Monitoring of labile metals in turbid coastal seawater using diffusive gradients in thin-films
Received
24th September 2002
, Accepted 11th November 2002
First published on 21st November 2002
Abstract
Diffusive gradients in thin-films (DGT) have been investigated for in situ monitoring of labile metals in north Australian coastal seawater. Field and experimental data showed that DGT devices provided adequate detection limits, accuracy and precision for monitoring of near-pristine levels of labile Mn, Co, Cu, Cd and Pb when deployed for periods of 3 days. However, Zn could not be adequately determined due to high blank levels. The ratio of DGT-labile to 0.45 µm-filtered metal levels in natural seawater ranged from 0.44–0.63 for Cu but was close to unity for Co and Cd. Elevated levels of suspended particulate matter up to 57.3 mg l−1 did not have an adverse effect on the performance of DGT.
Introduction
The diffusive gradients in thin films (DGT) technique introduced by Davison and Zhang1 was designed to provide in situ determination of kinetically labile metal species in aquatic systems. Since it is the labile fraction of the total metal inventory that is considered the most bio-available to aquatic organisms,2 DGT has the potential to become an important environmental monitoring tool. In Australia, as elsewhere, the recent introduction of new water quality guidelines3 has increased the need for cost-effective routine metal speciation methods. A range of other metal speciation techniques (e.g. anodic stripping voltametry, ion-selective electrodes and ligand competition techniques) provides useful information but fundamentally suffer from the disadvantage that sample collection and treatment may induce speciation changes. In addition to providing cost-effective in situ speciation information, the DGT technique is advantageous in providing a time-integrated measure during the deployment period and in avoiding the risk of sample contamination associated with collection and filtration of waters with low metal concentrations. The DGT devices are comprised of a layer of chelating resin immobilised in a gel (the resin gel) overlain first by an ion-permeable gel (the diffusive gel) and outermost a membrane filter in contact with the solution to be measured. By controlling the diffusion rate in the diffusive gel the convection rate in the measured solution becomes non-controlling in the metal uptake except in the most stagnant water bodies. Only free ions and relatively small labile complexes that are able to pass through the diffusive gel (pore size < 1–20 nm) and dissociate within the effective measurement time (a few minutes) will accumulate by chelation in the resin gel.4 To date, DGT has been used to determine labile metal species in both fresh and saline waters1,5–8 but very few studies9 have compared DGT with other metal analysis or speciation techniques in natural seawater.
This paper reports on a field trial of DGT to monitor labile metal levels in near-pristine coastal waters of northern Australia. In addition, experimental data for natural seawater was used to assess the performance of DGT over a range of labile metal concentrations and at elevated levels of suspended particulate matter (SPM) where fouling may be expected to affect DGT performance. Along the shallow coast of northern Australia SPM levels are commonly elevated (10–100 mg l−1) due to re-suspension of muddy bottom sediment by strong tidal currents and wave action.10
Methods
DGT preparation and analyses
Piston type DGT devices (20 mm diameter window), diffusive gel (type APA, thickness 0.8 mm) and Chelex resin gel were obtained from DGT Research Ltd. Trace metal clean procedures were used for all laboratory handling of DGT devices and associated equipment in a class 100 laminar flow cabinet. Gels and 0.45 µm membrane filters (Pall Gelman Laboratory GN6) were cut on an acrylic sheet using an acrylic punch. Initial tests showed that the caps holding the gels in place should not be re-used as cracks in the caps ‘weak point’ caused subsequent leakage and an overestimation of concentrations for short-term deployments (few hours). Loaded DGT were stored at 4 °C in polypropylene jars with several drops of high-purity water to prevent gels from drying out. For each deployment a minimum of 4 assembled DGT were left in storage for blank determination.
Following retrieval of deployed DGT their surfaces were rinsed with high purity water and stored in polypropylene jars at 4 °C. Upon disassembly, the resin gels were eluted in 2 ml of 10% nitric acid (AristaR grade) in 15 ml polypropylene vials placed vertically in an environmental shaker operating at 100 rpm at 30 °C for 12 h. The eluate was diluted by addition of 2 ml internal standard solution (40 µg l−1 Rh, In, Re) and high purity water to a total volume of 8 ml before analysis by inductively coupled plasma mass spectrometry (ICP-MS—Perkin Elmer ELAN 6000). Final seawater concentrations (after DGT blank subtraction) were calculated using the procedure of Davison and Zhang 1 and DGT Research Ltd.:11
|
Mass in resin: M
(µg)
=
[ConcentrationICPMS
(µg l−1)
× volume of solution (l)]/[elution factor]
| (1) |
|
Concentration in seawater: C
(µg l−1)
=
[M
(µg)
× diffusive gel + membrane thickness (cm)
× 1000]/[diffusion coefficient (cm2 s−1)
× time (s)
× gel area (cm2)]
| (2) |
In this study diffusion gel thickness = 0.08 cm; membrane thickness = 0.0153 cm; elution factor = 0.8; diffusion coefficients for APA gel (×10−6 cm2 s−1 at 25 °C): Mn = 5.85, Co = 5.94, Cu = 6.23, Zn = 6.08, Cd = 6.09, Pb = 8.03.11
Initial DGT test
The DGT devices were initially tested in filtered (0.45 µm) seawater collected from Darwin Harbour (salinity 27.7) and spiked with metal nitrate solutions to concentrations of 66.3 µg l−1 Mn, Cu, and Zn and 6.63 µg l−1 Co, Cd and Pb. The experiment was carried out in duplicate in magnetically stirred 4 l polypropylene containers (Nalgene) at 24 °C. Four DGT devices were suspended from nylon line and removed after 80, 158, 257 and 316 min, respectively. In this and the metal adsorption experiment reported below the concentrations of metals in solution were not substantially affected by DGT metal uptake. For the longest duration experiments the mass fraction taken up by DGT devices amounted to less than 2% of the total mass in solution.
Field trial
DGT devices were deployed at Fort Hill Wharf in Darwin Harbour, Northern Territory, Australia in the late wet-season (April 2002) in four 3-day periods over a range of SPM levels varying with the tidal regime, which included both neap tides (minimum amplitude 0.9 m) and spring tides (maximum amplitude 7.4 m). Although metal levels in Darwin Harbour in general are at near-pristine levels,10 in the past the Fort Hill Wharf area has been used for loading of ore-concentrate and may constitute a point source mainly for Cu and Pb. At the wharf the tidal stream varies from 0.05 m s−1 to 0.88 m s−112 exceeding the low threshold flow of 0.02 m s−1 required by the DGT technique.13 DGT were deployed in triplicate held face-down in a PVC holder suspended from a weighted nylon rope approximately 8 m above the seafloor and 1 m below the lowest tide level during the trial. At the time of DGT changeover conventional unfiltered and filtered (0.45 µm) water samples were collected using clean sampling techniques10 for later determination of metal and SPM concentrations. Salinity, pH, temperature, dissolved oxygen and turbidity were logged (Hydrolab Surveyor 4a) at 20 min intervals adjacent to the DGT samplers throughout their deployment.
Unfiltered seawater was collected from Darwin Harbour (salinity 35.6) in a 20 l polypropylene carboy and left to settle overnight. Clarified seawater was transferred to a stirred 4 l experimental container and settled SPM added to increase the SPM level to 57.3 mg l−1. The stirring action is likely to have caused fragile SPM flocculates to disaggregate to their primary grain size distribution.14 One DGT device was used to measure background metal concentrations over a 17 h period after which metal nitrate solutions were added to achieve nominal concentrations of 20 µg l−1 Mn, Cu, and Zn and 2.0 µg l−1 Co, Cd, and Pb. Six DGT were used to sequentially monitor labile metal concentrations during adsorption of metal to SPM over a 48 h period. Filtered (0.45 µm) water samples were collected during the experiment for determination of dissolved metal concentrations. Mineralogical analysis of SPM was carried out by X-ray diffraction analysis by CSIRO Land and Water (Adelaide, Australia) and CSIRO Minerals (Perth, Australia) carried out laser diffraction analysis of the particle size distribution.
Seawater analysis
Samples from the laboratory experiments containing high added metal levels were analysed directly by ICP-MS after dilution to a salinity of 2 using a matrix matched seawater blank and standard addition calibration. Blank seawater was processed as samples to determine the detection limits (3σ SD for process blanks in µg l−1): Mn = 0.5; Cu, Zn = 2; Co, Cd, Pb = 0.1. Both 0.45 µm filtered and unfiltered samples from the field trial were prepared by the APDC solvent extraction procedure of Batterham et al.15 and analysed by ICP-MS. This procedure provides total dissolved metal concentrations in filtered samples and total dissolved plus labile particulate metal concentrations in unfiltered samples.10 It does not extract Co from unfiltered samples or Mn from filtered or unfiltered samples. Detection limits were (3σ SD for process blanks in ng l−1): Zn = 10, Cu = 5 and Co, Cd, Pb = 2. Certified reference materials and metal spike additions were used for quality control.10 SPM was determined gravimetrically after sample filtration through 0.45 µm membrane filters.
Results
DGT blank levels
The DGT blanks for both laboratory experiments and the field trial were (in ng per disc ± 1σ SD): Mn = 0.21 ± 0.03, Co = 0.03 ± 0.01, Cu = 1.98 ± 0.17, Zn = 5.17 ± 2.86, Cd = 0.05 ± 0.02, Pb = 0.61 ± 0.04. The corresponding solution detection limits (3σ SD of DGT blanks) for a deployment of 3 days (72 h) at 30 °C were (in ng l−1): Mn = 1.7, Co = 0.5, Cu = 8.3, Zn = 145, Cd = 0.9 and Pb = 1.6. Solution detection limits are inversely proportional to the deployment time (eqn. (2)). The 3 day detection limits are below the 0.45 µm-filtered concentrations of all metals except Zn in north Australian coastal seawater.10 The high detection limit for Zn was due to a relatively high irreducible Zn level in the resin gel (2–4 ng per disc)11 and difficulty in handling the gels sufficiently cleanly.
Initial DGT test
The metal concentrations recovered by DGT relative to the nominal concentrations for the two longest deployments (257 and 316 min) were (in %): Mn = 101, Co = 106, Cu = 103, Zn = 110, Cd = 116 and Pb = 101. Given the uncertainties in the many factors contributing to the final solution concentration (eqns. (1) and (2)) the accuracy is considered satisfactory. For example, higher elution factors (0.92–0.95) than those recommended by DGT Research Ltd. (0.8) have been reported8,9 and lower diffusion coefficients may apply in seawater5,9 compared to low ionic-strength solutions. However, a higher elution factor and lower diffusion coefficients compared to those used here would affect the calculated solution concentrations in opposite directions and to some degree cancel out. For the two shortest deployments (80 and 158 min) the recoveries were on average 6% higher than the above recoveries. This was probably due to leakage around the DGT piston caps, leading to rapid metal saturation of a small portion of resin gel; such leakage will become progressively less important for longer deployment times as the metal mass in the saturated gel portion becomes relatively insignificant compared to the total metal mass accumulated through diffusion. This effect has not been observed in subsequent use of DGT devices where better sealing of caps was ensured. The precision of DGT determinations during this experiment was on average 4.2%
(1σ RSD, n
= 4), similar to that of other DGT investigations,6,16 and at these elevated metal levels, comparable to the precision achieved by other seawater analysis methods.15
Field trial
During the Fort Hill Wharf trial the logged physico-chemical parameters showed the following ranges: salinity = 34.9–36.5, pH = 7.78–8.07, temperature = 30.5–31.4 °C, dissolved oxygen saturation = 71–103% and turbidity = 9.3–37.3 NTU. Due to the relatively high detection limit for Zn compared to background seawater values and because Mn is not extracted by the solvent extraction procedure used, only Co, Cu, Cd and Pb data will be considered here. The ranges of DGT-labile and 0.45 µm-filtered concentrations obtained during the field trial are shown in Table 1 together with previously published data for Darwin Harbour.10Fig. 1a and b shows a comparison of DGT-labile, 0.45 µm-filtered and unfiltered metal concentrations together with simultaneously measured turbidity. Logged turbidity data and simultaneous SPM sampling showed the following relationship: turbidity = 0.949 × SPM + 8.35 (R2
= 0.966). The DGT precisions achieved at the low metal levels measured during the Fort Hill Wharf trial were significantly degraded (average of 1σ RSD in % for each of the four 3-day deployments: Mn = 8, Co = 6, Cu = 21, Cd = 16 and Pb = 17) compared to that achieved at high metal levels in the laboratory experiments. However, the precision is likely to be adequate for most monitoring purposes. In the Fort Hill Wharf trial fouling of filter membranes by algal growth and SPM adherence were just becoming visible after 3 days of deployment, which in these waters probably constitute an upper limit for fouling-free deployment.
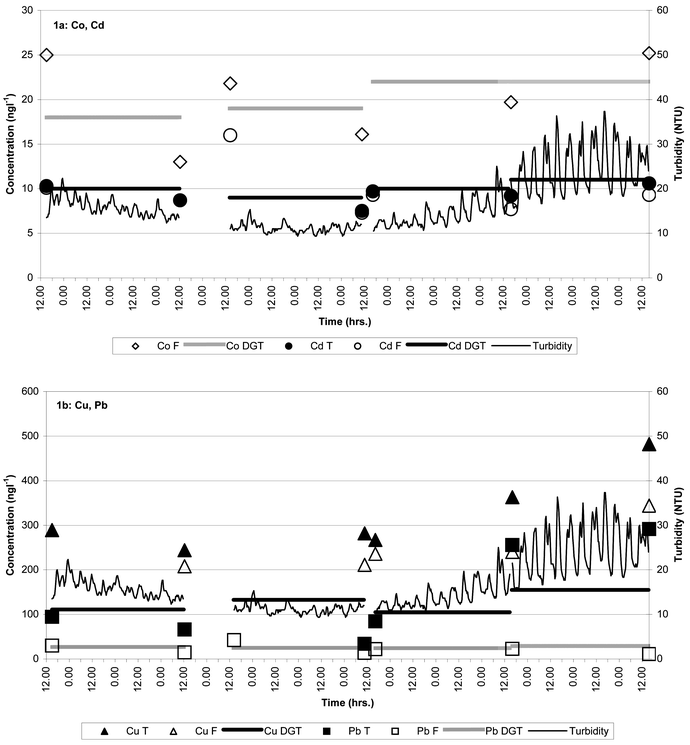 |
| Fig. 1 Concentrations of DGT-labile (DGT), 0.45 µm-filtered (F) and total dissolved + labile particulate (T) metals during four 3-day deployments at Fort Hill Wharf, April 2002 (Co(T) was not determined). | |
Table 1 Ranges and averages (in brackets) of DGT-labile and 0.45 µm-filtered metal (F) concentrations in Darwin Harbour
|
Co/ng l−1 |
Cu/ng l−1 |
Cd/ng l−1 |
Pb/ng l−1 |
Fort Hill Wharf (FHW) April 2002.
Darwin Harbour near pristine sites 1997–2000.10
|
FHWa DGT-labile |
18–22 (20) |
105–155 (126) |
9–11 (10) |
24–29 (26) |
FHWa F |
13–25 (20) |
208–344 (255) |
7–16 (10) |
11–42 (19) |
Darwin Harbourb F |
5–48 (25) |
151–521 (281) |
3–11 (6) |
<2–21 (9) |
Due to the relatively high detection limit for Zn for some of the short deployments during this experiment (3 h) only Mn, Co, Cd, Cu and Pb data will be considered here. Fig. 2a and b shows a comparison of DGT-labile and 0.45 µm-filtered concentrations following metal addition and during subsequent adsorption of metal to SPM. The DGT-labile metal concentrations in the experimental container prior to metal addition were (in ng l−1): Mn = 580, Co = 38, Cu = 313, Cd = 19 and Pb = 19. The mineralogical composition and grainsize distribution of the SPM used in this experiment (Table 2) were typical of muddy bottom sediments from a range of north Australian estuaries.17
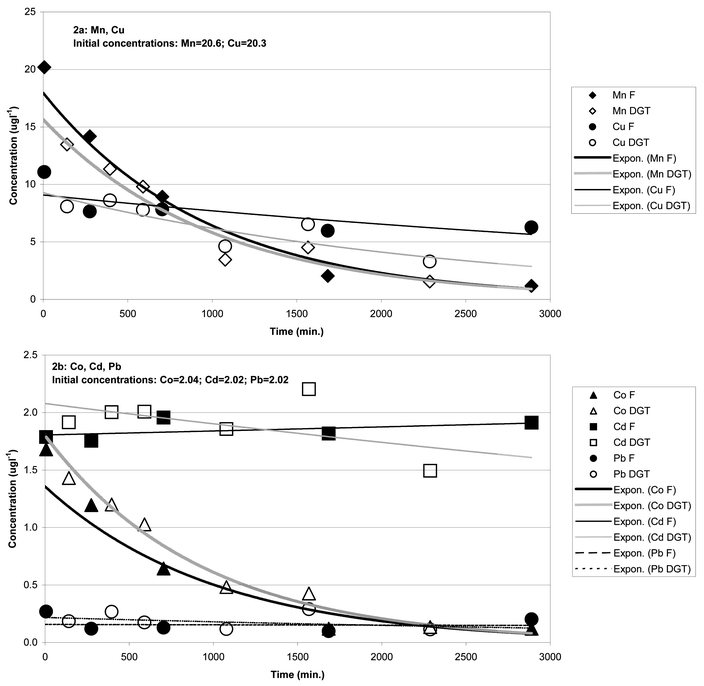 |
| Fig. 2 Concentrations of DGT-labile (DGT) and 0.45 µm-filtered (F) metals in adsorption experiment following metal addition (at 0 min). Note that DGT data are plotted at the mid point of the deployment period. Exponential trendlines fitted using Microsoft® Excel 2000. | |
Table 2 Mineralogy and primary grainsize distribution (dispersant added) of Darwin Harbour SPM
Mineralogy |
% wt. (±1 SD) |
Quartz |
16 (1) |
Smectite |
49 (3) |
Kaolin |
22 (3) |
Mica |
9 (2) |
Calcite |
2 (1) |
Aragonite |
2 (1) |
Anatase |
<1 |
Grainsize distribution |
Diameter/µm |
10th percentile |
3.3 |
50th percentile |
10.3 |
90th percentile |
39.4 |
Discussion
Zhang and Davison4 showed that metals complexed with strongly binding organic ligands have retarded diffusion rates in DGT and might be too large and/or not sufficiently labile for measurement by DGT. Furthermore, these authors found that under such conditions the DGT technique measures similar labile fractions as anodic stripping voltametry techniques. The importance of metal–organic complexation varies in aquatic environments and is, among other factors, determined by the type and concentration of organic ligands.18 However, in general, organic Cu complexes are among the strongest and most abundant in seawater, while those of Pb are of intermediate strength and abundance and those of Co and Cd are relatively weak and less abundant.18–21 Although a comparison of time-integrated DGT-labile data with 0.45 µm-filtered data from grab-samples is uncertain it may assist in validating DGT data. At Fort Hill Wharf the DGT labile metal levels were similar to 0.45 µm-filtered levels for Co, Cd and Pb, whereas the DGT labile Cu concentration was substantially less than the 0.45 µm-filtered Cu concentration (Table 3). The DGT labile/0.45 µm-filtered Cu concentration ratio varied from 0.44–0.63. In comparison, one recent study9 of US coastal waters found that DGT-labile Cu always exceeded inorganic Cu and also included a fraction of organically complexed Cu. In that study the average DGT-labile/0.2 µm-filtered Cu ratio varied from 0.14–0.38 between different areas. No other previously published study appears to have compared DGT with other metal speciation techniques in natural seawater. However, Donat et al.,22 using a Chelex resin partitioning technique with an analytical time scale of 2–3 s (compared to a DGT-APA time scale of a few minutes4,16), found a similar resin-labile/0.45 µm-filtered ratio of 0.28 in South San Francisco Bay. The ratios of DGT-labile to 0.45 µm-filtered Co and Cd were close to unity at Fort Hill Wharf, which qualitatively is in agreement with the expected low organic complexation strength for these metals18,20,21 and the low complexing capacity of Darwin Harbour seawater as indicated by ASV metal titration experiments.23 The Fort Hill Wharf DGT-labile/0.45 µm-filtered Pb ratio was highly variable including one unrealistic value ≫1 (Table 3). A possible explanation for this variation is the high ratio of labile particulate to 0.45 µm-filtered metal for Pb compared to the other metals determined in this study (Fig. 1a and b). Relatively small changes in this distribution may have caused significant short-term fluctuations in 0.45 µm-filtered and/or DGT-labile Pb rendering a comparison of time-integrated and grab-samples misleading.
Table 3 Ratios of DGT-labile and 0.45 µm-filtered (F) metal concentration at Fort Hill Wharf
Period (3 day deployment) |
SPM/mg l−1a |
Co (DGT/F) |
Cu (DGT/F) |
Cd (DGT/F) |
Pb (DGT/F) |
SPM inferred from turbidity data logged at 20 min intervals and averaged over the deployment period (turbidity = 0.949 × SPM + 8.35).
|
1 |
7.9 |
0.95 |
0.45 |
1.06 |
1.21 |
2 |
3.0 |
1.00 |
0.63 |
0.77 |
0.89 |
3 |
6.2 |
1.22 |
0.44 |
1.18 |
1.07 |
4 |
17.1 |
0.98 |
0.53 |
1.29 |
1.71 |
Average |
|
1.04 ± 0.12 |
0.51 ± 0.09 |
1.08 ± 0.22 |
1.22 ± 0.35 |
Relationships between measured metal levels and turbidity at Fort Hill Wharf were evaluated by correlation analysis. For Co, Cu, Cd and Pb there were no significant relationships between DGT-labile metal levels and turbidity (Fig. 1a and b). In contrast, Cu (R2
= 0.791) and Pb (R2
= 0.786), but not Cd, in unfiltered water samples showed strong direct linear relationships with turbidity (SPM). This indicates that when integrated over a 3 day period, DGT-labile metals were not significantly influenced by exchange reactions with the labile portion of metals adsorbed to particulate matter. Furthermore, the uniform DGT-labile levels obtained irrespective of turbidity level suggest that elevated SPM levels did not impact on the operation of the DGT devices through fouling of the membrane filter and/or diffusive gel as found in one other study24 during 30 day deployments.
The metal adsorption experiment in high-SPM seawater served to expand the relatively narrow range of DGT-labile metal levels obtained during the Fort Hill Wharf field trial. Large proportions of labile Pb and Cu were adsorbed to SPM within minutes, Mn and Co adsorption was slower and prolonged and for Cd almost no adsorption occurred (Fig. 2a and b). LogKD values [KD
=
(mass of particulate metal/mass total particulate)/(mass dissolved metal/volume water)]25,26 calculated at 2889 min after metal addition (Pb = 5.52 > Co = 5.20 ≈ Mn = 5.19 > Cu = 4.92 > Cd = 3.49) are within the ranges previously reported for north Australian coastal seawater.10 The high level of metal-ion addition in the experiment significantly exceeded the complexing capacity of dissolved ligands23 and the concentration of strongly bound metal–organic complexes would have been negligible throughout the experiment. This is reflected in the similarity of DGT-labile and 0.45 µm-filtered metal levels measured during the progression of the experiment (Fig. 2a and b). The ratios of DGT-labile and 0.45 µm-filtered concentrations integrated over the duration of the experiment following metal addition were close to unity: Mn = 0.79, Co = 1.04, Cu = 0.84, Cd = 1.04 and Pb = 1.16. The small deviations from unity are probably not significant given the uncertainty in comparing time-integrated data with data based on grab samples. The similarity of data obtained by two independent procedures is a further indication that the operation of DGT devices was not adversely affected through fouling by SPM.
Conclusion
Combined field and experimental data have shown that DGT devices provided adequate detection limits, accuracy and precision for monitoring of near-pristine levels of labile Mn, Co, Cu, Cd and Pb in north Australian coastal seawater when deployed for periods of 3 days. The DGT-labile proportions of 0.45 µm-filtered Co, Cu and Cd agreed qualitatively with data from other areas. Elevated levels of suspended particulate matter did not adversely affect DGT performance.
Acknowledgements
Françoise Foti and Yasmin Antwertinger are thanked for their invaluable assistance in the laboratory. A Northern Territory University Project Grant and Australian Research Council funding for the ICPMS laboratory are gratefully acknowledged.
References
- W. Davison and H. Zhang, Nature (London), 1994, 367, 546 CrossRef CAS.
-
P. G. C. Campbell, in Metal Speciation and Bioavailability in Aquatic Systems, John Wiley & Sons, Chichester, UK, 1995, p. 45 Search PubMed.
- ANZECC, Australian and New Zealand Guidelines for Fresh and Marine Water Quality, Australian and New Zealand Environment and Conservation Council, Canberra, 2000 Search PubMed.
- H. Zhang and W. Davison, Anal. Chem., 2000, 72, 4447 CrossRef CAS.
-
H. Zhang, W. Davison and P. Statham in 4th International Symposium on the Geochemistry of the Earth's surface, International Association of Geochemistry and Cosmochemistry, University of Leeds , 1999, vol. 4, p. 138 Search PubMed.
- S. Denney, J. Sherwood and J. Leyden, Sci. Total Environ., 239, 71 Search PubMed.
- M. C. Alfaro-De la Torre, P.-Y. Beaulieu and A. Tessier, Anal. Chim. Acta, 2000, 418, 53 CrossRef CAS.
- M. R. Sangi, M. J. Halstead and K. A. Hunter, Anal. Chim. Acta, 2002, 456, 241 CrossRef CAS.
- M. R. Twiss and J. W. Moffett, Environ. Sci. Technol., 2002, 36, 1061 CrossRef CAS.
- N. C. Munksgaard and D. L. Parry, Mar. Chem., 2001, 75, 165 CrossRef CAS.
- User's guide to DGT technique, DGT Research Ltd., http://www.dgtresearch.com.
-
Port Darwin 1:25000 Marine Chart, Hydrographic Services, Royal Australian Navy, 1989 Search PubMed.
- J. Gimpel, H. Zhang, W. Hutchinson and W. Davison, Anal. Chim. Acta, 2001, 448, 93 CrossRef CAS.
- E. Wolanski and R. J. Gibbs, J. Coastal Res., 1995, 11, 754 Search PubMed.
- G. J. Batterham, N. C. Munksgaard and D. L. Parry, J. Anal. At. Spectrom., 1997, 12, 1277 RSC.
- H. Zhang and W. Davison, Anal. Chem., 1995, 67, 3391 CrossRef CAS.
-
N. C. Munksgaard, K. Lim and D. L. Parry, Estuarine, Coastal Shelf Sci., 2002, in press Search PubMed.
-
F. M. M. Morel, Principles of Aquatic Chemistry, Wiley, New York, 1988, pp. 266–293 Search PubMed.
- D. J. Mackey and H. W. Higgins, Sci. Total Environ., 1988, 75, 151 CrossRef CAS.
- P. B. Kozelka and K. W. Bruland, Mar. Chem., 1998, 60, 267 CrossRef CAS.
-
F. Millero, Geochem. Trans., 2001, 8 Search PubMed.
- J. R. Donat, K. A. Lao and K. W Bruland, Anal. Chim. Acta, 1994, 284, 547 CrossRef.
-
N. C. Munksgaard and D. L. Parry, unpublished work.
- J. A. Webb and M. J. Keough, Mar. Pollut. Bull., 2002, 44, 222 CrossRef CAS.
- P. W. Balls, Neth. J. Sea Res., 1988, 22, 213 Search PubMed.
- G. Benoit, S. D. Oktay-Marshall, A. Cantu II, E. M. Hood, C. H. Coleman, M. O. Corapcioglu and P. H. Santschi, Mar. Chem., 1994, 45, 307 CrossRef CAS.
|
This journal is © The Royal Society of Chemistry 2003 |