DOI:
10.1039/A802099J
(Review Article)
Chem. Soc. Rev., 2001,
30, 26-35
Structural information from ion mobility measurements:
applications to semiconductor clusters
Received 14th August 2000
First published on 21st December 2000
Abstract
Ion mobility measurements are one of the few methods presently
available that can directly probe the structures of relatively large
molecules in the gas phase. Here we review the application of ion mobility
methods to the elucidation of the structures of semiconductor clusters
(Sin, Gen, and
Snn). We describe the new high-resolution
implementation of the technique and the advanced methods of mobility
calculations that are crucial for the correct analysis of the experimental
data.
Alexandre A. Shvartsburg |
Alexandre A. Shvartsburg was born in Moscow, Russia. He received an MS from
the University of Nevada, Reno in 1995 and a PhD from Northwestern
University in 1999. He is currently a Postdoctoral Fellow at York
University in Canada. |
Robert R. Hudgins |
Robert R. Hudgins was born in New Jersey, USA and obtained his
undergraduate education from the College of William and Mary in
Williamsburg, Virginia. He received his PhD from Northwestern University in
1999, where he worked on the development and applications of high
resolution ion mobility measurements. He currently holds an NSF
International Post- doctoral Fellowship at the University of Basel in
Switzerland. |
Philippe Dugourd |
Philippe Dugourd was born in Angers, France. He obtained his undergraduate
degree from the Ecole Normale Superieure in Paris, and received his PhD
from the University of Lyon. In 1991 he joined the French National Center
for Scientific Research (C.N.R.S.) and since then he has held a permanent
research appointment at the University of Lyon. In 1995 he spent a year as
a visiting scholar in the Chemistry Department at Northwestern
University. |
Martin F. Jarrold |
Martin F. Jarrold was born in Haslemere, Surrey. He obtained his
undergraduate and graduate degrees from the University of Warwick, and then
went to the University of California, Santa Barbara as a NATO Postdoctoral
Fellow. In 1985 he joined the Physics Research Division of AT&T Bell
Laboratories. Since 1992 he has been a Professor in the Chemistry
Department at Northwestern University. |
1 Introduction
It is difficult to understand anything without information about its
structure. While this statement is probably true in all areas of science,
it is particularly valid in the chemical sciences where understanding of a
molecular system almost always hinges on elucidation of its structure. In
the gas phase, high resolution spectroscopy has provided remarkably
detailed structural information for small molecules. However, current
interest is focused more on much larger and more complex systems, for
example, atomic clusters and anhydrous biomolecules. The traditional
spectroscopic methods often fail for these complex systems. And the other
structural tools available to chemists, such as NMR and X-ray diffraction,
cannot be used because the sample densities are too low. In this article,
we describe the development of high-resolution ion mobility methods as a
structural tool and the application of this approach to the
characterization of gas phase atomic clusters,1 specifically semiconductor clusters. Our interest
in the structures of these species stems from a desire to understand how
the geometries of small particles containing tens of atoms evolve into the
bulk crystal structure. The methods outlined here have also been applied to
relatively large biomolecules, such as peptides and proteins, but we do not
describe that work here.22 Ion mobility measurements
The mobility of an ion is a measure of how rapidly it moves through a
buffer gas under the influence of an electric field.3 In the low field limit (see below) the mobility of
a cation or anion depends on its orientationally-averaged collision
integral with the buffer gas, which in turn depends on the ion’s
geometry. Ions with open geometries undergo more collisions with the buffer
gas and hence travel more slowly than compact ions. Thus ion mobilities
provide a way to characterize an ion by its physical size and this property
has been exploited by analytical chemists.4
For example, the portable chemical warfare agent detectors currently used
by the US Army and NATO are ion mobility spectrometers that operate under
ambient conditions (the mobilities are measured in air). Ion mobility
measurements have been used by physical chemists for many years to
characterize ion–molecule interactions.3 These measurements are usually performed under
well-defined conditions on instruments that incorporate mass spectrometers
to identify the ion. Mobility measurements have also been used to determine
particle size distributions.5 That
structural isomers of the same ion can have different mobilities was first
demonstrated by Carr,6 though it was the
work of Bowers and collaborators which really showed the potential of
mobility measurements as a structural probe. Their studies of carbon
clusters confirmed the presence of chains, monocyclic rings, and fullerenes
and revealed a variety of polycyclic ring isomers.7 A number of new, and more exotic, carbon cluster
isomers have now been observed and assigned, including ‘fullerene
dumbells’ (fullerenes linked by a carbon chain) and
‘tadpoles’ (a chain attached to a carbon ring).8 Furthermore, annealing studies have shown that
carbon rings can be converted into fullerenes, providing the basis for the
most plausible mechanism of fullerene synthesis.9 Mobility measurements have also been applied to a
variety of metal-containing carbon clusters, salt clusters, and metal
clusters in addition to the clusters of the Group 14 elements discussed
here.103 Experimental methods
Mobility measurements are usually performed in a drift tube.3 The drift tube contains the buffer gas and
provides a uniform electric field for the ions. Experiments intended to
deduce structural information from ion mobility measurements must be
performed under well defined conditions, where the mass of the drifting ion
is known and the buffer gas does not contain species that can cluster with
the drifting ions. Two basic experimental configurations have been
employed: the injected ion drift tube (which usually has a buffer gas
pressure <10 Torr)11 and the high
resolution configuration (with a buffer gas pressure of hundreds of
Torr).12,13 In the injected ion drift
tube approach, ions (often mass-selected) are injected into a drift tube
through a small aperture. After traveling across the drift tube, some of
the ions exit through another aperture. They are then mass analyzed and
detected. Mobilities are measured by injecting a short packet of ions and
recording how long it takes for them to reach the detector. If the injected
packet is sufficiently narrow, the width of the ion packet at the detector
is limited by diffusional broadening as it travels through the drift tube
(the ion densities are usually low enough that space charge effects are
negligible in these experiments). The resolving power is then given by
eqn. (1)14 | 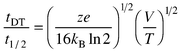 | (1) |
where tDT is the drift time, t1/2
is the width of the peak at half height, ze is the charge on the
ion, V is the voltage drop across the drift tube, and T
is the temperature. The resolving power is increased by raising V
or lowering T. The energy that the ions gain from the drift field
is determined by E/N, where E is the drift field
and N is the buffer gas number density. In the low field regime,
the drift velocity is much less than the average relative velocity between
the ion and a buffer gas atom and the mobility (defined by K =
vD/E) is independent of the field. In the high
field regime, the mobility depends on the drift field. It is desirable to
keep the drifting ions in the low field regime for several reasons, in
particular it greatly simplifies the interpretation of the results. So if
V is increased to enhance the resolution, N must be
raised to keep the ions in the low field regime. However, the pressure in
the drift tube cannot be increased too much above 10 Torr because then it
is no longer possible to inject intact ions into the drift field. As the
ions enter the drift tube they undergo collisions with the buffer gas which
converts some of their kinetic energy into internal energy. As the buffer
gas pressure is increased, higher injection energies are required and the
ions are collisionally heated to the point where they dissociate. In our
injected ion drift tube apparatus, cluster
ions are generated by pulsed laser vaporization, and then mass selected by
a quadrupole mass spectrometer before being injected into the drift tube.
The drift tube is 7.6 cm long and operated with a buffer gas pressure of
3–10 Torr and a drift voltage of 100 V. This yields a resolving power
of around 10. Ions that exit the drift tube are mass analyzed by a second
quadrupole mass spectrometer, and then detected by an off-axis collision
dynode and dual microchannel plates.The high resolution configuration is conceptually similar to that
employed in ion mobility spectrometers developed for analytical
applications. Our high resolution apparatus is shown schematically in
Fig. 1.12 It
consists of a source region directly attached to a 63 cm long drift tube
and the whole assembly operates at a pressure of around 500 Torr. At the
end of the drift tube the exiting ions are analyzed by a quadrupole mass
spectrometer and detected. Cluster ions are generated by pulsed laser
vaporization and drawn into the drift tube region of the instrument by
electric fields. Neutral species are prevented from passing from the source
region into the drift tube by the ion gate which consists of a stack of
electrodes with 5 mm apertures. An electric field draws the ions through
the ion gate while a counter flow of buffer gas stops the neutrals. In the
drift tube, a uniform electric field is provided by a stack of electrodes
connected to a voltage divider. The drift voltage is 10000–15000 V.
This provides a resolving power of >100, an order of magnitude
improvement over that obtained in the injected ion drift tube
configuration. The drift voltage is limited by electrical breakdown.
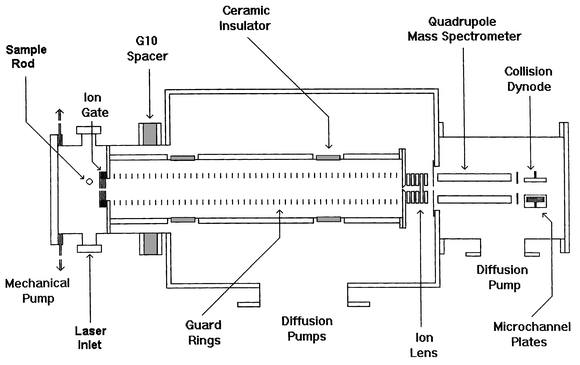 |
| Fig. 1 Schematic diagram of the high-resolution ion mobility apparatus. | |
In many respects the injected ion drift tube and the high resolution
configuration are complementary. With the ability to inject ions at
controlled energies, and with mass selection before and after the drift
tube, the injected ion drift tube can be used to perform studies beyond
simple mobility measurements. For example, isomer-specific reactivities
with molecules diluted into the buffer gas can be examined.11 By varying the injection energy one can examine
the interconversion between different structural isomers.10 Here, some of the ion’s injection energy
is converted into internal energy as it enters the drift tube. Once in the
drift tube the cluster cools by further collisions with the buffer gas.
This heating–cooling cycle anneals a cluster into the geometry that
is thermodynamically preferred at the energy where the isomer populations
are frozen-in (this is not necessarily the global minimum). By raising the
injection energy further the ions become hot enough to dissociate and one
can estimate dissociation energies and identify the fragmentation
products.15
Another important feature is the ability to change the temperature of
the drift tube. Temperature-dependent measurements provide information
about the ion–buffer gas interaction potential and enables one to
study the processes of thermally-induced dissociation and isomerization.
Information on liquid–solid phase transitions has also recently been
obtained (see below). The small drift tube used in the injected ion drift
tube apparatus is readily cooled down to <80 K by liquid nitrogen flow
or resistively heated to >500 K. The temperature of the large drift tube
in the high-resolution apparatus can be varied over a narrower range around
300 K. With the high resolution apparatus it is possible to follow cluster
isomerization processes that occur as the ions travel through the drift
tube. Isomerization rates can be obtained by varying the drift time (over
the range 0.1–1.0 s) and Arrhenius activation parameters can be
derived if these measurements are performed as a function of
temperature.10
4 Mobility calculations
Structural assignments for unknown species observed in mobility
measurements become possible by comparison of the measured mobilities with
mobilities computed for reasonable candidate geometries. In general, the
mobility of a rigid ion undergoing elastic collisions with a gas depends on
a series of collision integrals, Ω(l,s), which
characterize the ion and buffer gas combination. In the Rayleigh limit
where m (the mass of the ion) >> mb (the
mass of a buffer gas atom) the series of collision integrals reduces
essentially to a single one,
Ω(1,1) (see below). Since helium is the buffer
gas of choice, this approximation is almost always valid. Another crucial
simplification is achieved by operating in the low field limit where the
relative velocity distribution of the ion and buffer gas is essentially
thermal at the buffer gas temperature. This ensures that there is no
alignment of the drifting ion and so the effective collision integral
equals the orientationally averaged quantity Ω(1,1)avg
. In the low field limit, the mobility is proportional to the diffusion
constant and can be calculated from eqn.
(2)14 |  | (2) |
where ρ is the buffer gas number density. Evaluating eqn. (2) reduces to calculating
Ω(1,1)avg
. For a monatomic ion with a centrally symmetric potential,
Ω(1,1)avg
can be calculated analytically for many simple potential forms. For
polyatomic ions, collisions can exchange energy between the rotational and
translational degrees of freedom. The way to account for this using the
rigid rotor approximation has been worked out for diatomic ions.16 A general solution has not been attempted, but,
calculations indicate that the effect should be small (on average) for the
large ions considered here, because of the huge disparity between their
moments of inertia and the impulses provided by the collisions of light
helium atoms at typical room temperature velocities.Ignoring rotational–translational coupling, the collision integral
is obtained by integrating the momentum transfer cross-section over the
distribution of relative velocities between the buffer gas atom and the
ion. The momentum transfer cross-section (for a particular collision
geometry) is obtained by averaging a function of scattering angle
χ over the impact parameter beqn. (3):
| 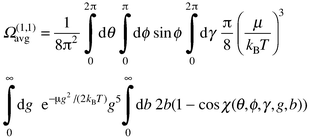 | (3) |
where
μ is the reduced mass,
g is the relative
velocity, and the collision geometry is defined by angles
θ,
phis, and
γ.
Eqn. (3) cannot be solved analytically and even a
numerical solution is difficult. The simplest approximation is to draw a
closed surface around the ion to mark the hard sphere collision distance
and to assume that the cross-section in each orientation is equal to the
projection of that surface on a plane (the ‘projection
approximation’). This reduces
eqn. (3)
to
eqn. (4):
| 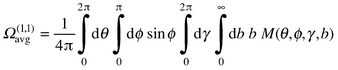 | (4) |
where
M is unity when a hard-sphere impact occurs for a collision
geometry defined by
θ,
phis,
γ, and
b and zero otherwise. It is interesting to note that this type of
model was first used in 1925 when it was employed to deduce structural
information from diffusion constant measurements (another transport
property which is closely related to the low field mobility). At that time
eqn. (4) was evaluated by constructing a
scale model of the candidate geometry out of bees wax, manually determining
the size of the shadow it projects, and then averaging over different
orientations. Now,
eqn. (4) can easily be
solved numerically on a computer. Each atom in the ion is represented by a
sphere with a radius given by the sum of the helium atom radius and the
radius of the atom in the ion. Since these collision radii are not
precisely defined, they are adjusted within a narrow range to fit the
measured collision integrals.
Substantial
internal inconsistencies have been found with the projection approximation.
For example, when the collision radius is set to reproduce the mobilities
of fullerenes, the calculated mobilities for carbon chains deviate from
experiment by more than 20%. Nevertheless, the projection approximation was
successfully used to assign features observed in the early studies of
carbon clusters. This is partly because the carbon cluster isomers
separated in the early studies have very different shapes and hence very
different mobilities, and partly because it was already known what isomers
to expect (for example, chains and then rings were expected for the small
clusters, and fullerenes were expected for the larger ones) so even a
fairly crude mobility calculation was enough for a successful structural
assignment. However, it is now clear that the projection approximation is
inadequate for many problems. And its inadequacy is compounded by the
development of improved experimental techniques which permit the resolution
of isomers with mobilities that differ by less than 1%.
While it is obvious that the projection approximation neglects all
long-range attractive interactions between the ion and buffer gas atoms, it
is less obvious that it also ignores all the details of the scattering
process. For a body made entirely of convex surfaces the effects of
scattering (with a hard sphere potential) are not important and the
collision integral evaluated from the scattering angles is equal to that
obtained from the projection approximation.17 However, when atoms are scattered on bodies with
concave surface some of the trajectories experience multiple collisions.
The collision integral calculated from the scattering angles then exceeds
that obtained from the projection approximation.17 While all molecular surfaces have concave parts
because of local surface roughness, this typically only results in a few
percent difference between the collision integral and the projection.
However, for objects with grossly uneven surfaces or cavities, differences
of up to 25% have been found.17 The exact
hard-spheres scattering (EHSS) model was developed to account for multiple
scattering within the framework of a hard sphere potential.17 Like the projection approximation, the ion is
treated as a collection of hard spheres and the collision radius is
adjusted to reproduce the measured mobilities. While this model is a
significant improvement over the projection approximation, it still ignores
all long-range interactions between the ion and buffer gas.
The long-range interactions are incorporated by propagating classical
trajectories between buffer gas atoms and the target ion in a realistic
intermolecular potential.18 The scattering
angle, χ, is determined from the trajectory and eqn. (3) is solved numerically. In the work
performed so far, the potential between the ion and buffer gas atom is
assumed to consist of two parts: van der Waals and charge-induced dipole
interactions. The first term is modeled as a sum of pair-wise
Lennard–Jones (6–12) interactions between the buffer gas atom
and each atom in the ion. The Lennard–Jones parameters (the depth
ε and σ, the distance where ε
is zero) are obtained by fitting measured mobilities as a function of
temperature. The charge-induced dipole term was initially evaluated
assuming that the charge was uniformly delocalized over all atoms. The
charge distribution obtained from the first-principles calculations is now
usually employed. In most cases, the charge distribution makes a noticeable
(>1%) difference only at low temperatures. However, a highly
non-symmetric charge distribution has significant consequences at room
temperature.8 Calculating the mobility by
the trajectory method is time consuming, requiring tens of hours on a
workstation for the clusters considered here.
In the three methods described above—the projection approximation,
the exact hard spheres scattering model, and the trajectory
method—the clusters are represented through the position of atomic
nuclei. In reality, the buffer gas atoms interact with ions via
their electronic wave functions, thus the mobility actually characterizes
not the nuclear geometry of an ion, but the electron density distribution.
Ideally, this distribution should be converted into a potential which can
then be used for trajectory calculations. Such an approach has not yet been
employed. However, the electron density distributions have been introduced
within the framework of a hard sphere potential in a model that employs
scattering on electron density isosurfaces (SEDI).19 In this approach, the cluster is represented by
a surface defined numerically as a set of points in space where the
calculated electron density assumes a certain value. This value is adjusted
to fit the measured mobilities. The collision integral is evaluated by
scattering buffer gas atoms on the isosurface. Like the EHSS model, this
treatment obviously ignores the long-range ion–buffer gas
interactions that are accounted for by the trajectory method. It is,
however, superior to the trajectory method in some respects for negative
ions. Anions often have mobilities which are systematically smaller than
the mobilities of the corresponding cations. This difference appears to be
related to the electron density of the anions extending further than for
the corresponding cations. Models where the clusters are represented
through the position of their atomic nuclei (EHSS and the trajectory
method) often fail to accurately reproduce the mobilities of anions,
presumably because of the difficulty of representing the spill-out of the
electron density. However, the SEDI model, which is based on calculated
electron densities, can account for the differences between the mobilities
of anions and cations.
5 Semiconductor clusters: an overview
Clusters of the semiconducting elements (silicon and germanium) are of
interest for both scientific and technological reasons. They are
fascinating scientifically because they assume geometries that have nothing
in common with the packing of the corresponding bulk solids. They are
important technologically because with the continuing miniaturization of
electronic devices, minimum device features will eventually approach the
cluster size regime. This makes understanding the properties of
semiconductor clusters of critical importance. Under ambient conditions tin
(which is below germanium in the periodic table) is a metal (white tin).
However, it appears that tin clusters adopt geometries similar to those
found for silicon and germanium clusters rather than those expected for a
metal cluster. Bulk tin has another allotrope (grey tin) that is a
semiconductor with the same crystal structure as silicon and germanium, but
it is only stable at low temperatures. Because tin clusters adopt
geometries that are similar to those found for silicon and germanium
clusters, our findings for tin clusters are included here. Tin clusters are
of particular interest because ion mobility measurements also indicate that
they have melting points that are higher than the bulk material (clusters
are normally expected to have depressed melting points). Finally, we also
touch upon results for lead clusters, to contrast them with the species of
lighter Group 14 elements.For the reasons described above, silicon clusters in particular have
attracted an intense research effort, and there has been a lot of
speculation about their structures. For example, the prevalence of
Si10 and Ge10 fragments in the dissociation of
Sin and Gen anions and
cations20 was interpreted as evidence for
various types of packing in larger Sin and
Gen clusters, and the low reactivity of
Si13+ with C2H4,
NH3, O2, and H2O21 has been
taken to indicate icosahedral packing. However, the preference for certain
fragments appears to reflect their thermodynamic stability rather than the
presence of particular structural units, and calculations for silicon
clusters consistently show the icosahedral geometry to be extremely high in
energy and not even stable as a local minimum. As we will describe below,
most of our current knowledge about the geometries of medium-sized atomic
clusters has been gained from ion mobility measurements.
There have been a number of spectroscopic investigations of small
silicon and germanium clusters. The geometries of some size-selected,
matrix-isolated Sin (n
≤ 7) have been
determined by Raman and IR spectroscopy.22
Structural assignments were made by comparing the measured vibrational
spectrum to those calculated for a variety of low energy geometries. The
structures of some Sin (n
≤ 7) and
Gen (n
≤ 4) clusters have also been
elucidated using vibrationally-resolved anion PES.23 Vibrational resolution could not be achieved for
larger cluster sizes, however the structures of
Si10−, Ge7−, and
Ge10− have been assigned on the basis of their
electronic band patterns.
Optimization of small Sin using ab
initio24 and density functional theory
(DFT)25 methods yields highly coordinated
structures which are favored over bulk-like fragments because they have
fewer dangling bonds. The global minima are a triangle for n = 3,
a rhombus for n = 4, trigonal, tetragonal, and pentagonal
bipyramids for n = 5, 6, and 7 respectively, and a distorted
bicapped octahedron for n = 8. Note the transition to
three-dimensional geometries at n = 5. The geometries for
n
≤ 7 have been confirmed by vibrational spectroscopy. For
n = 9 and 10, structures produced by capping an octahedron and a
trigonal prism are both low in energy. For Si10 the tetracapped
trigonal prism is now accepted as the global minimum. However, the global
minimum for Si9 is neither of the above but a capped
Bernal’s structure (a stack of two rhombuses with a capping atom on
top).26 Searches for ground state
geometries for n
≥ 11 using first-principles energy evaluations
have been less extensive. For n = 11–13 geometries based on
further capping of the trigonal prism unit have been considered.27,28 The results of unbiased geometry
searches for these and larger silicon clusters will be described below.
6 Ion mobility measurements for silicon cluster
cations
Fig. 2 shows drift time distributions
measured for Si27+ and
Si28+ using an injected ion drift tube apparatus (low
resolution) and the new high resolution apparatus. The drift time
distribution shows the amount of time it takes for the ions to travel
through the drift tube, and each peak represents a different structural
isomer. With the dramatic improvement in the resolving power many more
structural isomers have been resolved. The distribution for
Si27+ is dominated by two main isomers while that for
Si28+ is dominated by one. Fig.
3 shows a plot of the inverse reduced mobilities of the main
features resolved in the drift time distributions for silicon cluster
cations, Sin+, with n =
4–60.29 The dominant feature in the
drift time distribution for each cluster size is represented by the filled
point while other clearly resolved features are represented by open points.
The reduced mobility is the measured mobility normalized to the buffer gas
number density at STP, while the inverse reduced mobilities are plotted
here because they are proportional to the collision integral of the ion
with the buffer gas. The systematic increase in the inverse mobilities
apparent in Fig. 3 results from the increase
in the physical size from adding more atoms to the cluster. The break in
the mobilities at around n = 24–34 indicates a structural
transition to more compact geometries (both exist in the transition
region). Crude estimates suggested that the clusters undergo a transition
from prolate growth to near-spherical geometries.21 However, unlike carbon clusters where it was
known what geometries to expect, for silicon the geometries were largely
unknown. The results of theoretical studies for silicon clusters with up to
around 11 atoms were described above. The problems associated with
extending these studies to larger cluster sizes are well known: first, as
the cluster becomes larger the time required for a single energy
calculation usually increases as a fairly high power of the number of
atoms; and second, there is more phase space to search before one can be
sure that the lowest-energy geometry has been found. Many reports on the
structural optimization of silicon clusters have been published over the
last decade. Each one presenting geometries lower in energy than those
previously known. The major problem in these studies is not so much finding
a lower-energy geometry, but knowing when the lowest-energy geometry is
found and hence when to stop looking. The strategy that we have adopted is
to compare properties of the calculated geometries to those that have been
measured, and to use agreement between the measured and calculated
properties as a criteria for identification of the global minimum. Not
surprisingly, the most important piece of experimental information employed
in these studies is the mobility. While a mobility measurement alone
usually cannot uniquely identify a particular geometry (many different
geometries can have the same mobility) the criteria that the calculated
geometry be low in energy and have a mobility that fits the measured
mobility is often sufficient to uniquely identify the ground state. Another
important piece of experimental data employed in these studies is the
dissociation energies. Agreement with the measured dissociation energies as
a function of size indicates that the search procedure has, at least, come
close to finding the global minimum.30
Ionization energy measurements,31 electron
affinities, and fragmentation patterns have also been used to confirm the
structural assignments described below.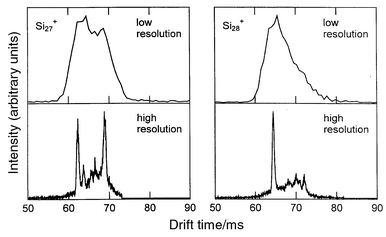 |
| Fig. 2 Drift time distributions measured for Si27+ and
Si28+ with the injected ion drift tube apparatus (low
resolution) and with the high resolution apparatus. | |
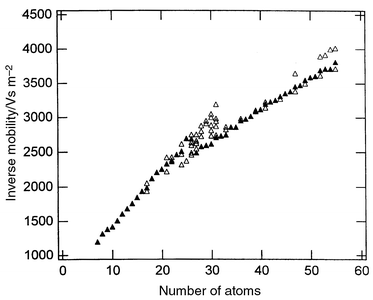 |
| Fig. 3 Plot of the inverse reduced mobilities for silicon cluster cations,
Sin+, n = 4–60. The dominant
feature in the drift time distribution for each cluster size is represented
by the filled point while other clearly-resolved features are represented
by open points. | |
The geometries for Sin, n = 10–20,
were found by Ho and collaborators32
primarily through an unbiased global search using simulated annealing and a
genetic algorithm coupled with a tight-binding potential. Promising
candidates were re-optimized with DFT. Simulated annealing had repeatedly failed to locate
the lowest-energy geometries for n > 12, so the use of a
genetic algorithm was critical. The geometries for cations and anions were
found by relaxing a number of low-energy neutral geometries for
each Sin (n
≤ 20) without symmetry
constraints. Additional simulated annealing was performed using the
Car–Parrinello LDA method. For n < 12, a global search
was performed. For n > 12 a local search was initiated from the
geometries of low-energy neutrals with the objective of finding any
distortions that lower the energy. For neutrals, cations, and
anions with n < 11 the accepted geometries were
obtained.19 For larger sizes, the
geometries were substantially lower in energy than any previously described
in the literature. The global minima found for n = 11–18 are
shown in Fig. 4. Geometries based on stacked
Si9 tricapped trigonal prism (TTP) units are prevalent. However,
the geometries of Sin,
Sin+, and
Sin− differ in details for most
n. For n = 19 and 20, near-spherical, cage-like
geometries become competitive with prolate TTP stacks, with the exact
energetic ordering depending on the charge.
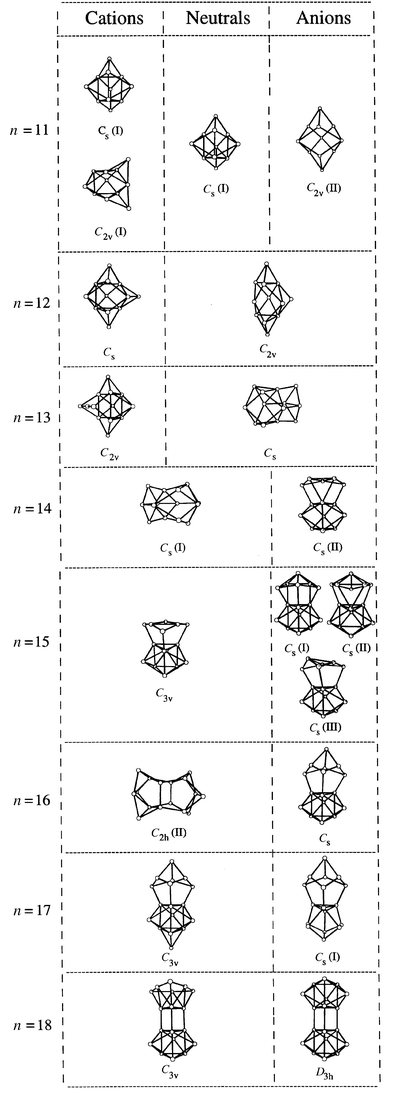 |
| Fig. 4 Global minima (obtained with the Perdew–Wang–Becke 88
gradient corrected functional) for the Sin, n
= 11–18, cations, neutrals, and anions. For
Si15−, the
Cs(I)/Cs(II) minimum that is
believed to be the lowest energy geometry (see text) is also shown.
Multiple entries indicate that the geometries are degenerate to within the
computational error margin (∼ 3 meV atom−1). | |
Fig. 5 shows a comparison of the measured
inverse mobilities for silicon cluster cations (n = 4–18) to
those calculated by the trajectory method for the lowest energy cation
geometries. Since the geometries of smallest clusters (n
≤ 7)
are known, they were used to extract the Lennard–Jones parameters for
the elementary Si–He interactions (ε = 1.38 meV and
σ = 3.47 Å). The calculations employed partial charge distributions obtained from
DFT, although this made only a small difference (<1%) to the calculated
mobilities. Except for Si18+ the calculated
mobilities are within 2% of the measured values. This is the generally
accepted error margin (it is a combination of the errors from the
experiment, the calculated bond lengths and angles, and the mobility
calculations). For Si18+, two isomers are resolved in
the high resolution ion mobility measurements. The mobility of the dominant
isomer does not match the mobility calculated for the lowest-energy
geometry found in the DFT calculations. However, the calculated mobility
for the second lowest-energy isomer found (a D3h
geometry) is in excellent agreement with the mobility of the dominant
isomer, while the mobility of the lowest energy isomer is in good agreement
with the mobility of the minor component in the drift time distribution.
Thus DFT may have switched the ordering of these two isomers. It is also
possible that the ground state geometry has yet to be found for this
cluster.
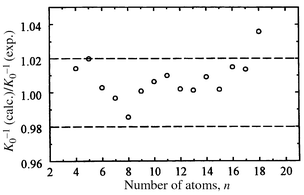 |
| Fig. 5 Comparison of measured inverse mobilities for the dominant features to
those calculated forthe lowest-energy geometries for the
Sin cations with n = 4–18. Dashed lines
delimit the customary 2% error margin. | |
Mobilities calculated for all previously proposed non-TTP based
Sin geometries in the n
≲ 25 range deviate
from the measured values by 5–15%. In particular, the mobility
measurements rule out the stacked alternating triangles,33 the stacked hexagonal rings,34 icosahedron-based geometries, and any geometry
based on the tetrahedral bonding network of bulk silicon. In addition to
agreement with the mobilities, the dissociation energies computed for
Sin (n
≤ 18) cations match the experimental
results, indicating that the search procedure has, at least, come close to
the global minima. The fragmentation channels calculated for
Sin+ global minima also agree with
experiment.
7 The differences between silicon cluster anions
and cations
The mobilities of silicon cluster anions show the same general features
as observed for the cations.29 The addition
of two electrons apparently increases the stability of the prolate isomers
relative to the more spherical ones, so that the structural transition from
prolate to more spherical geometries occurs slightly later for the anions
than for the cations. The inverse mobilities of the anions are also
systematically larger than those for the cations. This shift presumably
results from differences in the exterior electron densities caused by the
addition of the extra electrons. Mobilities calculated for the anions by
the trajectory method do not fit the measured values. The calculated
inverse mobilities increase faster as a function of cluster size than the
measured values. This discrepancy also probably results from the electron
density spill-out. The SEDI (scattering on electron density isosurfaces)
model should be able to account for this effect. Like the exact
hard-spheres scattering (EHSS) model, the SEDI model ignores the long-range
interactions between the ion and buffer gas. However, mobilities calculated
for all the silicon cluster cations by the EHSS model are within 1% of
those calculated by the trajectory method, and so the SEDI model should
work well for the anions. This is quite different from the situation with
carbon clusters where substantial deviations where found when the
long-range interactions were not properly taken into account. One obvious
difference is that silicon clusters have less diverse geometries than
carbon clusters. Silicon clusters with n
≥ 5 all have
relatively compact, three-dimensional structures. In addition, silicon
clusters have longer bond lengths, about 2.5 Å compared to
1.3–1.4 Å in carbon, and for this reason the cumulative
long-range potential for silicon clusters depends less strongly
on the cluster size and shape. Thus a Si–He collision distance, which
incorporates the local environment, is more transferable to different
cluster geometries than for carbon.The mobilities evaluated by the SEDI model for the global minima of the
Sin anions are within 2% of the measurements for all
sizes considered. In particular, the systematic deviations observed with
the EHSS model and the trajectory method are not present. The systematic
deviations result because the mean negative charge per atom is proportional
to 1/n. So the electron spill-out for smaller clusters is more
significant, making them physically ‘larger’. This effect is
neglected in the EHSS model, but accounted for by the SEDI model. In
principle, the same phenomenon should arise for cations, where the mean
positive charge increases with decreasing cluster size. In practice, the
expansion of the anion’s electronic cloud appears to be greater than
the contraction of the cation’s, thus the effect is primarily
manifested for anions.
Fig. 6 shows a plot of the difference
between the inverse mobilities of the Sin anions and
cations (the points are the experimental data for the dominant isomers and
the lines are SEDI calculations). The difference is about 100 Vs
m−2 for most n, but substantial fluctuations
occur for some cluster sizes. The fluctuations result when changing the
charge state causes a reordering of the low-energy isomers and hence a
geometry change. Significant fluctuations are observed for clusters as
large as Si43. The dashed line in Fig.
6 shows mobilities calculated by the SEDI method assuming that the
anions have the same geometries as the cations (except for local
relaxation). The solid line shows the result when the global minima are
used for the anions, except for Si15− (see
below). The minima at n = 9, 15, 16, and 18 indicate that the
anion geometries are more compact than the cation analogs for these
clusters. For Si15− the
Cs(III) global minima does not fit the difference
between the anion and cation mobilities, while that for the second lowest
energy isomer (the Cs(I)/Cs(II)
isomer shown in Fig. 4) does. The
Cs(I)/Cs(II) geometry may be the
global minimum for Si15−.
Note that the mobilities of both the Cs(III) and
Cs(I)/Cs(II) isomers are within 2%
of the measured value for Si15−; it is the
difference in mobilities on going from the cation to the anion that does
not fit for the Cs(III) geometry.
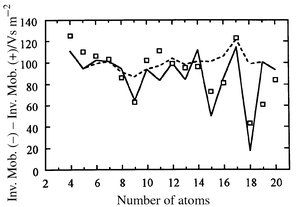 |
| Fig. 6 Differences between the inverse mobilities
(K0−1) for
Sin+ and
Sin–. The points are the measured
values for the dominant isomers and the lines are from SEDI calculations.
The solid line corresponds to the assumption that the clusters in both
charge states adopt their lowest-energy geometries (except that we have
used the Cs (I)/Cs (II) isomer for
Si15−). The dashed line shows the result if the
geometries of the Sin anions were identical to those of
global minima for cations except for local relaxation. | |
The mobility measurements have confirmed (with a few minor adjustments)
the TTP-based structures of medium-sized
Sin+ and
Sin− found by global optimization.
This is a strong indication that the neutral geometries predicted by the
same optimization scheme are also correct. This is supported by ionization
potentials calculated for the Sin global minima which
are in a good agreement with the measurements for all n < 20,
whereas ionization potentials calculated for second-lowest energy
geometries are not in agreement with experiment for many sizes.35
8 Structural characterization of medium-sized
germanium clusters
Bulk germanium and silicon pack in the same diamond lattice and the
geometries of smallest (n
≤ 10) Gen and
Sin clusters appear to be identical by both calculation
and experiment.26 So it was often assumed
that the geometries of silicon and germanium clusters would be identical
except for the 4% difference in the bond lengths. However, mobility
measurements for Gen cations revealed that the
structural transition to more spherical geometries begins at n
∼ 65,36 almost three times the size
where this occurs for Sin+ species. The
size-dependent features in mobilities of
Gen+ and
Sin+ start differing at
n
∼ 15. So in the intermediate size regime the geometries of
silicon and germanium clusters are significantly different and perhaps the
first question to answer is where and how the growth patterns of silicon
and germanium clusters diverge.A geometry search was performed for
Gen and Gen+ with
n
≤ 16 by relaxing an extensive set of low-energy
Sin and Sin+
isomers.37 The structures for
Gen and Sin neutrals first differ
at n = 13, while those for cations diverge one size earlier. The
geometries for Ge14 and Ge14+ are
identical to Si14 and Si14+, but they
diverge again for for n = 15 and 16. A comparison of the
Gen and Sin geometries for
n = 13, 15, and 16 is shown in Fig.
7. For n = 13 and 15 the silicon and germanium geometries
just differ in the orientation of the capping atoms. For n = 16
the difference is more substantial. To test these predictions, mobilities
were calculated and compared with the measured values. The results are
shown in Fig. 8. The filled points are the
lowest-energy Gen+ geometries according to
the DFT calculations of ref. 37. For
n = 12, 13, 15, and 16 the lowest-energy
Gen+ geometries are different from their
silicon analogs and the open points for these clusters are the mobilities
of the lowest- energy silicon geometries relaxed for germanium. For
Ge12+, the open point (the lowest-energy silicon
geometry relaxed for germanium) fits the measured value better than the
filled point (the lowest-energy Ge12+ geometry
according to DFT). Apparently, DFT has reversed the ordering of these
isomers. For Ge13+ the lowest-energy isomer according
to DFT and the lowest-energy silicon geometry relaxed for germanium are
both in good agreement with the measured mobility. However, for n
= 15 and 16 the lowest-energy isomers according to DFT fit the measured
mobilities while the lowest-energy silicon geometries relaxed for germanium
clearly do not. Thus comparison of the measured and calculated mobilities
proves that the growth pathways of Sin and
Gen cations indeed diverge by n = 15. This
conclusion is supported by data on the dissociation energies and the
fragmentation pathways.
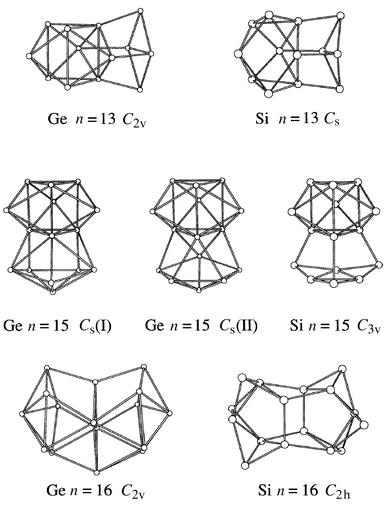 |
| Fig. 7 Lowest-energy geometries according to DFT (using the
Perdew–Wang–Becke 88 gradient corrected functional) for the
Sin and Gen neutrals with
n = 13, 15, and 16. | |
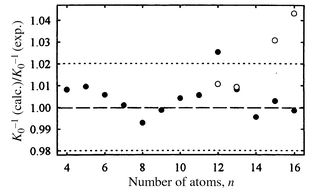 |
| Fig. 8 Relative deviations of the inverse mobilities
(K0−1) calculated for
Gen cations from the measurements. Filled circles are
for the lowest-energy isomers according to DFT. For
Sin+n < 12 and n = 14
the lowest energy germanium geometries are identical to their silicon
analogs. The open points for n = 12, 13, 15, and 16 are the
mobilities of the lowest-energy silicon geometries relaxed for
germanium. | |
9 Tin clusters are like silicon and germanium
clusters
Tin is at the transition between the semiconducting elements above it in
the periodic table, and the metallic elements below. Tin’s normal
allotrope under ambient conditions (β-tin) is a metal with a
body-centered tetragonal lattice, but it also has a semiconducting form
(α-tin) that is more stable below 286 K. α-Tin has the same
diamond lattice as silicon and germanium. The mobilities of
Snn cations have recently been measured up to
n = 68.38Fig. 9 shows a plot of the relative mobilities of
silicon, germanium, and tin clusters. The relative mobility is given by the
measured mobility divided by the mobility of a sphere of bulk density. A
prolate growth pattern is characterized by relative mobilities which
decrease with increasing cluster size. While clusters that retain spherical
geometries have relative mobilities close to 1.0. The relative mobilities
of tin clusters up to n
∼ 35, generally follow the prolate
growth pattern of Gen+, but there are
size-specific variations above n = 21 (see Fig. 9). This suggests that tin clusters in this
size regime adopt the same TTP-based geometries as their silicon and
germanium analogs. In the n
∼ 35–65 size range, tin
clusters gradually rearrange towards near-spherical geometries, passing
through several intermediate structural families. This stepwise
‘creep’ towards spherical structures is distinct from the
abrupt rearrangements that occurs for Sin and
Gen cations.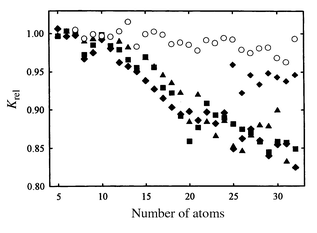 |
| Fig. 9 Relative mobilities of group 4 cluster cations measured at room
temperature. Filled symbols are for silicon (diamonds), germanium
(squares), and tin (triangles). Empty circles are for lead. | |
Mobilities were measured for tin clusters at low temperatures. Two or
three isomers were resolve for some Snn+
sizes in the n = 18–49 range at 78 K. However, the emergence
of these isomers is just a consequence of the improved resolution at low
temperature. Hence the geometries of Snn+
remain unchanged between 300 K and 78 K. In contrast, bulk tin undergoes a
reversible β to α phase transition within this temperature
range. Furthermore, annealing with high injection energies does not affect
the Snn+ mobilities even up to the point of
collisional dissociation. So the prolate TTP-based structures certainly
appear to be the lowest-energy geometries for tin clusters up to n
∼ 35. This is the first time that clusters of a metallic element have
been found to form highly distorted, non-compact geometries; in all other
cases they form near-spherical, close-packed structures.
10 Melting points of tin clusters
The melting transition in finite systems has attracted attention since
the early 1900s. All theoretical treatments (both analytical/macroscopic
and computational/microscopic) show a depression of the melting point in
nanoparticles compared to that of the bulk.39 This has been confirmed in experiments on both
surface-supported and gas-phase clusters.40
The simple explanation for the depressed melting points is that the surface
atoms are under-coordinated and thus can be detached from their positions
and moved around easier than internal atoms. Previously, the melting points
of free (unsupported) metal clusters have been determined indirectly by a
novel method that depends on monitoring the extent of photodissociation as
a function of temperature.40 Another way of
locating the melting transition is directly through a shape change. Since a
liquid cluster is expected to be a spherical droplet, if the solid cluster
has a geometry that is distorted from spherical, it should be possible to
identify the melting transition from the mobility change associated with
the non-spherical to spherical transition. Tin clusters with n
∼ 20–30 have highly prolate geometries and the low melting point
of bulk tin (505 K) makes the relevant temperature range easily accessible.
Note that many small molten metal clusters are expected to be slightly
distorted from perfect spherical symmetry because of electronic shell
effects. However, these distortions are small and, in particular, they are
much less distorted than the prolate tin clusters. Mobilities have been
measured for Snn+ (n < 32) at
temperatures up to ∼ 555 K.41
Surprisingly, the clusters retained their prolate geometries, thus no
melting occurs at temperatures up to 50 K above the bulk melting point.
This is the first observation of solid clusters above the bulk melting
point. However, the origin of the enhanced melting points is not really
clear. One factor may be that the clusters have geometries which are quite
different from the body-centered tetragonal habit of β-tin. On the
other hand, the cohesive energies of the reconstructed clusters are still
expected to be significantly less than the cohesive energy of bulk tin.11 The covalent to metal transition in group 14
clusters
It is apparent from the preceding that the geometries of medium-sized
tin clusters are more closely allied with the semiconducting elements of
group 14 than the close packed near-spherical geometries expected for a
typical metal cluster. In a sense, the nonmetal-to-metal transition that
occurs between germanium and tin in the bulk elements fails to occur in
their clusters. So a natural question to ask is whether this transition
occurs between Snn and Pbn.
Relative mobilities measured for Pbn (n
≤
32) cations are shown in Fig. 9.42 The relative mobilities are close to one for all
Pbn+ studied, which indicates that they
assume compact near-spherical geometries throughout this size range. Such
geometries are expected for metal clusters, and one can conclude that the
transition from ‘covalent’ to ‘metallic’ geometries
in the group 14 clusters occurs between tin and lead, one row lower than in
the bulk elements.12 Conclusions
In this article we have described recent developments in the measurement
and analysis of ion mobilities. The new high resolution implementation of
the technique provides an order of magnitude improvement in the resolving
power and permits the separation of previously unresolved structural
isomers. Structural assignments are based on the comparison of measured and
calculated mobilities, and there has been a corresponding improvement in
the methods used to calculate mobilities: the trajectory method and the
SEDI method (which is based on scattering off of the electron density) are
more sophisticated and more reliable than previous methods.A combination of ion mobility measurements and density functional theory
has been used to elucidate the geometries of medium-sized semiconductor
clusters. The geometries are based on stacked tricapped trigonal prisms.
Mobility measurements alone can only provide information on the general
shape of the cluster. They usually cannot identify the specific geometry
because many different geometries can have the same mobility. However, the
combination of theory with mobility measurements provides a powerful
approach to identifying the lowest-energy geometry. Agreement with the
measured mobility is a good indication that the lowest-energy geometry has
been found and that the search for a lower-energy geometry can be
terminated. For silicon cluster anions and cations with n
≤ 18
there was good agreement between the measured mobilities and those
determined for the optimized geometries, except for
Si15− and Si18+. For
these clusters the second-lowest energy isomer fits the experimental data.
It also possible that the lowest-energy isomer has not yet been found in
these cases. The methods described here are quite general and should be
extendable to larger cluster sizes and to different types of clusters.
In addition to information on the geometries, ion mobilities can be used
to provide information on phase transitions in clusters. So far the method
has only been used to show that tin clusters with around 20–30 atoms
have melting points that are more than 50 K above that of the bulk. The
measurement of melting points for individual clusters as a function of size
are feasible. The method should also be extendable to clusters of other
types of elements, the only limitation is that there should be a detectable
geometry or volume change associated with the melting transition.
13 Acknowledgments
We are indebted to K.-M. Ho, B. Liu, Z.-Y. Lu, and C.-Z. Wang for an
extended collaboration on the optimization of semiconductor clusters and
the calculation of their properties. We are grateful to G. C. Schatz for
help in developing methods to calculate mobilities. This research was
supported by the National Science Foundation and by the Army Research
Office.14 References
- For a recent review of atomic cluster studies, see, W. A. de Heer, Rev. Mod. Phys., 1993, 65, 611 CrossRef CAS.
- For a recent review of gas phase biomolecule studies, see, C. S. Hoagland-Hyzer, A. E. Counterman and D. E. Clemmer, Chem. Rev., 1999, 99, 3037 CrossRef.
- E. A. Mason and
E. W. McDaniel,
Transport Properties of Ions in Gases,
Wiley, New York,
1988. Search PubMed.
- For a recent review of the analytical applications of ion mobility
measurements, see, J. I. Baumbach and G. A. Eiceman, Appl. Spectrosc., 1999, 53, 338A Search PubMed.
- T. M. Sanders and S. R. Forest, J. Appl. Phys., 1989, 66, 3317 CrossRef CAS.
- T. W. Carr, J. Chromatogr. Sci., 1977, 15, 85 CAS.
- G. von Helden, M. T. Hsu, P. R. Kemper and M. T. Bowers, J. Chem. Phys., 1991, 95, 3835 CrossRef CAS.
- See, for example, P. Dugourd, R. R. Hudgins, J. M. Tenenbaum and M. F. Jarrold, Phys. Rev. Lett., 1998, 80, 4197 CrossRef CAS.
- For a review of the fullerene formation mechanisms, see, N. S. Goroff, Acc. Chem. Res., 1996, 29, 77 CrossRef CAS.
- For an overview of the other cluster studies, see, D. E. Clemmer and M. F. Jarrold, J. Mass Spectrom., 1997, 32, 577 CrossRef CAS.
- See, for example, M. F. Jarrold and J. E. Bower, J. Chem. Phys., 1992, 96, 9180 CrossRef CAS.
- Ph. Dugourd, R. R. Hudgins, D. E. Clemmer and M. F. Jarrold, Rev. Sci. Instrum., 1997, 68, 1122 CrossRef CAS.
- C. Wu, W. F. Siems, G. R. Asbury and H. H. Hill, Anal. Chem., 1998, 70, 4929 CrossRef CAS.
- H. E. Revercomb and E. A. Mason, Anal. Chem., 1975, 47, 970 CrossRef CAS.
- M. F. Jarrold and E. C. Honea, J. Phys. Chem., 1991, 95, 9181 CrossRef CAS.
- L. A. Viehland and A. S. Dickinson, Chem. Phys., 1995, 193, 255 CrossRef CAS.
- A. A. Shvartsburg and M. F. Jarrold, Chem. Phys. Lett., 1996, 261, 86 CrossRef CAS.
- M. F. Mesleh, J. M. Hunter, A. A. Shvartsburg, G. C. Schatz and M. F. Jarrold, J. Phys. Chem., 1996, 100, 16082 CrossRef CAS.
- A. A. Shvartsburg, B. Liu, M. F. Jarrold and K.-M. Ho, J. Chem. Phys., 2000, 112, 4517 CrossRef CAS.
- Q. L. Zhang, Y. Liu, R. F. Curl, F. K. Tittel and R. E. Smalley, J. Chem. Phys., 1988, 88, 1670 CrossRef CAS.
- M. F. Jarrold, Science, 1991, 252, 1085 CAS.
- E. C. Honea, A. Ogura, C. A. Murray, K. Raghavachari, W. O. Sprenger, M. F. Jarrold and W. L. Brown, Nature, 1993, 366, 42 CrossRef CAS.
- C. C. Arnold and D. M. Neumark, J. Chem. Phys., 1993, 99, 3353 CrossRef CAS.
- K. Raghavachari and C. M. Rohlfing, J. Chem. Phys., 1988, 89, 2219 CrossRef CAS.
- P. Ballone, W. Andreoni, R. Car and M. Parrinello, Phys. Rev. Lett., 1988, 60, 271 CrossRef CAS.
- I. Vasiliev, S. Öǧüt and J. R. Chelikowsky, Phys. Rev. Lett., 1997, 78, 4805 CrossRef CAS.
- M. V. Ramakrishna and A. Bahel, J. Chem. Phys., 1996, 104, 9833 CrossRef CAS.
- U. Röthlisberger, W. Andreoni and P. Giannozzi, J. Chem. Phys., 1992, 96, 1248 CrossRef.
- R. R. Hudgins, M. Imai, M. F. Jarrold and Ph. Dugourd, J. Chem. Phys., 1999, 111, 7865 CrossRef CAS.
- A. A. Shvartsburg, M. F. Jarrold, B. Liu, Z.-Y. Lu, C.-Z. Wang and K.-M. Ho, Phys. Rev. Lett., 1998, 81, 4616 CrossRef CAS.
- K. Fuke, K. Tsukamoto, F. Misaizu and M. Sanekata, J. Chem. Phys., 1993, 99, 7807 CrossRef CAS.
- K.-M. Ho, A. A. Shvartsburg, B. Pan, Z.-Y. Lu, C.-Z. Wang, J. G. Wacker, J. L. Fye and M. F. Jarrold, Nature, 1998, 392, 582 CrossRef CAS.
- J. C. Grossman and L. Mitas, Phys. Rev. Lett., 1995, 74, 1323 CrossRef CAS.
- E. Kaxiras and K. A. Jackson, Phys. Rev. Lett., 1993, 71, 727 CrossRef CAS.
- B. Liu, Z.-Y. Lu, B. Pan, C.-Z. Wang, K.-M. Ho, A. A. Shvartsburg and M. F. Jarrold, J. Chem. Phys., 1998, 109, 9401 CrossRef CAS.
- J. M. Hunter, J. L. Fye, M. F. Jarrold and J. E. Bower, Phys. Rev. Lett., 1994, 73, 2063 CrossRef CAS.
- A. A. Shvartsburg, B. Liu, Z.-Y. Lu, C.-Z. Wang, M. F. Jarrold and K.-M. Ho, Phys. Rev. Lett., 1999, 83, 2167 CrossRef CAS.
- A. A. Shvartsburg and M. F. Jarrold, Phys. Rev. A, 1999, 60, 1235 CrossRef CAS.
- For a review of melting and freezing in finite systems,
see, R. S. Berry, Microscale Thermophysical Engineering, 1997, 1, 1 Search PubMed.
- See, for example, M. Schmidt, R. Kusche, B. von Issendorff and H. Haberland, Nature, 1998, 393, 238 CrossRef CAS.
- A.A. Shvartsburg and M.F. Jarrold, Phys. Rev. Lett., 2000, 85, 2530 CrossRef CAS.
- A.A. Shvartsburg and M.F. Jarrold, Chem. Phys. Lett., 2000, 317, 615 CrossRef CAS.
Footnotes |
† Present address: Department of Chemistry,
York University, 4700 Keele Street, Toronto, Ontario, M3J 1P3, Canada. |
‡ Present address: Institute for Physical
Chemistry, University of Basel, Klingelbergstrasse 80, CH-4056 Basel,
Switzerland. |
|
This journal is © The Royal Society of Chemistry 2001 |
Click here to see how this site uses Cookies. View our privacy policy here.