observed in Fig. 10(b), a ferromagnetic (FM) signal appears at 110 K and gradually disappears when the temperature approaches 230 K, consistent with the value of TC obtained from the magnetization (Fig. 8). When the temperature is lowered to 230 K, an additional small peak occurs around H
= 1000 G. This additional peak is related to the ferromagnetism.34,35 When the temperature is further lowered, the intensity of the additional peak gradually increases, accompanied by a gradual decrease of the PM peak. When the FM state occurs, the remaining PM regime is actually surrounded by an internal magnetic field induced by the FM phase. Therefore, at a lower applied magnetic field, the sum of the internal and applied fields can satisfy the required magnitude of the resonance field. Thus, the location of the PM peak is shifted to a lower field with decreasing temperature and an additional peak appears at lower field. On the other hand, temperature-dependent ESR signals of PM lines with g
= 1.996 also occur below 285 K, different from the PM lines above 285 K. Since the super-paramagnetic state coexists with the ferromagnetic state below TB (as deduced from Fig. 8), this newly PM state may be due to the contribution of the super-paramagnetism of nanocrystalline Fe2P. The two magnetic phases, FM and PM, coexist from 110 to 230 K, according to the ESR spectra, providing a direct evidence for the coexistence of FM and super-paramagnetic states in nanocrystalline Fe2P.
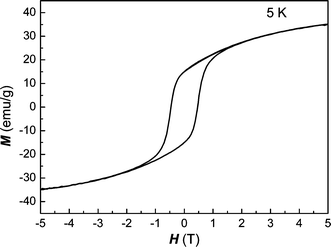 |
| Fig. 7 Hysteresis loop of nanocrystalline Fe2P at 5 K. | |
 |
| Fig. 8 ZFC and FC magnetization as a function of temperature in applied fields of 100 and 500 Oe. | |
 |
| Fig. 9 Typical M–H and dM/dH–H curves of Fe2P at 5 K. | |
 |
| Fig. 10 ESR signals at various temperatures for nanocrystalline Fe2P: (a) from 300 to 255 K (5 K intervals), (b) from 255 to 110 K (5 K intervals in the range 255–200 K, 10 K intervals in the range 200–110 K). | |
Conclusions
We have synthesized nanosized Fe2P particles through a mild solvothermal route based on the direct reaction of the metal halide with yellow phosphorus. The magnetoresistance effect is observed and reaches 1.2% at 5 K in a field of 50 kG, originating from the spin polarized tunneling effect. The super-paramagnetic state confirmed by magnetic measurements affects the metamagnetic behavior of nanocrystalline Fe2P, and the coexistence of ferromagnetic and super-paramagnetic states is also observed from 230 to 110 K according to ESR spectroscopy.Acknowledgements
The authors would like to acknowledge the support of MOST of China (G19980613), NSFC (Nos. 50272006, 20221101 & 20023005), Training Project for Doctoral Student of MOE, and the Founder Foundation of PKU.References
- H. G. von Schnering and W. Honle, Encyclopedia of Inorganic Chemistry, John Wiley & Sons, Chichester, UK, 1994, vol. 6, p. 3106 Search PubMed.
- B. Arinsson, T. Landstron and S. Rundquist, Borides, Silicides and Phosphides, John Wiley & Sons, New York, 1965 Search PubMed.
- R. Wappling, L. Haggstrom, T. Ericsson, S. Devanarayan, E. Karlsson, B. Carlsson and S. Rundquist, J. Solid State Chem., 1975, 13, 258 CAS.
- C. Stinner, R. Prins and Th. Weber, J. Catal., 2001, 202, 187 CrossRef CAS.
- V. B. Chernogorenko, S. V. Muchnik, K. A. Lynchak and Z. A. Klimak, Mater. Res. Bull., 1981, 16, 1 CrossRef CAS.
- A. Catalano and R. B. Hall, J. Phys. Chem. Solids, 1980, 41, 635 CrossRef CAS.
- M. Jian, J. L. Rico Cerda and R. Prins, Catal. Lett., 1995, 35, 193 CAS.
- T. Oki, T. Sato, Y. Uwatoko, H. Fujii and A. V. Andreev, J. Alloys Compd., 2000, 306, 1 CrossRef CAS.
- J. Brig, T. C. Anthony and J. Nickel, Mater. Res. Soc. Bull., 1996, 21, 23 Search PubMed.
- J. M. Daughton, J. Magn. Magn. Mater., 1999, 192, 334 CrossRef CAS.
- H. Fujii, Y. Uwatoko, K. Motoya, Y. Ito and T. Okamoto, J. Phys. Soc. Jpn., 1988, 57, 2143 Search PubMed.
- M. B. Stearn, Physica, 1977, 91(B + C), 37 Search PubMed.
- H. Y. Hwang, S. W. Cheong, N. P. Ong and B. Batlogg, Phys. Rev. Lett., 1996, 77, 2041 CrossRef CAS.
- Comprehensive Inorganic Chemistry, ed. J. C. Bailer, H. J. Emelius, R. Nyholm and A. F. Trotman-Dickenson, Pergamon, Oxford, 1973, vol. 2 Search PubMed.
- J. R. van Wazer, Phosphorus and Its Compounds, Interscience, New York, 1958, vol. 1 Search PubMed.
- P. R. Bonneau, R. F. Jarris and R. B. Kaner, Nature, 1991, 349, 510 CrossRef CAS.
- I. P. Parkin, Chem. Soc. Rev., 1996, 199 RSC.
- J. Gopalakrishnan, S. Pandey and K. K. Rangan, Chem. Mater., 1997, 9, 2113 CrossRef CAS.
- C. M. Lukehart, B. Milne Stephen and S. R. Stock, Chem. Mater., 1998, 10, 903 CrossRef CAS.
- Xian-Qin Wang, P. Clark and S. Ted Oyama, J. Catal., 2002, 208, 321 CrossRef CAS.
- Y. Xie, H. L. Su, X. F. Qian, X. M. Liu and Y. T. Qian, J. Solid State Chem., 2000, 149, 88 CrossRef CAS.
- Shu-Hong Yu, Jian Yang, Yong-Sheng Wu, Zhao-Hui Han, Lei Shu, Yi Xie and Yi-Tai Qian, J. Mater. Res., 1998, 13, 3365 CAS.
- Yun-Le Guo, Fan Guo, Yi-Tai Qian, Hua-Gui Zheng and Zi-Ping Yang, Mater. Res. Bull., 2002, 37, 1101 CrossRef.
- C. M. Lukehart, B. Milne Stephen, S. R. Stock, R. D. Shull and E. Wittig James, Mater. Sci. Eng. A, 1995, 204, 176 Search PubMed.
- H. Fujii, S. Komura, T. Takeda, T. Okamoto, Y. Ito and J. Akimistsu, J. Phys. Soc. Jpn., 1979, 51, 1616 Search PubMed.
- B. Carlson, J. Solid State Chem., 1973, 8, 57 CrossRef.
- S. Rundqvist and P. C. Nawapong, Acta Chem. Scand., 1965, 19, 1006 CAS.
- Fasiska and L. Zwell, Trans. Am. Inst. Min. Metall. Pet. Eng., 1967, 239, 924 Search PubMed.
- A. Koumina, M. Bacmann, D. Fruchart, J. L. Soubeyroux, P. Wolfers, J. Tobola, S. Kaprzyk, S. Niziol, M. Mesnaoui and R. Zach, Ann. Chim. Sci. Mater., 1998, 23, 177 CrossRef CAS.
- R. W. Li, H. Xiong, J. R. Sun, Q. A. Li, Z. H. Wang, J. Zhang and B. G. Shen, J. Phys.: Condens. Mater., 2001, 13, 141 CrossRef CAS.
- A. J. P. Meyer and M. C. Cadeville, J. Phys. Soc. Jpn. Suppl. (Proc. Int. Conf. Mag. Crys. Vol. 1), 1962, 17, 223 Search PubMed.
- S. J. Park, S. Kim, S. Lee, Z. G. Khim, K. Char and T. Hyeon, J. Am. Chem. Soc., 2000, 122, 8581 CrossRef CAS.
- G. Feher and A. F. Kip, Phys. Rev., 1955, 98, 337 CrossRef CAS.
- S. M. Zhou, M. Yoshizawa, P. Lou, S. Z. Jin and Y. H. Zhang, J. Appl. Phys., 2001, 89, 3377 CrossRef CAS.
- N. O. Moreno, P. G. Pagliuso, C. Rettori, J. S. Gardner, J. L. Sarrao, J. D. Thompson, D. L. Huber, J. F. Mitchell, J. J. Martinez and S. B. Oseroff, Phys. Rev. B, 2001, 63, 174413 Search PubMed.
|
This journal is © The Royal Society of Chemistry 2004 |
Click here to see how this site uses Cookies. View our privacy policy here.