DOI:
10.1039/A907827D
(Paper)
Analyst, 2000,
125, 29-33
Nanobiotechnology and its role in the development of
new analytical devices†
Received 28th September 1999, Accepted 30th November 1999
First published on UnassignedUnassigned7th January 2000
Abstract
Physical methods of molecule observation and manipulation will
prove useful, not only as research tools for investigating biomolecular
structure and behavior, but also for the creation of nanostructures.
Supramolecular and self-assembling structures are able to generate
nanostructures, with many such systems being of biological origin. They
form the interface between nanotechnology and biotechnology. Whereas
biotechnological processes usually involve populations of cells or
molecules, nanotechnological methods operate at the level of individual
molecule manipulation. This article considers what advances have been made
through cross-fertilisation between nanotechnology and biotechnology to
develop for the next millennium new analytical tools at the microscale,
using nanostructures as the sensitive part and with the ability to detect
individual molecules.
The convergence of technologies being developed for machining
materials at the atomic scale with technologies achieved for observing
nanostructures has promoted the emergence and rapid growth of
nanotechnology.Supramolecular and self-assembling structures are able to generate
nanostructures, many of such systems being of biological origin. They form
the interface between nanotechnology and biotechnology.
Many biological systems—including viruses, membranes and protein
complexes—are natural nanostructures. However, they may be analysed
in the same way as non-biological structures, and their characteristics can
be exploited in nanostructure design and development. It is becoming
increasingly obvious that systems that integrate biological and chemical
components with physical devices and electrodes have enormous potential for
application, not only for developing analytical and monitoring devices, but
also for molecular-scale bioreactors. Whereas biotechnological processes
usually involve populations of cells or molecules (or, in some cases,
individual cells or organelles), nanotechnological methods operate at the
level of manipulation of individual molecules.
This article considers what advances have been made through
cross-fertilisation between nanotechnology and biotechnology to develop:
studies of single biological macromolecules (i.e., proteins);
biotransducers in new analytical devices; and micro-total analysis
systems.
A. Studies of single protein molecule
Since many decades ago, characterization of the functional and
structural properties of biological molecules has been made by conventional
techniques that operate on a population of biomolecules. Catalytic
parameters of an enzyme determined by spectrophotometric measurement or the
3D structure of the enzyme obtained from X-ray diffraction are always the
average behavior of an enzyme population. The current hypothesis is to
assume that a homogeneous population of molecules allows the extrapolation
of the results to the single molecule. In the last years, techniques appear
to characterize and manipulate individual biomolecules, such techniques are
more precisely scanning probes and optical techniques (see the recent
reviews1–4). With these
techniques, heterogeneity of populations of molecules has been
identified.Scanning probe microscopy
Over these past years, scanning probe microscopy (SPM) and especially
atomic force microscopy (AFM)5 has been
intensively used to study biological samples at the nanometer scale.
However, at the beginning, these techniques were not applied easily to
biological specimens. Specific problems due to the technique were not known
and some image interpretations were erroneous.6 Today the technique has matured and is reliable,
the artifacts are well known and the image interpretation is generally not
ambiguous. Concerning topographic imaging, some recent advances in sample
preparations, operating conditions, probes and imaging mode have decreased
the resolution to the nanometer level. For biological observations, the tip
curvature radius (typically 10 nm to be comparable with the protein radius,
i.e., albumin has a radius of 4 nm) and the protein compliance
lead to great difficulties in observing the substructures of proteins
adsorbed onto a solid surface. Nevertheless AFM has generated a growing
interest due to the new capabilities it offers. It preserves the native
state of molecules or cells because no sample preparations (fixation,
dehydration, coatings, etc.) are needed and because proteins,
e.g., bovine albumin,7 RNA
polymerase8 or antibody9 can be explored in physiological media. Thus
dynamic processes like antibody–antigen complex formation,10 enzyme activity11 or the DNA transcription12 have been observed. Another important aspect of
AFM is its capability to measure the force between a sharp tip and the
sample surface. This offers the possibility of measuring a wide range of
properties from mechanical properties (elasticity, viscosity, plasticity,
friction, etc.) to forces (attraction, adhesion, interaction,
etc.) using various imaging modes. Nevertheless it is very
difficult to measure quantitatively these properties. Generally, the signal
of the imaging mode is just used to elaborate an image with an arbitrary
unit opening the door to over-interpretation of data. For some imaging
modes it is possible to realize a quantitative image of the tip/surface
interaction (i.e., contact stiffness) but very difficult if not
impossible to realize a quantitative image of the surface properties
(i.e., elastic modulus).The atomic force microscope could be also used as a force-sensing
instrument to measure the tip/surface force as a function of the distance
and more interestingly the interaction energy (which is the integration of
force with respect to distance).13 These
two properties are important because they are responsible for dynamic
behaviors like kinetic or affinity constants. Because the atomic force has
been built for imaging it has several flaws concerning force-sensing
measurement. The most notable of them is that the so-called force curve
describes the tip/surface force as a function of the sample position
instead of the tip/surface distance. One of the solutions to prevent this
phenomena is to achieve an external force on the cantilever (via a
magnet for example) to generate a true force tip/sample distance
curve.14 But this has, to our knowledge,
not been applied to biological experiment. Nevertheless this kind of
experiment has been used to measure the avidin–biotin15–17 or antigen–antibody
interactions18–20 or the
elongation force of titin domains.21
Another interesting evolution of SPM is the development of the near field
scanning optical microscope (SNOM).22–25 SNOM has been successfully used for biological
investigations.26–30 Recent developments of probes25 and of shear force mode31 has decreased the resolution to a few
nanometers.32 Future work could be the
study of the luminescence activity of a single protein.
Optical techniques
Regrouping of Rhodamine molecules adsorbed on Si wafers was revealed as
fluorescent spots with a measurable number of individual molecules.33 Fluorescence of the active site of an enzyme,
i.e., FAD of cholesterol oxidase,34 allows one to follow the turnover of a single
enzyme molecule. From the results of this kind of experiment, a new view of
the population of enzyme molecules with a static and dynamic disorder of
reaction rates appears. Individual enzyme molecules have different
catalytic turnovers35 and a molecular
memory phenomenon exists in the same enzyme molecule so that enzymatic
turnovers are not independent of previous ones.34Recently,36 the rotation of F1 ATPase
during ATP hydrolysis has been observed in an epifluoresence microscope.
Visualization of the motion of the F1 subunit was effected by the
attachment of a fluorescent actin to the F1 ATPase immobilized on a
coverslip. Optical tweezers exploit the fact that light exerts force on
matter. As in AFM, elasticity of macromolecules, i.e.,
titin,37 can be quantified by elongation or
twisting of the molecule by optical tweezers. Optical tweezers also allow
the measurement of the binding force between two biological molecules or
movements. Applications concerned the linear motor proteins that move along
a polymer track such as kinesin, myosin or RNA polymerase.4 Understanding of the mechanisms of the linear
motor proteins allows the possibility of using these proteins as nanomotors
for motions at the nanometer scale.
B. Biotransducers
Many technology applications in analysis require the organization of
atoms or molecules in a two-dimensional space. Two approaches may be used
to create the initial framework. First, as demonstrated by the
microelectronic industry, such frameworks can be generated by the
manufacture of silicon-based materials, which may then be machined with the
desired structure or pattern. This approach will find many applications in
labs-on-a-chip fabrication. Second, it is possible to use the self-assembly
abilities of synthetic and biological molecules. Self-assembled nano- and
microstructures can be generated from natural amphiphilic molecules, and
these molecular self-assemblies may be used directly as potential
biomimetic systems (for more details see ref. 38). This approach is more
related to the fabrication of biochips to combine the sensitivity of a
physical detection and the biological specificity. Because of the
importance of DNA-based assays for detection of diseases, genome sequencing
and environmental contol, a DNA chip is one of the major challenges.Supported layer
Self-assembled monolayers (SAMs) may be generated by the spontaneous
physisorption or chemisorption of molecules onto a surface. Fusion of
phospholipid vesicles on hydrophobic SAM, i.e., octadecyl
trichlorosilane (Fig. 1), results in the
formation of a phospholipid monolayer on a SAM (Fig.
2A). Peripheral enzyme membranes, such as pyruvate oxidase,39 and membrane electron carriers, such as
plastoquinone and ubiquinone,40 can be
incorporated in the supported bilayer at the physiological level (Fig. 1). When this bilayer was laterally in contact
with a built-in gold electrode, lateral diffusion of quinone allows this
electron carrier to shuttle between the electrode and pyruvate oxidase
(Fig. 1). A catalytic current was then
measured which reflected the electrochemical reaction of the quinone on the
gold electrode, the lateral diffusion of the quinone along the bilayer and
the catalytic reaction of the ubiquinone with the enzyme. |
| Fig. 1 Reconstituted biological electron transfer. A lipid monolayer which
incorporated a physiological amount of ubiquinone was added to the
alkylated monolayer by fusion of lipid vesicles before the incorporation of
pyruvate oxidase, a peripheral membrane enzyme. The electron carrier,
ubiquinone, diffused along the biomimetic bilayer and made the shuttle
between the gold electrode and pyruvate oxidase. The catalytic current
measured both the electrochemical reaction of ubiquinone on the gold
electrode, the lateral diffusion of ubiquinone along the alkane–lipid
bilayer and the catalytic reaction of the ubiquinone with pyruvate
oxidase. | |
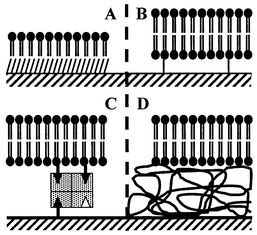 |
| Fig. 2 Schemes of supported lipid layer. A supported lipid monolayer that
allows the incorporation of peripheral membrane proteins is obtained by
fusion of lipid vesicles on the top of an alkylated layer (A). Several
experimental methods were tested to obtain a lipid bilayer compatible with
the incorporation of integral membrane proteins: lipid bilayer tethered by
a hydrophilic spacer (B) or by an avidin–biotin complex (C); lipid
bilayer onto a polymeric layer (D). | |
During the last few years, technological efforts have been made to
increase the efficiency of supported layers with comparison to the
biological membrane. Formation of a new kind of lipid bilayer spaced from
the surface by a linker has been done (Fig.
2). The bilayer was supported on a thin polymer layer41 (Fig. 2D) or
tethered to the surface by a hydrophilic spacer42 (Fig. 2A),
peptide43 or avidin–biotin complex
(Fig. 2C). In the spaced bilayer, an
integral membrane protein such as ATPase43
or rhodopsin42 has been successfully
incorporated. Moreover, the spaced bilayer creates an ionic reservoir
between the bilayer and the support.44 The
ionic permeability of the bilayer can be modulated by the incorporation of
peptides such as valinomycin44 and
gramicidin45 that open the way to a new
class of biocapture using ion-channel switches.46 We can guess that the future biochip based on a
lipid bilayer will integrate membrane receptors to perform a very specific
and very sensible analysis.
2D and 3D imprinting
For some purposes, nanostructures cannot be created by self-assembly
alone. In these cases, a technique called ‘templating’ can be
used in two possible ways: a template can be used to create a more complex
initial pattern for subsequent self-assembly; alternatively, the original
structure can be used as a template that can be modified by chemical or
physical means to stabilize, or tailor, the properties for a specific
purpose (for more details see ref. 38). Molecular imprinting is one example
of the templating approach (Fig. 3). A
‘print’ molecule interacts with a solution of molecules
(Fig. 3A), which is subsequently
‘fixed’ (Fig. 3B) to form an
impression of the print molecule47 (Fig. 3C). Macroscopic polymer matrices are generally
used to create the recognition sites (Fig. 3
upper part). The recognition sites of the polymer are related to a
biomimetic approach to create synthetic receptors48 or synthetic antibodies.49,50 In some cases, the imprint has catalytic properties,
such as ATP hydrolysis.51 Imprinting
polymers appears then as a tool to create synthetic enzymes47 in order to increase the stability of the
catalyst. The imprinting polymer matrices are applied mainly for affinity
chromatography, i.e., for nucleotides,51,52 steroids,48
drugs49 or herbicide analysis.53 A thin film of the imprinting polymer can coat
the surface of a physical transducer to create substrate-selective sensors.
Examples of transducers used in imprinted polymer sensors are various:
capacitance, amperometry, conductometry, potentiometry, pH, fluorescence,
colorimetric, ellipsometry, surface plasmon resonance, surface acoustic
wave and quartz microbalance.54 |
| Fig. 3 Schemes of 2D and 3D molecular imprinting. The different steps for 2D
molecular imprinting (lower part) and 3D molecular imprinting (upper part)
are: specific interactions with the host molecule (A), polymerization or
self-assembly of molecules around the host molecule (B) and removing of the
host molecule from the molecular imprint (C). The molecular imprint has
both specific interaction sites and a specific shape to recognize the host
molecule. | |
Besides macromolecular polymers, 2D imprinting can be done using a SAM
to create functionalyzed monolayers assembled on a surface, i.e.,
with size and shape specific molecular recognition sites (Fig. 3 lower part). Perforated monolayers that
include molecular channels were then tailored and molecular components,
i.e., tetradecanethiol and ‘trans’ quinone for
photochemical imprinting,55 were
co-assembled in two-dimensional monolayers.
DNA chips
DNA-based assays have an increasing importance for detection of
diseases, genome sequencing, forensic and environmental control. They are
challenged by the detection of trace amounts of DNA fragments,
characterized by a specific sequence in a huge background of other
molecules and unspecific DNA. The polymerase chain reaction (PCR) can be
used in conjunction with labeled probes to detect very small amount of DNA.
The following examples illustrate the purpose in a non-exhaustive way
considering the huge research in the field of DNA chips.The diagnostic method is typically based on hybridization of
oligonucleotides probes. Single DNA chips can consist of a matrix of dots
coated with various oligonucleotides that simultaneously detect a range of
DNA sequences. Deposition of DNA or oligonucleotides on the dot surface can
be done by covalent linkage of activated oligonucleotide on amine
functionalized surfaces,56 attachment of
thiol functionalized DNA directly on a gold surface,57 electrochemical polymerization of
oligonucleotide pyrrole,58 intercalation of
DNA with a self-assembled monolayer of acridin,59 specific interaction of biotinylated
oligonucleotide with streptavidin immobilized directly on the
surface60 or on a lipid supported
layer60,61 (Fig. 4).
 |
| Fig. 4 Schemes of oligonucleotide deposition. The deposition of oligonucleotide
on the surface of a DNA chip can be performed in different ways: direct
covalent linkage of the oligonucleotide on the surface (A), electrochemical
polymerization of oligonucleotide pyrrole (C), immobilization with an
avidin–biotin complex directly on the surface (B) or on a lipid
supported bilayer (D). | |
Sensors are mostly based on radioactive labeled probes, fluorescent
probes or electrochemically active probes. Electrochemical probes can be
obtained by the linking of an electroactive unit such as ferrocene62 with the oligonucleotide. The hybridization
event can be also detected electrochemically by the intercalation of
electroactive molecule such as Orange Acridin or daunomycin.63 A peak potential shift results from the
hydrophobic interactions (intercalation) between DNA and intercalators. The
properties of the intercalators to distinguish between double-stranded and
single-stranded DNA are then very important to detect a gene specifically
with high sensitivity. In the case of an oligonucleotide-functionalized
polypyrolle electrode, hybridization decreases the intensity of the current
peak due to the oxidation (or doping) of the polypyrrole chain.58 Using fluorescent probes, such as fluorescein
labeled oligonucleotides,56 a simultaneous
detection of multiple DNA sequences is possible using a fiber-optic
biosensor array system. In this system, several optical fibers were bundled
together each with a different probe immobilized on its tip. With the
possibility to detect single fluorophore molecules, ultra-sensitive
fluorescence techniques allow the detection of individual pairing of
oligonucleotides.60
Another approach is based on the use of peptide nucleic acids
(PNA)64 as a recognition layer in DNA
chips. PNA is a structural DNA analogue with a pseudopeptide backbone that
mimics DNA by forming complementary duplexes with normal DNA. The PNA/DNA
duplexes, that can be done using shorter probes, have higher thermal
stability and can be formed at low ionic strength.
C. Micro-total analysis systems
Micro-total analysis systems (μTAS), also called
‘labs-on-a-chip’, must perform the functions of large
analytical devices in small units. They must contain elements for the
acquisition, pretreatment, separation, post-treatment and detection of the
samples.65 Biochips could be included as a
functional element in a μTAS device but a number of other processes are
necessary to go from reactants to analysis. Several methods exist for
carrying out chemistry confined to well-defined regions of a planar device.
For silicon or glass devices, methods include photolithography based on
beams of light, electrons or ions; these methods have been typically
developed for microelectronics. A network of microfluidic channels can be
generated using an elastomeric polymer, e.g.,
poly(dimethylsiloxane),66,67 in which
analytes are transported, mixed and separated in μTAS. Patterning of
molecules on a reactive surface can be done by microcontact printing of the
active molecule, e.g., alkanethiol on a gold surface,68 with an elastomeric stamp. In this case,
patterning of the surface with a hydrophobic and a hydrophilic SAM can also
create microfluidic channels. Conventional syringe pumps or microfabricated
pumps perform liquid flow in the microfluidic microchannel. Release
mechanism of drugs can be for example based on electrochemical dissolution
of a thin anode membrane covering microreservoirs filled with chemicals in
solid, liquid or gel form.69Strong points of the use of μTAS include the reduction of the
consumption of samples and reagents, reduction of the time of analysis, and
the increase of sensitivity. These high performances of μTAS will be
particularly valuable in the pharmaceutical industry for the screening of
combinatorial libraries, in clinical analyses, in DNA-based diagnostics and
genotyping. Ramsey’s recent work using microchip electrophoresis as a
separation method illustrates the performance. Separations of 15 pM
Rhodamine 6G and 30 pM Rhodamine B (200 pL of solution) were performed
using microchip electrophoresis and were detected by counting fluorescence
bursts from individual molecules.70 A
binary mixture of Rhodamine and dichlorofluorescein were resolved in less
than 1 ms using microchip electrophoresis.71 Polymerase chain reactions were carried out on
as many as four DNA samples at a time on a microchip device and the PCR
products analyzed on the same device by microchip electrophoresis.72
D. Concluding remarks
The first major advances in nanotechnology were done by the
microelectronics industry with the manufacture and machining of silicon
materials. The second breakthrough was the emergence of the discipline of
nanotribology from attempts to understand friction, wear and lubrication at
the atomic scale, and the atomic-force and friction-force microscopes were
developed as investigative tools for this purpose. The third step in
nanotechnology is the convergence between the field and the recent
achievements in biotechnology.There is not only a fertilisation of nanotechnologies through the use of
biotechnologies but also a huge reciprocal impact of the nanoconcepts on
biology.
The acquisition of expertise in these areas will soon become a
prerequisite for those wishing to participate in the next phase of
biotechnological evolution. Nanobiotechnology is important, not only for
its research and development tools, but also for its potential commercial
significance in the development of new analytical devices and preparative
bioreactors.
References
- W. E. Moerner and M. Orrit, Science, 1999, 283, 1670 CrossRef CAS.
- S. Weiss, Science, 1999, 283, 1676 CrossRef CAS.
- J. K. Gimzewski and C. Joachim, Science, 1999, 283, 1683 CrossRef CAS.
- A. D. Mehta, M. Rief, J. A. Spudich, D. A. Smith and R. M. Simmons, Science, 1999, 283, 1689 CrossRef CAS.
- G. Binnig, C. F. Quate and C. Gerber, Phys. Rev. Lett., 1986, 56, 930 CrossRef.
- T. P. Beebe, T. E. Wilson, D. F. Ogletree, J. E. Katz, R. Balhorn, M. B. Salmeron and W. J. Siekhaus, Science, 1989, 243, 370 CAS.
- N. B. Sheller, S. Petrash, M. D. Foster and V. V. Tsukruk, Langmuir, 1998, 14, 4535 CrossRef CAS.
- N. H. Thompson, B. L. Smith, N. Almqvist, L. Schmitt, M. Kashlev, E. T. Kool and P. K. Hansma, Biophys. J., 1999, 76, 1024 Search PubMed.
- D. Anafi and G. M. Wu,
http://www.di.com/Theater/Misc/IgG-Amgen.html.
- A. P. Quist, A. A. Bergman, C. T. Reimann, S. O. Oscarsson and B. U. R. Sundqvist, Scanning Microsc., 1995, 9, 395 Search PubMed.
- M. Radmacher, M. Fritz, H. G. Hansma and P. K. Hansma, Science, 1994, 265, 1577 CAS.
- S. Kasas, N. H. Thompson, B. L. Smith, H. G. Hansma, X. Zhu, M. Guthold, C. Bustamante, E. T. Kool, M. Kashlev and P. K. Hansma, Biochemistry, 1997, 36, 461 CrossRef CAS.
- W. F. Heinz and J. H. Hoh, Trends Biotechnol., 1999, 17, 143 CrossRef CAS.
- S. P. Jarvis, H. Yamada, S. I. Yamamoto, H. Tokumoto and J. B. Pethica, Nature (London), 1996, 384, 247 CrossRef CAS.
- E. L. Florin, V. T. Moy and H. E. Gaub, Science, 1994, 364, 415.
- Y. S. Lo, N. D. Huefner, W. S. Chan, F. Stevens, J. M. Harris and T. P. Beebe, Langmuir, 1999, 15, 4373.
- S. S. Wong, E. Joselevich, A. T. Woodlley, C. L. Cheung and C. M. Lieber, Nature (London), 1998, 394, 52 CrossRef CAS.
- P. Hinterdorfer, W. Baumgartner, H. J. Gruber, K. Schilcher and H. Schindler, Proc. Natl. Acad. Sci. USA, 1996, 93, 3477 CrossRef CAS.
- S. Allen, X. Chen, J. Davies, M. C. Davies, A. C. Dawkes, J. C. Edwards, C. J. Roberts, J. Sefton, S. J. B. Tendler and P. M. Williams, Biochemistry, 1997, 36, 7457 CrossRef CAS.
- U. Dammer, M. Hegner, D. Anselmetti, P. Wagner, M. Dreier, W. Huber and H. J. Guntherodt, Biophys. J., 1996, 70, 2437 Search PubMed.
- M. Rief, M. Gautel, F. Oesterhelt, J. M. Fernandez and H. E. Gaub, Science, 1997, 276, 1109 CrossRef CAS.
- D. W. Pohl, W. Denk and M. Lanz, Appl. Phys. Lett., 1984, 44, 651 CrossRef.
- A. Lewis, M. Isaacson, A. Harootunian and A. Murray, Ultramicroscopy, 1984, 13, 227 CrossRef.
- D. van Laeke, in
Scanning probe microscopy; beyond the images, ed. S.
Gauthier and S. Joachim, Les éditions de physique,
Paris, 1992, pp.
229–275. Search PubMed.
- Ultramicroscopy, 1995, 61,
pp. 1–314. Search PubMed.
- P. G. Haydon, S. P. Marchese Ragona, T. A. Basarsky, M. Szulczewxki and M. McClosky, J. Microsc., 1996, 182, 208 CrossRef CAS.
- T. Enderle, T. Ha, D. F. Ogletree, D. S. Chemla and C. Magowan, Proc. Natl. Acad. Sci. USA, 1997, 94, 520 CrossRef CAS.
- L. A. Gherber, J. Hwang and M. Edidin, Appl. Opt., 1998, 37, 3574 Search PubMed.
- M. F. Garcia Parajo, J. A. Veerman, A. G. T. Ruiter and N. F. van Hulst, Ultramicroscopy, 1998, 71, 311 CrossRef CAS.
- H. Muramatsu, N. Chiba, T. Umemoto, K. Homma, K. Nakajima and T. Ataka, Ultramicroscopy, 1995, 61, 265 CrossRef CAS.
- E. Betzig, P. L. Finn and J. S. Weiner, Appl. Phys. Lett., 1992, 60, 2484 CrossRef CAS.
- F. Zenhausen, Y. Martin and H. K. Wickramasinghe, Science, 1995, 269, 1083 CrossRef.
- M. Ishikawa, O. Yogi, J. Y. Ye, T. Yasuda and Y. Maruyama, Anal. Chem., 1998, 70, 5198 CrossRef CAS.
- H. P. Lu, L. Xun and X. S. Xie, Science, 1998, 282, 1877 CrossRef CAS.
- D. B. Craig, E. Arriaga, J. C. Y. Wong, H. Lu and N. J. Dovichi, Anal. Chem., 1998, 70, 39A CAS.
- H. Noji, Science, 1998, 282, 1844 CrossRef CAS.
- H. P. Erickson, Science, 1997, 276, 1090 CrossRef CAS.
- J. M. Laval, J. Chopineau and D. Thomas, Trends Biotechnol., 1995, 13, 474 CrossRef CAS.
- O. Pierrat, C. Bourdillon, J. Moiroux and J. M. Laval, Langmuir, 1998, 14, 1692 CrossRef CAS.
- D. Marchal, W. Boireau, J. M. Laval, J. Moiroux and C. Bourdillon, Biophys. J., 1998, 74, 1937 Search PubMed.
- J. Majewski, J. Y. Wong, C. K. Park, M. Seitz, J. N. Israelachvili and G. S. Smith, Biophys. J., 1998, 75, 2363 Search PubMed.
- S. Heyse, O. P. Ernst, Z. Dienes, K. P. Hofmann and H. Vogel, Biochemistry, 1998, 37, 507 CrossRef CAS.
- N. Bunjes, E. K. Schmidt, A. Jonczyk, F. Rippmann, D. Beyer, H. Ringsdorf, P. Graber, W. Knoll and R. Naumann, Langmuir, 1997, 13, 6188 CrossRef CAS.
- B. Raguse, V. Braach-Maksvytis, B. A. Cornell, L. G. King, P. D. J. Osman, R. J. Pace and L. Wieczorek, Langmuir, 1998, 14, 648 CrossRef CAS.
- A. T. A. Jenkins, R. J. Bushby, N. Boden, S. D. Evans, P. F. Knowles, Q. Liu, R. E. Miles and S. D. Ogier, Langmuir, 1998, 14, 4675 CrossRef CAS.
- B. A. Cornell, V. L. B. Braach-Maksvytis, L. G. King, P. D. J. Osman, B. Raguse, L. Wieczorek and R. J. Pace, Nature (London), 1997, 387, 580 CrossRef CAS.
- K. Mosbach, Trends Biotechnol., 1994, 19, 9 CAS.
- S. H. Cheong, S. McNiven, A. Rachkov, R. Levi, K. Yano and I. Karube, Macromolecules, 1997, 30, 1317 CrossRef CAS.
- R. J. Ansell, O. Ramstrom and K. Mosbach, Clin. Chem., 1996, 42, 1506 Search PubMed.
- M. Burow and N. Minoura, Biochem. Biophys. Res. Commun., 1996, 227, 419 CrossRef CAS.
- J. Mathew and O. Buchardt, Bioconjugate Chem., 1995, 6, 524 CrossRef CAS.
- K. J. Shea, D. A. Spivak and B. Sellergen, J. Am. Chem. Soc., 1993, 115, 3368 CrossRef CAS.
- J. Matsui, Y. Miyoshi, O. Dobhoff-Dier and T. Takeuchi, Anal. Chem., 1995, 67, 4404 CrossRef CAS.
- K. Haupt and K. Mosbach, Biochem. Soc. Trans., 1999, 27, 344 CAS.
- M. Lahav, E. Katz, A. Doron, F. Patolsky and I. Willner, J. Am. Chem. Soc., 1999, 121, 862 CrossRef CAS.
- J. A. Fergusson, T. C. Boles, C. P. Adams and D. R. Walt, Nature Biotechnol., 1996, 14, 1681 Search PubMed.
- R. Levicky, T. M. Herne, M. J. Tarlov and S. K. Satija, J. Am. Chem. Soc., 1998, 120, 9787 CrossRef CAS.
- H. Korri-Youssouffi, F. Garnier, P. Srivastava, P. Godillot and A. Yassar, J. Am. Chem. Soc., 1997, 119, 7388 CrossRef.
- N. Higashi, M. Takahashi and M. Niwa, Langmuir, 1999, 15, 111 CrossRef CAS.
- W. Trabesinger, G. J. Schutz, H. J. Gruber, H. Schindler and T. Schmidt, Anal. Chem., 1999, 71, 279 CrossRef CAS.
- K. Ijiro, H. Ringsdorf, E. Birch-Hirschfeld, S. Hoffmann, U. Schilken and M. Strube, Langmuir, 1998, 14, 2796 CrossRef CAS.
- S. Takenaka, Y. Uto, H. Kondo, T. Ihara and M. Takagi, Anal. Biochem., 1994, 218, 436 CrossRef CAS.
- K. Hashimoto, K. Ito and Y. Ishimori, Anal. Chim. Acta, 1994, 286, 219 CrossRef CAS.
- J. Wang, E. Palecek, P. E. Nielsen, G. Rivas, X. Cai, H. Shiraishi, N. Dontha, D. Luo and P. A. M. Farias, J. Am. Chem. Soc., 1996, 118, 7667 CrossRef CAS.
- B. H. Weigl and P. Yager, Science, 1999, 283, 346 CrossRef.
- D. C. Duffy, J. C. McDonald, O. J. A. Schueller and G. M. Whitesides, Anal. Chem., 1998, 70, 4974 CrossRef CAS.
- E. Delamarche, A. Bernard, H. Schmid, A. Bietsch, B. Michel and H. Biebuyck, J. Am. Chem. Soc., 1998, 120, 500 CrossRef CAS.
- E. Delamarche, H. Schmid, A. Bietsch, N. B. Larsen, H. Rothuizen, B. Michel and H. Biebuyck, J. Phys. Chem., 1998, 102, 3324 CrossRef CAS.
- J. T. Santini, M. J. Cima and R. Langer, Nature (London), 1999, 397, 335 CrossRef CAS.
- J. C. Fister, S. C. Jacobson, L. M. Davis and J. M. Ramsey, Anal. Chem., 1998, 70, 431 CrossRef CAS.
- S. C. Jacobson, C. T. Culbertson, J. E. Daler and J. M. Ramsey, Anal. Chem., 1998, 70, 3476 CrossRef CAS.
- L. C. Waters, S. C. Jacobson, N. Kroutchinina, J. Khandurina, R. S. Foote and J. M. Ramsey, Anal. Chem., 1998, 70, 5172 CrossRef CAS.
Footnote |
† Presented at SAC 99, Dublin, Ireland, July 25–30,
1999. |
|
This journal is © The Royal Society of Chemistry 2000 |
Click here to see how this site uses Cookies. View our privacy policy here.