DOI:
10.1039/B006221I
(Paper)
Analyst, 2001,
126, 71-78
High sensitivity microgravimetric biosensor for
qualitative and quantitative diagnostic detection of polychlorinated
dibenzo-p-dioxins
Received 1st August 2000, Accepted 24th October 2000
First published on 30th November 2000
Abstract
A piezoelectric immunosensor system was developed for the
rapid detection of polychlorinated dibenzo-p-dioxins (PCDDs). The
system uses a competitive inhibition enzyme immunoassay (EIA) based on a
mouse monoclonal antibody that is specific for
2,3,7,8-tetrachlorodibenzo-p-dioxin (2,3,7,8-TCDD) and a conjugate
of a dioxin-like competitor coupled to the enzyme horseradish peroxidase
(HRP). The anti-dioxin antibody was deposited on a 10 MHz AT-cut quartz
crystal resonator modified with a self-assembly monolayer of
dithiobis-N-succinimidyl propionate. PCDDs at different
concentrations in the range 0.001–10 ng mL−1 were
mixed with a constant amount of HRP-conjugated competitor. The frequency
responses due to the adsorption of the mixed samples on the biosensor
surface were measured. The results show that 2,3,7,8-TCDD can be
quantitatively detected with the developed immunosensor in the
concentration range 0.01–1.3 ng mL−1.
Cross-reactivities of the biosensor to various PCDD congeners were also
investigated. The sensitivity and selectivity of the quartz crystal
microbalance (QCM) biosensor is comparable to EIA and ELISA methods in the
detection of polychlorinated dibenzo-p-dioxins. The developed QCM
immunosensing system offers significant improvements in speed, sample
throughout and cost for the qualitative and quantitative detection of PCDDs
compared with GC-MS.
Introduction
Polychlorinated dibenzodioxins (PCDDs) and polychlorinated dibenzofurans
(PCDFs) are persistent, toxic pollutants which pose a threat both to human
health and to the biosphere generally.1,2 PCDDs and PCDFs have been shown to be widespread
environmental pollutants, occurring in most human and animal adipose
samples, milk, sediment and numerous other matrices.3–8
2,3,7,8-Tetrachlorodibenzo-p-dioxin (TCDD), commonly reported by
news media as dioxin, is one member (congener) of the polychlorinated
dibenzo-p-dioxin family, of which there are 75 possible congeners
whose structures vary according to the number and location of the chlorine
atoms. TCDD is known as the most toxic congener. With a view to
environmental protection, it has become a matter of high priority to
address the issue of detection of these compounds. An important,
non-trivial first step is to identify sites of pollution, and on-field
monitoring, which requires a simple, economical and rapid test for the
detection of the presence of these compounds in samples taken from
industrial processing, soils, human and animal tissues and foodstuffs.
Because the toxicity varies among the congeners, the analyses for PCDDs
require the identification and quantification of each isomer and
congener.The present methods for the congener specific analysis of PCDDs or PCDFs
typically use high-resolution gas chromatography with ion capture or mass
spectrometric detection systems9,10
Although these methods are accurate, there are also very expensive and time
consuming because of their reliance on sophisticated analytical
instrumentation and skilled operators. Depending on the amount of sample
preparation needed, the analysis can take several days to complete. As a
result, the screening of large numbers of samples has been limited and
supplemental methods are required. Although the regulation of PCDDs and
PCDFs differs from country to country, there is common analytical interest
in determining the presence and amounts of PCDDs and PCDFs and of their
toxic congeners in the environment, and in materials that are potential
sources of PCDDs or PCDFs in the environment. Regardless of the laws and
rules, the analytical needs are similar: reliable, practical, rapid,
cost-effective, field-portable and high-sensitivity methods are needed that
can determine PCDDs or PCDFs and their toxic congeners in a variety of
matrices.
Immunoassay based analytical methods have demonstrated value for
specific, high throughput screening and quantitative analyses of many
environmental analytes.11 Moreover,
immunoassay can be accomplished with minimal sample preparation and
instrumentation. Existing immunoassays for PCDDs or PCDFs are
radioimmunoassays (RIAs) and enzyme immunoassays (EIAs) that give varying
specificity for the PCDDs and PCDFs.12,13 However, the reported RIAs were time consuming and
utilized polyclonal antibodies (PAbs).12
Stanker et al. generated a monoclonal antibody (MAb) to dioxin and
developed MAb-based enzyme-linked immunosorbent assay (ELISA)
formats.14–17 The
selectivity of the ELISA was very similar to that of the RIA. The optimized
assay detected 2000 pg per well of 2,3,7,8-TCDD as the IC50 (the
analyte concentration giving 50% inhibition).17 Langley et al.18 reported the development of PAb-based ELISAs
that detected 1 ng per well of 2,3,7,8-TCDD as the IC50.
Recently, Harrison and Carlson developed a tube test and a microplate test
system and reported detection limits of 100 and 25 pg per well of
2,3,7,8-TCDD, respectively.19 Sugawara
et al. reported an ELISA method for the detection of PCDDs based
on polyclonal antibodies.20 Although these
results led to increased sensitivity, further improvements are needed to
approach the detection limits of GC-MS (⩽1 pg of 2,3,7,8-TCDD in a 1 g
sample).
Biosensors are considered to be promising tools for monitoring pollution
in the environment.21–23
Biosensors are rapid, cost-effective, field-portable and high-sensitivity
instruments. Many biosensing devices including optical, electrochemical and
wave acoustic sensors are being developed for the detection of triazine
herbicides, represented by atrazine.24–29 The direct and indirect immunochemical
system have been investigated to improve the biosensor properties. The
indirect immunochemical system uses a variety of labeling approaches to
quantity the extent of competitive immunoreaction.
Piezoelectric transducers offer the advantages of a solid-state
construction, chemical inertness, durability and ultimately the possibility
of low cost mass production. The piezoelectric based quartz crystal
microbalance (QCM) is a high-sensitivity sensor for the mass deposited on
its surface. In recent years, QCM methods have found wide application in
immunoassay.30–32 QCM
measures the immunochemical interaction without any chemical labels by
monitoring the oscillation frequency change, which is proportional to the
analyte concentration. The crystal surface is coated with an
immunoadsorbent that selectively interacts with the analyte of interest and
subsequent binding increases the mass of the coated crystal with a
resulting shift of its fundamental frequency of oscillation. A plethora of
methods for antibody immobilization on the crystal surface have been
investigated, including physical adsorption of antibodies,33–35 cross-linking,28,36,37 covalent attachment to
self-assembled monolayers,38–41 entrapment,28,42,43 and, more recently, the use of
antibody–thiol complexes.44 This last
technique has also had much success in the analysis of biotin,45 herbicides in drinking water,28 microbes, enzymes,37,43 viruses46,47
and human cells.48
In this paper, we report the development of a highly sensitive QCM
biosensors for the detection of PCDDs based on a competitive immunoreaction
by measuring the frequency change of a dioxin-like competitor coupled to
the enzyme horseradish peroxidase that is introduced to compete for the
available binding sites on the immobilized antibody. The principle
piezoelectric immunosensor is illustrated in Scheme
1. The determination of polychlorinated
dibenzo-p-dioxin (PCDD) at sub-nanomolar concentrations was
attempted using such immunosensors. The optimized assay was then used to
assess the cross-reactivity by congeners of halogenated dioxins and related
structures.
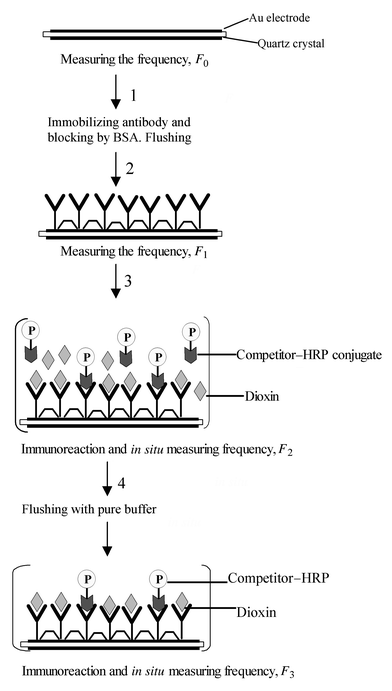 |
| Scheme 1 Schematic illustration of the immunosensor preparation and
measurement. | |
Experimental
Chemicals
The standards 2,3,7,8-tetrachlorodibenzo-p-dioxin
(2,3,7,8-TCDD), 1,2,3,4-tetrachlorodibenzo-p-dioxin
(1,2,3,4-TCDD), 1-chlorodibenzo-p-dioxin (1-CDD),
2,7-dichlorodibenzo-p-dioxin (diCDD),
octachlorodibenzo-p-dioxin and
2,3,7-trichlorodibenzo-p-dioxin (2,3,7-triCDD) were purchased from
Chem Service (West Chester, PA, USA) and
1,2,3,7,8-pentachlorodibenzo-p-dioxin (PentaCDD) and
1,2,4-trichlorodibenzo-p-dioxin (1,2,4-triCDD) from Cambridge
Isotope Laboratories (Andover, MA, USA). Competitor compound (Scheme 2) was synthesized by Friedel–Crafts
acylation of the appropriate chlorobenzene followed by isolation of the
desired isomer. Horseradish peroxidase (HRP),37,49 bovine serum albumin and Tween 20 were purchased from
Sigma Chemical (St. Louis, MO, USA). Dithiobis-N-succinimidyl
propionate (DTSP), N-hydroxysuccinimide (NHS) and
N-ethyl-N′-[3-(dimethyl)aminopropyl]carbodiimide
hydrochloride (EDC) were purchased from Fluka (Buchs, Switzerland) and
stored at 4 °C. Other chemicals and reagents were purchased from Fisher
Scientific (Pittsburgh, PA, USA) or Aldrich Chemical (Milwaukee, WI, USA).
Mouse monoclonal anti-dioxin antibody DD-3 was obtained from the American
Type Culture Collection (ATCC) (Rockville, MD, USA).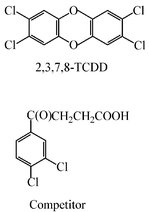 |
| Scheme 2 Structures of polychlorinated dibenzo-p-dioxins and competitor
used in this study. | |
Synthesis of HRP-conjugated competitor
Competitor was conjugated to HRP using a carbodiimide-mediated carboxyl
activation procedure. A typical procedure for synthesis of the conjugated
competitor is as follows. The competitor, calculated to be in a 100-fold
molar excess over HRP, was dissolved in 400 μL of dimethylformamide and
activated to form the N-hydroxysuccinimide (NHS) ester. The
activation was carried out using a 1.4-fold molar excess (calculated over
the competitor) of EDC and a twofold molar excess (calculated over the
competitor) of NHS added dry to the competitor solution. The HRP protein
was dissolved in borate buffer (0.1 M, pH 9.4) to a final concentration of
10 mg mL−1. The HRP solution was stirred overnight at
0–5 °C to ensure that all of the protein was dissolved.
Dimethylformamide was added to a concentration of 5% v/v. The activated
competitor solution was added to the HRP solution, 10 μL at a time,
every 30–60 min, using a 10 μL pipetter. The conjugation mixtures
were stirred overnight at room temperature after the competitor solution
had been added. The reaction solutions were transferred to wetted cellulose
dialysis tubing (Mr cut-off 1200–14000) and
dialyzed vs. two changes of 0.5–1.0 L volumes of 10% v/v
propan-2-ol in phosphate-buffered saline (PBS) (pH 7.4) over 2 d. The
reaction solutions were then dialyzed vs. two changes of
0.5–1.0 L of PBS over 2 d to remove any trace of propan-2-ol. After
dialysis, the conjugate solution was collected from the dialysis tubing.
The conjugate stock solutions typically had a concentration of 5–10
mg mL−1 HRP, and they were stored at 4 °C.Instrumentation
The quartz crystals employed in this study were commercially available
10 MHz, AT-cut type (diameter 13.67 mm) with Au electrodes (5.1 mm
diameter) on both sides, purchased from International Crystal Manufacturing
(Oklahoma City, OK, USA). The electrodes from the crystal were connected to
a TTL oscillating circuit based on IC 74LS04, similar to that described in
the literature.50 The output frequency was
measured using a universal counter (Thurlby-Thandar, Cambridge, UK, Model
TF830) attached to a personal computer. PZTools (Universal Sensors, USA)
was used for measurements, data storage and evaluation). The following
equation has been established for an AT-cut shear mode QCM by
Sauerbrey:51 | Δf = −2
f02(ρqμ
q)−1/2Δm/A | (1
) |
where Δf is the measured frequency shift due to the added
mass in hertz, f0 the fundamental oscillation frequency
of the dry crystal in hertz, Δm/A the surface mass loading
in grams per square centimeter, ρq the density of
quartz (2.65 g cm−3) and μq the
shear modulus (2.95 x1011 dyn cm−2). For the 10
MHz quartz crystals used in this work, eqn.
(1) predicts that a frequency change of 1 Hz corresponds to a mass
increase of 0.902 ± 0.01 ng on the electrode.For in situ detection of frequency change, the quartz crystal
was fixed vertically between two blocks of Plexiglas of a detection cell in
which only one side of the quartz crystal was allowed to contact the
aqueous sample solution. The detection cell was set up in a water-bath at
constant temperature (32 °C). The frequency response was stable within
±1.0 Hz when contacted with buffer solution.
Electrode conditioning
Gold-disk electrodes were polished with diamond paste down to 1 μm,
followed by sonication in a water-bath. The cyclic voltammogram
characteristic of a clean gold electrode was recorded at 100 mV
s−1 and used to calculate the microscopic area. The
electrode was subsequently rinsed with water and acetone and used
immediately in the preparation and characterization of the
dithiobis-N-succinimidyl propionate (DTSP) monolayer.Immobilization of antibody on quartz
The two sides of the Au electrodes of the QCM were washed with freshly
prepared ‘piranha’ solution
(3H2SO4·1H2O2)
(Caution: this mixture reacts violently with organic
materials, and it should not be stored in closed containers) in order
to remove organic adsorbate impurities from the gold surfaces. The QCMs
were rinsed water purified with a Milli-Q system several times and dried
under a nitrogen atmosphere. The cleaned crystals were incubated with 4 mM
DTSP in absolute DMSO for 12 h, thoroughly rinsed with absolute ethanol,
and allowed to dry. The coupling of DD-3 antibody on the gold surface was
then performed in 0.1 mg mL−1 DD-3 in PBS (20 mM, pH 7.4)
containing 0.05% Tween 20 (PBST) for another 4 h. After rinsing with
distilled water, 3% BSA in PBST was used to block any unreacted spots of
DTSP on the gold surface. The crystal was then rinsed with pure buffer.
Bare QCM and QCM immobilized without DD-3 antibody but only BSA in the same
manner were used for control experiments.Quartz crystal microbalance (QCM) measurements
A PCDDs stock standard solution was prepared in DMSO. The stock standard
solutions were serially diluted to concentrations in the range
0.001–10 ng mL−1. A 10 μl volume of the sample
solution was mixed with 20 μl of the HRP-conjugated competitor in PBST
buffer. QCM measurements were performed with one side of the quartz crystal
in contact with 0.5 mL of PBST buffer solution. After stabilization of the
fundamental resonance frequency of the quartz crystal, an aliquot of
mixture was injected into the solution. The frequency changes in solution
due to competitive adsorption of competitor on the surface of the crystal
were monitored as a function of time.Buffer solution
PBST was used as the background solution for the sensor
measurements.Determination of cross-reactivities
The cross-reactivities (CR) were calculated relative to the
concentration producing 50% inhibition (IC50) by PCDDs. The data
were obtained from calibration curves for the related compounds and
calculated according to the equation | %CR =
(IC50 of TCDD)/(IC50 of the cross-reacting compound)
× 100 | (2) |
Safety considerations
Extreme caution was exercized to avoid exposure to PCDDs during the
assays because the toxicity of the compounds utilized in this study is
unknown. When dioxins and related compounds are handled, two pairs of
protective gloves should be worn with some water between the two layers to
avoid penetration of highly lipophilic compounds. Activated carbon can be
used to eliminate PCDD-like substances from waste solutions. UV radiation
has been reported to degrade PCDDs and some related compounds and may
therefore be useful for cleanup.Results and discussion
Sensing interface preparation and characterization
The application of self-assembled monolayers (SAMs) for protein
immobilization on gold surfaces has grown enormously in recent years
because they offer a method of orientated, covalent attachment of
antibodies to the gold surface that form a densely packed monolayer which
should lead to tightly bound layers of protein. The most commonly used SAMs
are thiols and sulfides bearing terminal carboxylic acid groups. For
immobilization of protein, the formed SAM of thiol was activated with EDC
and then NHS. The two-step immobilization method is time consuming and
difficult to handle. DTSP is a water insoluble, homobifunctional NHS ester,
known as Lomant’s reagent.52 DTSP
also can form a self-assembly monolayer on gold surfaces,53 through the disulfide group, so that the
terminal succinimidyl groups allow further covalent immobilization of
amino-containing biomolecules through acylation of free primary or
secondary aliphatic amino groups.54 In this
work, we use DTSP for the immobilization of the antibody. The formation of
the DTSP self-assembly monolayer was characterized. Fig. 1 shows the cyclic voltammetric
characterization of the DTSP modified electrode by soaking of a gold
electrode/crystal in a 4 mM solution of DTSP in DMSO. As can be seen in
Fig. 1(a), there was a significant decrease
in the capacitative current, as would be expected for an electrode covered
with a low dielectric layer [Fig. 1(a),
solid line]. However, no Faradaic processes were observed over the
potential range from 0 to −0.4 V for either the bare or the DTSP
modified gold electrode. This result indicates that an adsorbed layer in
formed upon immersion of gold/crystal in DTSP solution, and this layer is
stable to rinsing with buffer solution. A typical voltammogram shows a
well-defined chemically irreversible wave with a peak potential value of
–0.74 V [Fig. 1(b), solid line]. On
subsequent scans, the peak was essentially absent [Fig. 1(b), dashed line]. This result is consistent
with the reductive desorption of a 3-mercaptopropane monolayer from a gold
electrode surface as reported by Imabayashi et al.55 The surface coverage calculated by integration
of the charge under the voltammetric wave was established to be 1.4 ×
10−10 mol cm−2, assuming that the process
involves the transfer of two electrons per mole.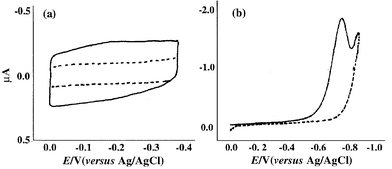 |
| Fig. 1 (a) Cyclic voltammogram at 50 mV s−1 of a bare gold
electrode and a gold electrode modified with a DTSP monolayer over the
potential range from 0.0 to −0.4 V. (b) Linear sweep voltammograms of
a gold electrode modified with a DTSP monolayer in 0.1 M phosphate buffer
(pH 7.0) over the potential range from 0.0 to −0.90 V. | |
The immobilization of DD-3 antibody on DTSP modified gold surfaces takes
place by nucleophilic attack of primary amino groups of the enzyme on the
terminal N-succinimidyl esters in the monolayer. To study the
immobilization of the antibody on a DTSP monolayer, a DTSP modified QCM Au
crystal was installed in the detection cell with one side of the electrode
contacting a thermostated solution (0.5 mL of 20 mM phosphate buffer, pH
7.4) and the frequency was monitored as a function of time. After the
temperature and the frequency had stabilized, 5 μL of the antibody stock
standard solution (1 μg μL−1) were added to the
solution, so that the final antibody concentration was 0.01μg
μL−1. After the frequency decrease had reached a steady
state, the detection cell was flushed with pure buffer and 0.5 mL of pure
buffer was added. A 10 μL volume of 0.05 mg mL−1 BSA
solution in PBST was injected into the detection cell to block the
non-specific binding sites. Fig. 2 shows the
resulting frequency changes as a function of time. As can be seen, on
addition of the antibody, the frequency decreased rapidly during the first
5 min and subsequently decreased more slowly until a steady state was
reached within 20 min. The frequency change for anti-dioxin antibody
adsorption is 128 Hz. Only a small frequency increase (7 Hz) was observed
after flushing with buffer solution, indicating that the antibody was
covalently bonded on the DTSP monolayer and the co-adsorption of antibody
was negligible.
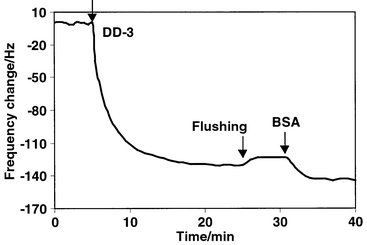 |
| Fig. 2 Time dependence of the frequency changes of a DTSP modified quartz
crystal resonator in 5.0 mM phosphate buffer solution at 25 °C, on
addition of anti-dioxin antibody. | |
However, the in-solution frequency change
(ΔFsol) of antibody immobilization on the DTSP
modified Au/crystal cannot be directly transposed to mass change because in
the liquid phase the QCM does not necessarily behave as predicted by the
Sauerbrey equation51 owing to viscoelastic
effects arising from the solvent and the adsorbed layers. In addition,
water entrapped within the layers also contributed to the observed
in-solution frequency changes. In order to study the immobilized amount of
antibody, the antibody adsorption after rinsing with water and drying is
recorded. The in-air frequency change (ΔFair) for
the antibody immobilization after drying is 58 ± 5 Hz (average
± standard deviation for triplicate experiments). The factor of
∼2 difference between the ΔFsol and
ΔFair values can be ascribed to water entrapped
within the protein layers. Converting the ΔFair
value to a mass using 0.902 ng Hz−1 for the QCM
system56 yields 50 ± 5 ng for
anti-dioxin antibody immobilized on the QCM. The immobilization procedure
shows good reproducibility, the average frequency change due to the
adsorption of antibody was 130 Hz and the relative standard deviation was
10%, as indicated in Table 1. After
flushing with pure buffer again, the QCM sensor is ready for dioxin
analysis.
Table 1 Frequency changes for the immobilization of anti-dioxin antibody and
blocking with BSA
No. | Frequency change for antibody/Hz | Frequency change for BSA blocking/Hz |
---|
1 | 132 | 15 |
2 | 119 | 27 |
3 | 120 | 20 |
4 | 145 | 31 |
5 | 122 | 19 |
6 | 140 | 22 |
7 | 113 | 30 |
8 | 142 | 32 |
9 | 125 | 21 |
10 | 146 | 18 |
Average ±
s | 130 ± 10 | 20 ± 5 |
Calibration graph for PCDD
The Sauerbrey theory is based on the idealized assumption that mass
coating of the QCM shows ideal acoustic coupling to the crystal surface and
the crystal is an infinite plane. Recent advances in solution sensing
indicated that the QCM frequency responses are influenced by many factors,
such as mass, effective viscosity, conductivity, dielectric constant,
electrode morphology, density and temperature of the liquid and ionic
status of a crystal–electrode interface with respect to
water–buffer. This indicates the need to characterize the QCM
biosensing system with the respective sensing environment. In order to
minimize the effect of temperature change on the sensing system, the
detection cell was set up in a water-bath at a constant temperature of 32
°C. To facilitate minimization of possible pH changes and viscosity
changes due to the addition of sample to the detection cell, 0.02 M PBST
buffer was used in all experimental detection procedures. Fig. 3 shows the response profiles of DD-3 antibody
coated crystals on addition of pure enzyme HRP and competitor–HRP
conjugate to the buffer solution. The frequency response on the addition of
20 μL of enzyme HRP sample (20 μg) to the detection buffer was very
small (3–5 Hz) (curve a). This obviously implies that the frequency
changes caused by the pH changes and viscosity changes due to the sample
addition to the detection cell are negligible. (The volume of sample
injected into the detection cell was less than 20 μL in all the
experiments). The frequency change caused by the addition of HRP-conjugated
competitor, which reached a plateau of 130 Hz, can be attributed to the
affinity interaction of the competitor with the specific antibody
immobilized on the crystal surface (Fig. 3,
curve b).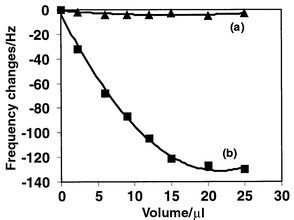 |
| Fig. 3 Typical response profiles of the antibody immobilized sensor in the
detection cell with 0.5 mL of PBST buffer on addition of 10 mg
mL−1 of pure HRP solution (a) and 10 mg
mL−1 HRP conjugated competitor solution (b). | |
The molecular weight of PCDDs is around 300–600, hence the
expected frequency change due to the immunological adsorption of PCDDs on
the immobilized antibody layer should be much smaller than that obtained
for the antibody. In order to detect the dioxin at sub-nanomolar
concentrations, a competitive immunoassay was carried out to enhance the
frequency change due to the adsorption of larger molecules than the PCDDs.
Fig. 4 shows the typical response of a DD-3
immobilized crystal to TCDD–competitor mixtures and the results of
control experiments. The immunoreaction can be completed, as shown by a
steady-state frequency response that was reached after 10 min. However, the
frequency response for the direct detection of dioxin is very small and
cannot permit reasonable detection (Fig. 4,
curve a). In the competitive immunoreaction system, the frequency change
measured is caused by the competitive immunological adsorption of both TCDD
and competitor on the sensing interface. The amount of conjugated
competitor bound by anti-dioxin antibody is inversely related to the amount
of TCDD originally present in the sample. Hence, the observed frequency
change is also inversely related to the TCDD concentration. As the
HRP-conjugated competitor is a large biomolecule, the frequency response is
enhanced (Fig. 4, curves b–d). In
order to establish the background response for the biosensor, a series of
control experiments were performed by interaction of uncoated crystal and
DTSP–BSA coated crystal with the analyte and conjugated competitor,
respectively. Fig. 4 (curves e–h)
indicate that the non-specific adsorption of TCCD at 10 ng
mL−1 to the control crystals is undetectable (<2
± 2 Hz) and the non-specific adsorption of conjugated competitor on
the control crystals is <14 Hz. The level of non-specific adsorption was
found to be approximately 10% of the biosensor response. This result
suggests that the non-specific adsorption of analytes and conjugated
competitor has little effect on the frequency response of the developed
immunosensor. Hence the frequency changes in Fig.
4 (curves b–d) testify to be the expected immunoreaction of
the analyte and competitor with the immobilized anti-dioxin antibody.
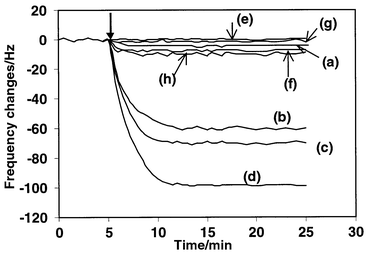 |
| Fig. 4 Typical response profiles of the anti-dioxin antibody modified sensor in
the detection cell on reaction with (a) 5 μg mL−1 TCDD,
(b) 2 μg mL−1 of competitor + 1 ng
mL−1 TCDD, (c) 2 μg mL−1 competitor +
0.2 ng mL−1 TCDD and (d) 2 μg mL−1
competitor +0.02 ng mL−1 TCDD. Response of the control
crystals: bare Au/crystal in reaction with 5 μg mL−1
TCDD (e) and 2 μg mL−1 of competitor (f);
DTSP–BSA modified Au/crystal in reaction with 5 μg
mL−1 of TCDD (g) and 2 μg mL−1 of
competitor (h). All the concentrations are the final concentration of
analyte in the detection cell. | |
Fig. 5 shows a typical calibration curve
for 2,3,7,8-TCDD generated by employing the anti-dioxin antibody modified
crystal and HRP conjugated competitor. Each point represents the average
value of the measurements of triplicate antibody modified crystals at one
concentration. The calibration curve exhibited a typical sigmodial response
and a long scale with a response range from 0.001 to 10 ng
mL−1. The IC50 of 2,3,7,8-TCDD that produces
50% inhibition is calculated as 0.083 ng mL−1. The limit
of detection (LOD) was calculated as 0.008 ng for 2,3,7,8-TCDD with the
biosensing system. The definition of LOD used here is the concentration of
the frequency shift equal to the frequency shift at zero concentration
minus three times the standard deviation of the frequency shift at zero
concentration. The quantitative working range of the fabricated biosensor
was established between the concentrations producing 15 and 85% frequency
shifts, i.e., 0.01–1.3 ng mL−1. The
detection limit in this work was comparable to the EIA detection system
reported by Harrison and Carlson.19
Compared with EIA techniques, the QCM biosensor is rapid, simple to use and
more suitable for performing on-site field analysis.
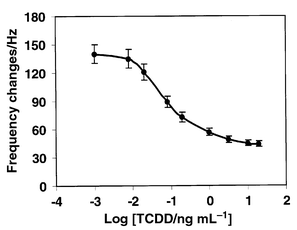 |
| Fig. 5 Calibration graph for TCDD. The data represent average values of three
signal measurements. The standard deviation is indicated by error bars. | |
The precision and accuracy for three individual samples at 0.0125, 0.082
and 1 ng of TCDD (mixed with competitor) were tested with 12 crystals
modified with antibody (Table 2). The
average relative standard deviation (RSD) for TCDD samples of 0.0125, 0.082
and 1 ng were 21, 11 and 8%, respectively. The precision for the spiked
samples was improved as the concentration increased. The average recoveries
were 78, 106 and 89% for TCDD samples of 0.0125, 0.082 and 1 ng,
respectively. As expected, good recoveries were obtained (near 100%) for
samples near the IC50. The frequency changes of the biosensor to
0.0125 and 1 ng samples correspond to 85 and 15% of the frequency shift of
pure conjugated competitor, respectively. These inhibition levels are at
the edge of the portion of the calibration curve used for quantification,
and therefore these measurements were less accurate.
Table 2 Spike recoveries of individual TCDD samplesa
Spike concentration/ng | Average recovery/ng (n = 4)b | Average RSD (%) (n = 4)b | Average recovery (%) (n = 4)b |
---|
The TCDD samples were mixed with conjugated competitor for
measurements. n The number of biosensors tested. |
---|
0.0125 | 0.0098 | 21 | 78 |
0.082 | 0.086 | 11 | 106 |
1 | 0.89 | 8 | 89 |
Cross-reactivity with various PCDDs
The cross-reactivity of the QCM biosensor was evaluated by using various
PCDD congeners. The results of the cross-reactivity study are presented in
Table 3. For determination of
cross-reactivities, the IC50 of 2,3,7,8-TCDD was assigned a
value of 100%, and the cross-reactivities for the other PCDD compounds are
reported according to their IC50 relative to this value. The
data indicated that PCDD compounds having structures with a
1,2,3,4-substitution pattern showed very low cross-reactivity. However,
compounds with three, four or five chlorine atoms in a substitution pattern
similar to that of 2,3,7,8-TCDD exhibited relatively high
cross-reactivities, such as 1,2,3,7,8-PentCDD (106%) and 2,3,7-TriCDD
(34%). Reduction from a congener with three chlorine atoms (2,3,7-TriCDD)
to one with two chlorine atoms (2,7-DiCDD) results in a dramatic decrease
in cross-reactivity (from 34 to 0.9%).
Table 3 Cross-reactivities for the QCM biosensor for the PCDDs
Compound | Cross-reactivity (%) |
---|
2,3,7,8-Tetrachlorodibenzo-p-dioxin | 100 |
1,2,3,7,8-Pentachlorodibenzo-p-dioxin | 106 |
1,2,3,4-Tetrachlorodibenzo-p-dioxin | 0.5 |
1,2,4-Trichlorodibenzo-p-dioxin | 5 |
2,3,7-Trichlorodibenzo-p-dioxin | 34 |
2,7-Dichlorodibenzo-p-dioxin | 0.9 |
1-Chlorodibenzo-p-dioxin | 1 |
2-Chlorodibenzo-p-dioxin | 8 |
Octachlorodibenzo-p-dioxin | 0.03 |
Dibenzo-p-dioxin | 0.02 |
Dibenzofuran | 0.01 |
2,8-Dichlorodibenzofuran | 6 |
Dibenzofuran | 0.01 |
Regeneration of sensing interface
Regeneration of the modified sensor interface by dissociating the bound
analyte from the antibody-coated sensor indicates the reusability of this
biosensor. In this study, both 20 mM glycine–HCl buffer (pH 2.5) and
6 M urea were used to regenerate the QCM biosensor. Typical frequency
responses with time for introductions of 1 ng mL−1 of
2,3,7,8-TCDD–competitor sample, glycine–HCl buffer and fresh
PBST buffer to the anti-dioxin antibody modified crystal are shown in
Fig. 6. The injection of the
TCDD–competitor sample causes a frequency decrease of approximately
60 Hz, which is presumably caused by the immunocomplex formation and the
effect of the viscosity changes of the buffer. When glycine–HCl
buffer solution was introduced, it decreased the frequency further. The
frequency decrease rather than increase (due to the dissociation of analyte
and competitor from the immunocomplex) on introduction of glycine–HCl
buffer solution can be explained by the effect of bulk density and
viscosity changes. Effective removal of the bound analyte by
glycine–HCl can be observed after PBST washing. After the frequency
response had become stable, the regenerated crystal was used to perform
another assay for the TCDD–competitor sample. The results indicate
that the sensing interface still retained more than 86% activity after four
regeneration cycles performed with glycine–HCl buffer (pH 2.5). The
recovered activity using 6 M urea was 70% after four regeneration cycles,
as shown in Table 4. Obviously, the
glycine–HCl buffer (pH 2.5) would be more suitable for the
regeneration treatment.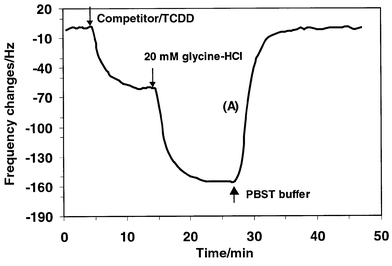 |
| Fig. 6 Frequency response of anti-dioxin antibody modified quartz crystal
resonator to the presence of 0.02 M PBST buffer containing competitor + 1
ng mL−1 TCDD mixture, glycine–HCl buffer solution
(pH 2.5) and 0.02 M PBST fresh buffer. | |
Table 4 Recovered activity for regeneration
Regeneration No | Frequency changea
±
s/Hz | Recovered activity (%) | Frequency changeb
±
s/Hz | Recovered activity (%) |
---|
Regeneration with glycine–HCl buffer (pH 2.5). Regeneration with 6 M urea. The results are the average values of three
measurements. |
---|
1 | 98 ± 5 | 100 | 98 ± 5 | 100 |
2 | 93 ± 5 | 94 | 90 ± 5 | 91 |
3 | 90 ± 4 | 91 | 86 ± 4 | 87 |
4 | 88 ± 5 | 89 | 78 ± 5 | 80 |
5 | 85 ± 4 | 86 | 69 ± 4 | 70 |
Conclusion
We constructed a QCM immunosensor based on a SAM of DTSP on a
gold-plated quartz crystal. An immuno-competitive system was developed to
detect quantitatively the PCDDs. The quantitative working range of the
fabricated biosensor was between 0.01 and 1.3 ng mL−1
TCDD. The detection limit in this work was comparable to that for the EIA
detection system reported by Harrison and Carlson.19 Compared with the EIA techniques, the QCM
biosensor is rapid, simple to use and more suitable for performing on-site
field analysis. The results of this study suggest that the micro-biosensor
array can be used for large-scale sample screening in environmental
monitoring.References
- S. Safe and
O. Hutzinger, in
Polychlorinated Dibenzo-p-dioxins and -furans (PCDDs/PCDFs): Sources
and Environmental Imoact, Epidemiology, Mechanisms of Action, Health
Risks, ed. S. Safe, O. Hutzinger and T. A. Hill, Environmental
Toxin Series 3, Springer, New York,
1990, pp. 1–22. Search PubMed.
- T. Sakurai, J.-G. Kim, N. Suzuki and J. Nakanishi, Chemosphere, 1996, 33, 2007 CrossRef CAS.
- A. Poland and J. C. Knutson, Annu. Rev. Pharmacol. Toxicol., 982, 22, 517 Search PubMed.
- R. D. Kimbrough, H. Falk, P. Stehr and G. Fries, J. Toxicol. Environ. Health, 1984, 14, 47 CrossRef CAS.
- H. Muto and Y. Takizawa, Bull. Environ. Contam. Toxicol., 1992, 49, 701 CrossRef CAS.
- J. P. Giesy, J. P. Ludwig and D. E. Tillitt, Environ. Sci. Toxicol., 1994, 28, 128A Search PubMed.
- C. Rappe, Environ. Sci. Toxicol., 1984, 18, 78A Search PubMed.
- R. J. Kociba and B. Schwetz, Drug Metab. Rev., 1982, 13, 387 CAS.
- USEPA, Method 1613: Tetra Through Octa-Chlorinated Dioxins and
Furans by Isotope Dilution HRGC/HPMS, Revision B, USEPA Office of
Water Regulations and Standards, Washington, DC, 1994..
- R. E. Clement, Anal. Chem., 1991, 63, 1130A CAS.
- J. P. Sherry, CRC Crit. Rev. Anal. Chem., 1992, 64, 217 Search PubMed.
- P. W. Albro, M. I. Luster, K. Chae, S. K. Chaudhary, G. Clark, L. D. Lawson, J. T. Corbett and J. D. Mckinney, Toxicol. Appl. Pharmacol., 1979, 50, 137 CrossRef CAS.
- S. J. Kennel, C. Jason, P. W. Albro, G. Mason and S. H. Safe, Toxicol. Appl. Pharmacol., 1986, 82, 256 CrossRef CAS.
- L. Stanker, B. Watkins, M. Vanderlaan and W. L. Budde, Chemosphere, 1987, 16, 1635 CrossRef CAS.
- L. Stanker, B. Watkins, N. Rogers and M. Vanderlaan, Toxicology, 1987, 45, 229 CrossRef CAS.
- M. Vanderlaan, L. H. Stanker and B. Watkins, Environ. Toxicol. Chem., 1988, 7, 859 CAS.
- B. E. Watkins, L. H. Stanker and M. Vanderlaan, Chemosphere, 1989, 19, 267 CrossRef.
- M. N. Langley, R. K. Chopra, C. S. Creaser, R. J. K. Taylor, M. D. Rose, J. R. Starlin, H. A. Lee and M. R. A. Morgan, Food Agric. Immunol., 1992, 4, 143 CAS.
- R. O. Harrison and R. E. Carlson, Chemosphere, 1997, 34, 915 CrossRef CAS.
- Y. Sugawara, S. J. Gee, J. R. Sanborn, S. D. Gilman and B. D. Hammock, Anal. Chem., 1998, 70, 1092 CrossRef CAS.
- M. P. Marco, S. Gee and B. D. Hammock, Trends Anal. Chem., 1995, 14, 341 CAS.
- I. Karube, Y. Nomura and Y. Arikawa, Trends Anal. Chem., 1995, 14, 295 CAS.
- K. R. Rogers, Biosens. Bioelectron., 1995, 10, 533 CrossRef CAS.
- M. Minunnni and M. Mascini, Anal. Lett., 1993, 26, 1441.
- A. Brecht, J. Piehler, G. Lang and G. Gauglitz, Anal. Chim. Acta, 1995, 311, 289 CrossRef CAS.
- L. Engel and W. Baumann, Fresenius’ J. Anal. Chem., 1993, 346, 745 CrossRef CAS.
- M. Tommoy, R. L. Baer, D. Spirasolomon and T. P. Doherty, Anal. Chem., 1995, 67, 1510 CrossRef CAS.
- G. G. Guilbault, B. Hock and R. Schmid, Biosens. Bioelectron., 1992, 7, 411 CrossRef CAS.
- C. Steegborn and P. Skladal, Biosens. Bioelectron., 1997, 12, 19 CrossRef CAS.
- R. C. Ebersole and M. D. Ward, J. Am. Chem. Soc., 1988, 110, 8623 CrossRef CAS.
- E. Grabbe and R. Buck, J. Electroanal. Chem., 1987, 223, 67 CrossRef CAS.
- M. Thompson, C. Arthur and G. Dhaliwal, Anal. Chem., 1986, 58, 1206 CrossRef CAS.
- R. M. Carter, M. B. Jacobs, G. J. Lubrano and G. G. Guilbault, Anal. Lett., 1995, 28, 1379 CAS.
- M. Minunni, M. Mascini, R. M. Carter, M. B. Jacobs, G. J. Lubrano and G. G. Guilbault, Anal. Chim. Acta, 1996, 335, 169 CrossRef CAS.
- K. Yun, E. Kobatake, T. Haruyuma, M. Laukkaned, K. Keinanen and M. Aizawa, Anal. Chem., 1998, 70, 260 CrossRef CAS.
- A. Shons, F. Dorman and J. Najarian, J. Biomed. Mater. Res., 1972, 6, 565 CrossRef CAS.
- E. Prusak-Sochaczewski and J. H. Luong, Enzyme Microb. Technol., 1990, 12, 173 CrossRef CAS.
- J. Rickert and A. Brecht, Biosens. Bioelectron., 1997, 12, 567 CrossRef CAS.
- J. Horacek and P. Skladal, Anal. Chim. Acta, 1997, 347, 43 CrossRef CAS.
- S. Storri, T. Santorini, M. Minunni and M. Mascini, Biosens. Bioelectron., 1998, 13, 347 CrossRef CAS.
- R. Vaughan, C. K. O’Sullivan and G. G. Guilbault, Fresenius’ Z. Anal. Chem., 1999, 364, 54 CrossRef.
- C. R. Suri, P. K. Jain and G. C. Mishra, J. Biotechnol., 1995, 39, 27 CrossRef CAS.
- M. Plomer, G. G. Guilbault and B. Hock, Enzyme Microb. Technol., 1992, 14, 230 CrossRef CAS.
- I. S. Park and N. Kim, Biosens. Bioelectron., 1998, 13, 1091 CrossRef CAS.
- M. Másson, K. S. Yun, T. Haruyama, E. Kobatake and M. Aizawa, Anal. Chem., 1995, 67, 2212 CrossRef CAS.
- S. J. Vigmond, M. Jwakura, F. Mizutani and T. Katsura, Langmuir, 1994, 10, 2860 CrossRef CAS.
- F. Alberl, H. Wolf, C. Kösslinger, S. Drost, P. Woias and S. Koch, Sens. Actuators, B, 1994, 18, 271 CrossRef.
- B. König and M. Grätzel, Anal. Chim. Acta, 1995, 309, 19 CrossRef.
- J. R. Sanborn, S. D. Gilman, S. J. Gee, Y. Sugawara, A. D. Jones, J. M. Stotamire, M. S. Denison and B. D. Hammock, J. Agric. Food. Chem., 1998, 46, 2407 CrossRef CAS.
- T. Nomura, M. Watanabe and T. M. West, Anal. Chim. Acta, 1985, 175, 107 CrossRef CAS.
- G. Sauerbrey, Z. Phys., 1959, 155, 206 Search PubMed.
- A. J. Lomant and C. Fairbanks, J. Mol. Biol., 1976, 104, 243 CrossRef CAS.
- E. Katz, J. Electroanal. Chem., 1990, 218, 257 CrossRef.
- P. Wagner, M. Hegner, P. Kernen, F. Zaugg and G. Semenza, Biophys. J., 1996, 70, 2052 Search PubMed.
- S. Imabayasji, D. Hobara and T. Kakiuchi, Langmuir, 1997, 13, 4502 CrossRef.
- X. C. Zhou, S. J. O’Shea and S. F. Y. Li, Chem. Commun., 2000, 953 RSC.
|
This journal is © The Royal Society of Chemistry 2001 |