DOI:
10.1039/D0RA01084G
(Review Article)
RSC Adv., 2020,
10, 19309-19336
Nanotechnology-based approaches for food sensing and packaging applications
Received
4th February 2020
, Accepted 22nd April 2020
First published on 20th May 2020
Abstract
The rapid advancement of nanotechnology has provided opportunities for the development of new sensing and food packaging solutions, addressing long-standing challenges in the food sector to extend shelf-life, reduce waste, assess safety and improve the quality of food. Nanomaterials can be used to reinforce mechanical strength, enhance gas barrier properties, increase water repellence, and provide antimicrobial and scavenging activity to food packaging. They can be incorporated in chemical and biological sensors enabling the design of rapid and sensitive devices to assess freshness, and detect allergens, toxins or pathogenic contaminants. This review summarizes recent studies on the use of nanomaterials in the development of: (1) (bio)sensing technologies for detection of nutritional and non-nutritional components, antioxidants, adulterants and toxicants, (2) methods to improve the barrier and mechanical properties of food packaging, and (3) active functional packaging. The environmental, health and safety implications of nanomaterials in the food sector, along with an overview of regulation and consumer perception is also provided.
1 Introduction
Nanoscale materials and devices have been heavily explored in the last 20 years. It is well known that materials with dimensions below 100 nm, commonly referred to nanomaterials, provide high surface area, enhanced reactivity, and unique mechanical and optoelectronic properties, which has enabled significant advances in fields such as electronics, medicine, foods, cosmetics and energy devices, among others.1 Several nanoscale materials such as fat globules micelles, whey proteins in milk, and nanosized particles resulting from food digestion exist naturally in food products.2 Although the nanotechnology field is well developed, its application in the food sector is relatively recent. The novel properties of nanomaterials, e.g. mechanical strength, solubility, diffusivity, optical features, etc., have attracted the attention of the scientific community who has begun to investigate their potential to develop technologies to increase product shelf life and freshness, design methods and tools for rapid in situ analysis, and provide environmentally safe and degradable packaging for food. The applications of nanotechnologies in food can be categorized into three groups: nanomaterials as food ingredients for food processing, nanosensing, and nano-enabled packaging. Nanomaterials can be used as nutrients carriers, additives or antimicrobial agents in food packaging. Their optoelectronic properties can be used as sensors and transducers to assess the quality of food, while their mechanical strengths and durability can improve the quality of packaging.
The main nanomaterials used in consumer products are silver nanoparticles (AgNPs), titanium dioxide (TiO2) and silicon dioxide (SiO2) NPs.3 SiO2 NPs were used to develop a light, strong, heat resistant plastic (Durethan) with gas barriers properties to prevent food spoilage and prolong shelf-life.4 The European Food Safety Authority (EFSA) approved three nanomaterials: titanium nitride NPs (TiN NPs) to prevent CO2 leakage from carbonated drinks in polyethylene terephthalate (PET) bottle,5 carbon black and SiO2 NPs for use in food packaging; however no specific migration limits have been set.6 Products that contain processed, produced or packaged nanotechnology tools are commonly referred as nano-food.7 Integration of nanomaterials in food serves different purposes: (1) nanoencapsulation to protect and improve stability of beneficial ingredients, nutrients or nutraceuticals (e.g. vitamin D encapsulated in casein micelle)8–11 for fortifying food products (e.g. fish oil encapsulated in nano-liposomes for fortification of yogurt);12 (2) coating surfaces with active nanomaterials to provide protective functions (e.g. AgNPs for antibacterial packaging13); (3) fillers to improve mechanical properties of packaging (e.g. nanoclay14); (4) sensing materials to develop methods for in situ monitoring of food quality and safety, ideally attached to or included in packaging (e.g. TiO2 for O2 sensing15).
Herein, we discuss the most representative classes of nanomaterials used to develop sensors for food analysis such as nutrients, contaminants (e.g. adulterants, pesticides residues), and microbial contamination. Furthermore, we discuss incorporation of nanomaterials in food packaging, e.g. active packaging, smart packaging and biodegradable packaging. Moreover, we highlight the risks and benefits associated with the use of nanotechnology in the food sector and conclude with an outlook to possible future research in this field. Scheme 1 summarizes the content of this paper highlighting sensing, smart, responsive and biodegradable packaging, as well as a perspective on risk, safety and consumer perceptions.
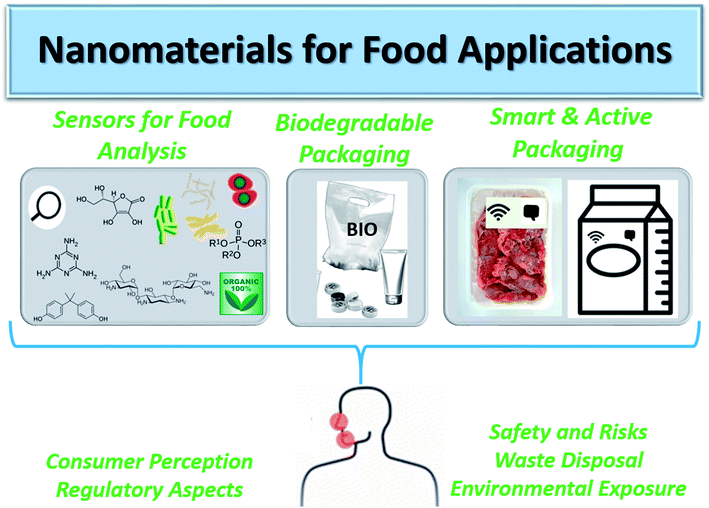 |
| Scheme 1 Summary of the applications of nanomaterials in the food sector. | |
2 Nanotechnology-based sensors for food analysis
Food safety monitoring is very important throughout the food production and supply chain. Today, most food analysis is performed in centralized labs with conventional laboratory equipment and only a limited number of samples can be tested. Rapid, inexpensive and portable assays would enable food producers, storage facilities and consumers to monitor food quality and safety during transportation, storage and use. The incorporation of nanomaterials has enabled the development of a variety of sensing platforms that can monitor food at the source. These platforms are based on either chemical transduction mechanisms, designed primarily for measuring volatile compounds, or on bimolecular recognition for increased selectivity, e.g. biosensors. The biosensors contain a biological receptor (enzymes, aptamers, cells, antibodies, etc.) that can specifically recognize a selected target, and the binding process is transduced by electrochemical, optical, mass or thermal processes. Most reported biosensors are those based on optical or electrochemical processes, e.g. color and electrical current change.
Biosensors have been reported that can detect pathogens (e.g. E. coli, Salmonella),16–18 contaminants (e.g. heavy metals,19 residual veterinary antibiotics,20 residual pesticides, illegal additives,21–24 mycotoxins, bisphenol A25–28 etc.), allergens29 and nutrients (e.g. antioxidants30,31) with potential to overcome the limitations of costly and laborious laboratory techniques. Nanomaterials that are commonly used in these platforms include: (i) Au NPs, AgNPs that have surface platform resonance (SPR) properties and high conductivity enabling them to be used in optical and electrochemical sensors, (ii) magnetic NPs such as Fe2O3 NPs that provide magnetic functions for the efficient separation and enrichment of the targeted analytes,32 (iii) carbon nanotubes and graphene based nanomaterials that amplify electrochemical signals due to their high electrical conductivity. The following section discusses the utilization of various nanomaterials in electrochemical and optical (bio)sensors for food analysis and provides examples of applications.
2.1 Nutrients (antioxidants and sugars)
Antioxidants are well-known for their beneficial health effects (Kaur and Kapoor 2001; Higdon and Frei 2003) by providing protection against excessive free radical accumulation, which has been associated with the pathophysiology of diseases such as cancer, pulmonary, rheumatoid and neurodegenerative diseases, as well as ageing (Harman 1956; McCord 1985; Dröge 2002; Valko et al. 2007). Robust methods for quantification of antioxidants are needed for determining the antioxidants content in food. Methods with enhanced portability, sensitivity and cost have been developed in recent years by implementing the unique properties of nanosize materials. Most assays are based on gold and silver nanostructures (e.g. NPs, nanorods, composites) interfaced with electrochemical or spectroscopic transduction. Other platforms use cerium dioxide (CeO2), TiO2, or carbon-based materials like graphene and carbon nanotubes, which provide high surface area, conductivity and stability making them appealing for use in portable assays.
Electrochemical sensors utilize electrodes modified with conductive or catalytic nanostructures that can directly quantify antioxidants at their characteristic oxidoreduction potential. AuNPs electrodeposited on a glassy carbon electrode (GCE) enhanced electrochemical signals for detection of three antioxidants: butylated hydroxyanisole (BHA), butylated hydroxytoluene (BHT) and butylated hydroquinone (TBHQ). The AuNPs-modified electrode showed a shift in the anodic and cathodic peaks with significant enhancement of peak current as compared to the bare GCE (Fig. 1).30,33
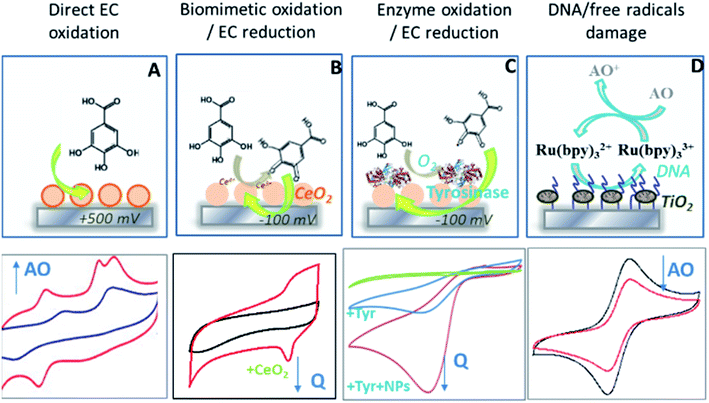 |
| Fig. 1 Summary of electrochemical approaches for measuring antioxidants by direct oxidation using metal NPs or carbon nanotubes to amplify electrochemical (EC) signals (A), biomimetic oxidation with enzyme-like catalysts (CeO2 NPs) (B) or enzymes (tyrosinase) (C) followed by EC reduction of the quinone at −100 mV, and by quantifying reduction of free radicals-induced DNA damage inhibited by antioxidants where TiO2 NP are used to produce free radicals (D) (schematics summarizing detection platforms reported in ref. 30, 33, 36 and 37). | |
Other sensors utilize enzymes like laccase or tyrosinase to biocatalytically convert antioxidants, particularly those with phenolic structures to their quinones.34 The generated quinones are measured by electrochemical reduction at a low applied potential (∼−100 mV), where interferences from co-existing electroactive species are minimal. Nanomaterials such as carbon nanotubes are added to the enzyme immobilization matrix to increase detection sensitivity towards polyphenolic compounds such as Trolox.35 The two-step biochemical-electrochemical process can be represented by the following reaction, where HQ2 and Q are the reduced and oxidized forms of the phenolic antioxidants.
QH2 + 1/2Otyrosinase2 → Q + H2O biochemical reaction (tyrosinase-catalyzed process) |
O2 + 4H+ + 4e− → 2H2O electrochemical reaction |
A growing trend is to replace enzymes with biomimetic nanomaterials possessing enzyme-like properties to catalytically convert these compounds to provide increased stability and low cost as compared to traditional biological catalysts. Such an example is nanoceria, a NP system that has oxidase-like activity, enabling catalytic conversion of common phenolic antioxidants such as caffeic acid, gallic acid and quercetin to their corresponding quinones, followed by their electrochemical detection in the concentration range from 1 to 100 μM. A low cost disposable sensor based on these materials showed good functionality and the ability to assess the antioxidant content in wine.36
In an alternative design, DNA was used as target to measure the oxidative damage induced by free radicals. The biosensor principle is based on the damage of guanine triggered by hydroxyl radical (˙OH) produced by Fenton reaction. The reaction is inhibited if antioxidant compounds are present. DNA was electrodeposited on graphene nanoribbon (GNR) and the biosensor was used for the estimation of total antioxidant capacity (TAC) in fruit juice. The biosensor showed improved analytical performance compared to bare electrodes that were attributed to the enhanced surface area and good electrochemical properties of the GNR.37 In this design, the signal was measured electrochemically using a redox mediator, tris-2,2′-bipyridine ruthenium(II) [Ru(bpy)32+], and the free radicals were generated with TiO2 NPs.38
A summary of the different chemical and biological approaches used to construct biosensors for measuring antioxidant compounds is shown in Fig. 1. Metal or metal oxide NPs such as AuNPs and carbon-based nanostructures like carbon nanotubes or graphene are used as electrode materials to amplify electrochemical signals.
Several types of chemical and biological sensors have been reported to measure the glycemic index (GI) of sugars in food. The GI is a tool used to rate carbohydrate-containing foods that cause an increase in blood glucose levels. The higher the index the faster the release and the larger the blood glucose concentration.39 Controlling the sugar content in food is necessary due to the health issues which are related to sugar (glucose, sucrose, fructose etc.) consumption such as obesity and diabetes. Glucose sensors have been heavily used since the development of the first generation enzymatic glucometer in 1962.40,41 The first three generations of glucometers are dependent on glucose oxidation by glucose oxidase, or glucose dehydrogenase. A fourth generation non-enzymatic glucose sensors was proposed in which enzymes are replaced by nanoparticle catalysts.41 Non-enzymatic sensors provide more affordable platforms that tend to avoid the instability issues associated with enzymes. The main nanomaterial used for these applications is copper. For example, a graphene–Cu NPs nanocomposite was synthesized in situ and mixed with paraffin oil. The mixture was packed in a fused silica capillary tube42 and the tube was used as an electrode to sense several carbohydrates (mannitol, sucrose, lactose, glucose and fructose). The detection mechanism at the Cu electrodes involves deprotonation of carbohydrates (enediol form) in alkaline media, followed by adsorption on the Cu surface, and the electrochemical oxidation of Cu2+. The use of graphene in the graphene–Cu NPs nanocomposite was shown to enhance the detection sensitivity. The limits of detection (LOD) were 0.29, 0.42, 0.43, 0.37, and 0.51 μM for mannitol, sucrose, lactose, glucose, and fructose, respectively. The sensor was successfully used to test the carbohydrate levels in bovine milk and banana. Another study utilized carbon nanotubes (CNTs)-Cu NPs to evaluate mannitol, sucrose, glucose, and fructose based on a similar mechanism.43 The resulted LOD were very close to the ones reported in the previous study with LODs of 0.18, 0.42, 0.40 and 0.62 μM for each respectively. A disposable paper-based electrochemical sensor for carbohydrate detection was developed by immobilizing Cu NPs on paper, which was used as a sensing platform.44 The amperometric sensor provided a low-cost method for the determination of the total carbohydrate. The sensor was fabricated by depositing a conductive ink containing Cu NPs, graphite and polystyrene on paper. Using this design, glucose, fructose and sucrose were successfully determined in soft drinks.
A simplified more convenient analysis of nutraceuticals, antioxidants and sugars was achieved using colorimetric sensors. Colorimetric approaches employ metal or metal oxide NPs that have spectral properties and localized surface plasmon resonance (LSPR) and produce an optical shift in presence of antioxidants through surface reactions, changes in NP size (e.g. target-induced aggregation) or redox processes. Au or AgNPs are the most known to generate color shifts from red to blue for Au, and yellow to brown for Ag due to changes in the LSPR signal.45,46 Szydlowska-Czerniak et al. evaluated the ability of antioxidants to reduce silver ions to AgNPs, and measuring the LSPR of the resulting NPs at 405 nm, as in Fig. 2 (shown for sinapic acid):
 |
| Fig. 2 The oxidation of Ag+ with the antioxidant sinapic acid resulted in production of AgNPs which can be detected spectrophotometrically. Adapted from ref. 47 with permission from Royal Society of Chemistry, Copyright 2012. | |
The method was tested for assessing the antioxidant capacity of 15 varieties of rapeseed oils and its performance was compared to the conventional FRAP, DPPH, and FC methods showing linear correlations.46 The method uses silver nitrate which is a more stable reagent than the reagents used in FRAP, ABTS, and DPPH methods, and the detection procedure does not involve expensive reagents or specialized instrumentation. Using a similar principle, morphological changes of Au nanocage to Au@Ag nanobox were reported in presence of gallic acid antioxidant as a reducing agent for AgNO3. These structural changes resulted in a significant shift of LSPR properties (Fig. 3), enabling detection of antioxidants in a concentration-dependent manner.48 This assay was interfaced with a cellphone reading and its applicability was demonstrated for detection of the total antioxidant content in green tea showing remarkable color change and detection sensitivity.
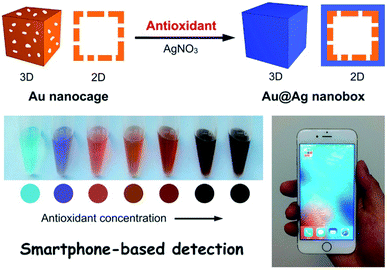 |
| Fig. 3 The sensing principle of antioxidant based on the morphological change of An nanocage upon the reduction of AgNO3 producing Au@Ag nanobox in presence of antioxidants. Adapted from ref. 48 with permission from Royal Society of Chemistry, Copyright 2018. | |
Other optical methods have been designed based on metal oxide NPs such as CeO2 that have unique redox reactivity towards antioxidant compounds via a combined redox and surface attachment processes. These interactions generate formation of charge transfer complexes of characteristic colors, as shown in Fig. 4 for caffeic acid. The spectral changes vary depending on the oxidation potential, the structure and degree of hydroxylation of the antioxidant compound.31 Sharpe et al. (2013) developed a portable paper-based sensor to detect antioxidants in tea and mushrooms.31 The sensor was fabricated on paper, which was used as support for the immobilization of the CeO2 NPs probes. CeO2 NPs have dual oxidation state of (3+ and 4+), reacting with antioxidants and producing a colorimetric change in presence ascorbic acid, gallic acid, vanillic acid, quercetin, caffeic acid, and epigallocatechin gallate. When combined with other metal oxides, the method provided a library of antioxidant signatures enhancing detection accuracy and precision.49
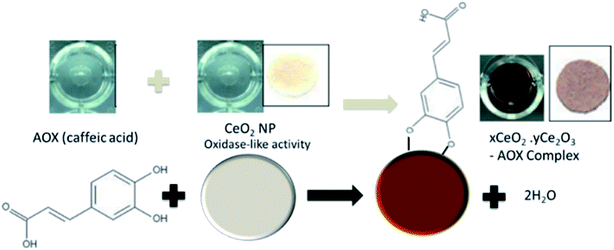 |
| Fig. 4 Reaction mechanism of CeO2 NPs with antioxidants for the colorimetric detection of antioxidants using caffeic acid as an example. In presence of antioxidants, the color of the NPs change from white/yellowish to dark brown due to surface oxidation and formation of charge transfer complexes. Adapted with modification from ref. 31 and a permission from Royal Society of Chemistry, Copyright 2013. | |
Other sensors employ MnO2 nanostructures that are known for their oxidase-like activity. A colorimetric sensor based on MnO2 nanosheets was fabricated for detection of ascorbic acid in orange fruit and orange juice. Single layered MnO2 nanosheets exhibited oxidase like activity, as indicated by the oxidation of 3,3′,5,5′-tetramethylbenzidine (TMB) (colorless) to (TMB ox) (blue). The oxidation activity was diminished in the presence of ascorbic acid, which converts Mn in MnO2 nanosheets to Mn2+. The sensor provided a sensitive, selective and low-cost platform for ascorbic acid detection.50
2.2 Toxins
The presence of toxins such as pesticides, food-borne toxins and heavy metals in food are recognized risks to human health. Possible risks are also posed by the leaching of materials from materials used in packaging, causing unintended exposure. An example is bisphenol A (BPA), a chemical used in the manufacture of polycarbonate and epoxy resin. The monomer is used in the fabrication of a variety of food packaging such as water bottles, feeding bottles and coating material for processed food cans.36,51 In the US, FDA has banned the use of polycarbonates in baby bottles and spill-proof cups and the use of BPA-based coatings in packaging for infant milk in 2012.36,37
BPA is electrochemically active and can be determined by direct oxidation at electrode surfaces. Electrodes can be modified with metal NPs or carbon-based nanomaterials to enhance detection capabilities. A GCE modified with multiwalled carbon nanotubes (MWCNTs) and a molecularly imprinted polymer (MIP) enabled sensitive electrochemical detection of BPA25 in which the MWCNT is used as a conductive layer and the MIP provides a specific site for BPA binding. The method demonstrated applicability for quantifying BPA in baby feeding bottles. An electrochemical sensor for BPA was also developed by modifying GCE with acidic functionalized MWCNTs and AuNPs composite (AuNP/MWCNTs/GCE).27 The oxidation current of BPA increased 40% after modification of the electrode with AuNPs, 124% after modifying the electrode with MWCNT (MWCNTs/GCE), and a huge increase of 278% when both AuNP and MWCNTs were used. The method demonstrated high sensitivity and reproducibility for BPA quantification in water. Other nano-based approaches for BPA detection are to use NPs such as Au, Ni or Fe2O3 functionalized with tyrosinase, which converts the BPA to quinone (Fig. 5). The NPs catalytically amplify the reduction of quinone. Both the enzyme and the NPs were immobilized onto the surface of a low cost disposable screen printed electrode. The NP biosensor showed high reproducibility and reusability for up to 100 assays. Of the three NPs tested, Ni NPs provided the highest sensitivity for BPA, with a detection limit of 7.1 × 10−9 M.33
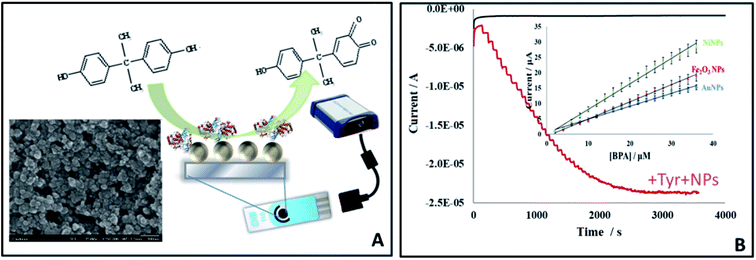 |
| Fig. 5 Enzyme-based detection of BPA by NP amplification using screen printed electrodes modified with Au, Ni or Fe2O3 NPs: sensor design (A) and amperometric response with comparative calibration curve (B) showing differential responses to the three types of NPs of which Ni NPs showed the highest sensitivity (schematic summarizing detection platform and results reported in ref. 33). | |
Among chemical hazards in food, toxic fungal metabolites such as mycotoxins pose particular challenges due to their extremely high toxicity at low exposure levels. Currently, there are no suitable methods for field detection of mycotoxins, making early identification of a possible contamination difficult. It is estimated that at least 25% of the grain produced worldwide is contaminated with mycotoxins. Even small concentrations of mycotoxins can induce significant health problems including vomiting, kidney disease, liver disease, cancer and death.52 Ochratoxin A (OTA) is a nephrotoxic toxin, produced by Aspergillus ochraceus and Penicillium verrucosum, with strong carcinogenic effects on rodents, as well as documented teratogenic and immunotoxic effects in human.53,54 OTA is one of the most abundant food-contaminating mycotoxin in the world. Bülbül et al. (2016) developed a CeO2 NPs based sensor to detect ochratoxin A (OTA).55 The biosensor was assembled by functionalizing CeO2 particles with OTA specific ssDNA aptamers resulting in higher dispersibility and activity. Changes in the redox properties at the CeO2 surface upon binding of the ssDNA and its target, measured using TMB enabled rapid visual detection of OTA. In the presence of OTA, the ssDNA aptamer changed its structure from loose random coils to a compact tertiary form following target binding. As a result, a decreased catalytic effect against TMB oxidation is observed. The system was able to detect as low as 0.15 nM OTA. These changes resulting from ssDNA and target-induced assembly in response to the target demonstrate that aptamer recognition on the surface of CeO2 is feasible and can provide a quantitative measure of aptamer targets.
2.3 Adulterants
Exogenous compounds such as dyes are added to change properties and appearance of food. Among adulterants, food dyes such as Sudan I utilized for food coloring, are the most popular. Concerns about the carcinogenic and mutagenic effects of these azo dyes have resulted in the classification of these dyes as a third category carcinogen by the International Agency for Research on Cancer (IARC).21 In 2005, over 570 products containing chili powder were found contaminated with the dye leading to an international ban on adding this dye to foodstuff.21 A combination of nanomaterials of palladium/gold core–shell nanocrystallines (Pd/Au CSNs) and polyamidoamine dendrimers-cadmium sulfide quantum dots CdSeQDs-PAMAM was used for signal amplification in an electrochemiluminescent (ECL) immunosensor for Sudan I detection.22 GNRs/GO was also used to modify the GCE surface and serve as a platform for the immobilization of Sudan I antibodies. The detection mechanism was based on a competitive immunoassay where Sudan I was applied to the surface of the modified electrode followed by addition of a QDs-PAMAM-Pd/Au CSNs-labeled antibody probe. After incubation and washing ECL measurements were carried out in PBS containing 0.1 M K2S2O8. The ECL signal decreased with increasing Sudan I concentration over a wide linear range and the method showed high sensitivity. A procedure for semi-quantitative screening of Sudan I was also reported using a portable colorimetric immuno-dip strip functionalized with nanocolloidal Au.23 The strip was prepared on a nitrocellulose membrane coated with Sudan I antigen (OVA-S1). Dipping the strip in Au nanocolloid labelled with Sudan I monoclonal antibody (Mab) and Sudan I mixture allowed binding of unbound Au-Mab conjugate with OVA-S1 creating a visual red signal within 10 min. The method was tested on tomato sauce and chili powder samples, showing promising performance for real sample analysis.
Dopamine powder is added to animal feed to increase the muscle building of livestock. Because dopamine is linked to many neurological and psychiatric diseases such as Parkinson's disease, and schizophrenia, the use of dopamine for such purposes has been prohibited in many countries.56 Our group has reported several colorimetric assays that use redox active nanoceria where dopamine was found to be oxidized by the CeO2 NPs and the oxidative reaction product resulted formed a colored charge transfer complex that can be easily monitored spectroscopically.57,58 The method was evaluated for measuring dopamine in aqueous media and human serum. Moreover, CeO2 and TiO2 NPs mix were co-immobilized with tyrosinase and used to develop an electrochemical biosensor for dopamine.59 The tyrosinase enzyme catalyzes the oxidation of dopamine and the CeO2/TiO2 NPs provided a catalytic amplification of the electrochemical signal, enhancing the sensitivity. The LOD of this biosensor was 1 nM.
Another adulterant that is commonly used in milk is melamine, which is added to provide an apparent increase in the protein content.60 In 2008, more than 290
000 infants were affected in China due to milk adulteration by melamine.61 The incident raised significant safety issues and raised the need to detect melamine in milk. Oh, Seo Yeong, et al. 2019 developed a LSPR sensor chip for detecting melamine in milk products using UV-Vis spectrophotometry.24 To prepare the plasmonic chip, AuNPs were immobilized on a glass substrate (Fig. 6) with p-nitroaniline (p-NA) as a chemical receptor. The sensor showed high sensitivity to melamine when tested in commercial milk powder samples.
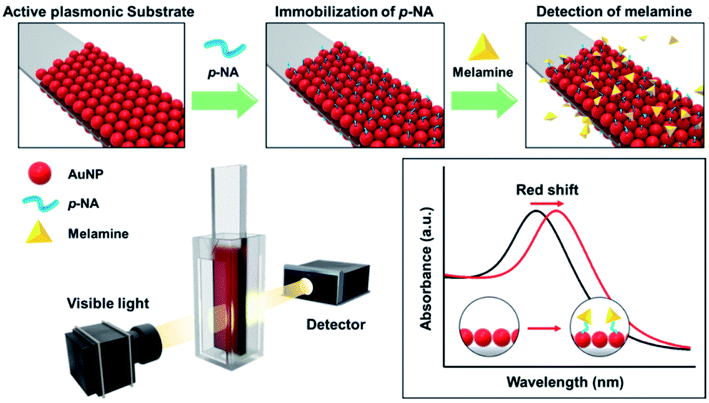 |
| Fig. 6 Schematic illustration for melamine detection with functionalized plasmonic chip (adapted from ref. 24, open access). | |
2.4 Residual veterinary antibiotics and pesticides
Veterinary drugs used for animal diseases treatment and growth enhancement such as antimicrobial drugs, antiparasitic drugs and growth promoters can accumulate in animal meat and animal derived products such as dairy and eggs. The residual amounts of drugs raise safety concerns of which the development of antibiotic resistance is the most important.20 Methods for rapid evaluation of the residual antibiotic content are currently needed to evaluate the antibiotic presence at the point of use. A SERS based Au@AgNPs aptasensor with a DNA aptamer covalently immobilized on AuNPs, followed by coating with Ag shell was reported for kanamycin detection in milk.62 A Raman Cy3 reporter that hybridize with probe DNAs by complementary base pairing was used. Results showed that increasing kanamycin concentration results in a decreased Raman intensity of the Cy3 signal. A detection limit of 0.9 pg mL−1 and recoveries of 90.4–112% were reported. Other sensors were tested on 26 type of sulfonamides antibiotics in honey using a AuNPs immune-based lateral flow device.63 The lateral flow strip was fabricated using a group-specific monoclonal antibody (mAb) conjugated to AuNPs. The performance of the sensor was tested with commercial honey samples and validated with HPLC, and the sensor showed comparative results. The detection limits for sulfonamides such as sulfamethoxazole, sulfamethizole and sulfadiazine ranged from 0.25, to 10 μg kg−1, depending on the antibiotic.
Pesticides are intensively used in agriculture to protect crops and increase food production but their toxicity pause human health risks. Pesticides include a wide range of chemicals, e.g. insecticides, fungicides, herbicides and plant growth regulators. Upon application, residual pesticides remain in plants, soil and water leading to long term exposure and serious human health risks.64 Organophosphate pesticides such as chlorpyrifos, fenthion and methyl parathion were detected by a non-enzymatic approach using CuO nanostructures grown in situ over indium tin oxide ITO.65 The detection principle is based on the inhibition of pralidoxime chloride (PAM) coated over the pimelic acid functionalized CuO nanostructures on the ITO surface. The in situ assembly of the nanostructure provided enhanced surface area and loading, hence enhanced signal. The method was demonstrated for detection of chlorpyrifos in cabbage and spinach extracts. More recently, a disposable, printed graphene-based biosensor was developed for organophosphates detection.66 The electrode was modified through inkjet mask-less lithography (IML) and fabricated by patterning graphene with the IML technique, followed by laser annealing, electrodeposition of PtNPs, and finally drop casting of a phosphotriesterase ink (Fig. 7). Pt NPs were further used to increase surface area, conductivity and enzyme loading. The biosensor provided rapid and sensitive detection of paraoxon in soil and water samples. Methods based on acetylcholinesterase (AChE) inhibition are also popular and have been reported in conjunction with a variety of nanomaterials, in their vast majority MWCNTs or graphene.67 Other examples of nanotechnology approaches for pesticide sensing can be found in reviews dedicated to this topic.68,69
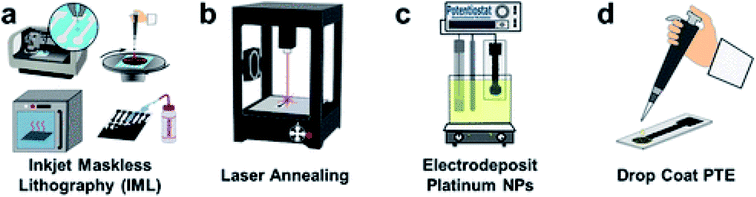 |
| Fig. 7 Schematic representation of the fabrication process of PtNP-IML-PGE biosensor. First graphene is patterned by IML (a) followed by annealing with laser (b) electrodeposition of Pt NPs (c) and drop-coating of PTE ink (d). Reprinted with permission from ref. 66. Copyright (2018) American Chemical Society. | |
2.5 Pathogens
Approximately 48 million people are affected by diseases caused by bacteria, viruses and parasites according to statistics and reports issued by WHO Foodborne Disease Burden Epidemiology Reference Group (FERG).70 Pathogenic bacteria such as Salmonella, Campylobacter spp. and Escherichia coli (E. coli)71 are responsible for most outbreaks.72–75 The availability of rapid analytical methodologies that can identify pathogens in a timely manner would present a significant improvement over conventional approaches that are cumbersome, time and labor intensive.76 Nano-based sensing approaches have demonstrated improvements in miniaturization, increased sensitivity and portability. Main platforms are based on concepts that incorporate immuno and aptamer recognition with MWCNTs, graphene, quantum dots (QD), Au or AgNPs, phage-based approaches, MIPs and antimicrobial peptides.77 Recent works by Zhong, Miao, et al. (2019) reported the use of cadmium sulfide (CdS) QDs confined within a metal organic framework (zeolitic imidazolate framework-8 (ZIF-8)) for electrochemical immuno detection of E. coli O157:H7 (ref. 78) with the CdS@ZIF-8 used as an amplification tag. To introduce specificity, the CdS@ZIF-8 particles were decorated with polyethyleneimine (PEI) which facilitates attachment of E. coli specific antibodies CdS@ZIF-8@PEI-Ab. E. coli detection was achieved using a sandwich assay with measurement of the Cd(II) leaching, quantified with differential pulse voltammetry. The use of ZIF-8 enhanced the detection limit (3 CFU mL−1) by 16 times compared to the ZIF-8 free sensor (Fig. 8).
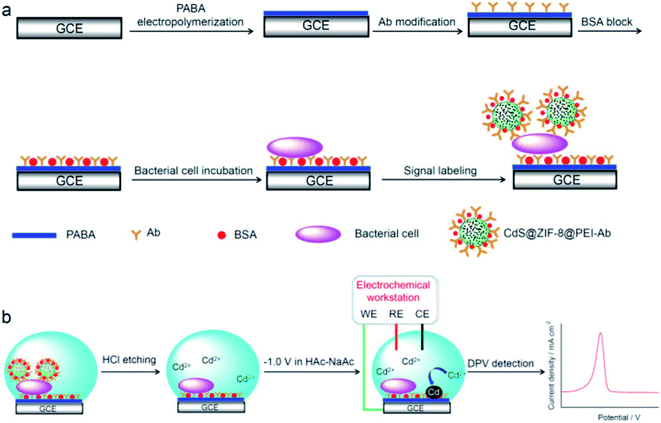 |
| Fig. 8 (a) Fabrication of sandwiched electrochemical immunosensor for detection of E. coli: GCE was modified with poly(p-aminobenzoic acid) (PABA) followed by anti-E. coli O157:H7 Ab covalent immobilization, blocking with bovine serum albumin (BSA), E. coli incubation and finally labelling with CdS@ZIF-8@PEI-Ab tags. (b) Electrochemical detection of leached Cd(II) resulting from etching of CdS@ZIF-8@PEI-Ab tag. Adapted from ref. 78 with permission from Elsevier B.V., Copyright 2018. | |
A strategy combining AuNPs amplification with magneto–immunorecognition was developed for detection of Salmonella enterica subsp using a low cost screen-printed carbon electrode (SPCE) integrated with a permanent magnet to facilitate separation and detection of Salmonella in skimmed milk. The use of immunomagnetic beads with anti-Salmonella Ab were effective at capturing and concentrating the bacteria. A limit of detection of 143 cells per mL was reported, demonstrating potential for achieving high sensitivity and a shorter analysis time as compared to conventional methods.79
E. coli and Salmonella have also been detected using a AuNPs Surface Plasmon Resonance (SPR) assay developed for use in crude food samples.80 The sensor was fabricated by modifying a SPR chip with poly(carboxybetaine acrylamide) (pCBAA) brushes with a 20 nm brush thickness, followed by covalent binding of Ab onto their surface. Crude cucumber and hamburger extract containing bacteria were deposited on the surface followed by addition of a secondary biotinylated Ab and later streptavidin-coated spherical AuNPs. The system was able to detect down to 57 CFU mL−1 and 17 CFU mL−1 E. coli and 7.4 × 103 CFU mL−1 and 11.7 × 103 CFU mL−1 for Salmonella in cucumber and hamburger extracts within 80 min. In another study, AuNPs were decorated on the surface of magnetic NPs (MNPs) to enable both detection and separation of bacteria.32 MnFe2O4 NP cores were coated with a polyethylene imine layer (+charge) followed by AuNPs shell (−ve charge) seeding on the surface through electrostatic interactions. The Au MNPs were bound to Staphylococcus aureus specific Abs and the platform was used for separation and detection of the target bacteria. The method was able to detect 10 cells per mL (Fig. 9).
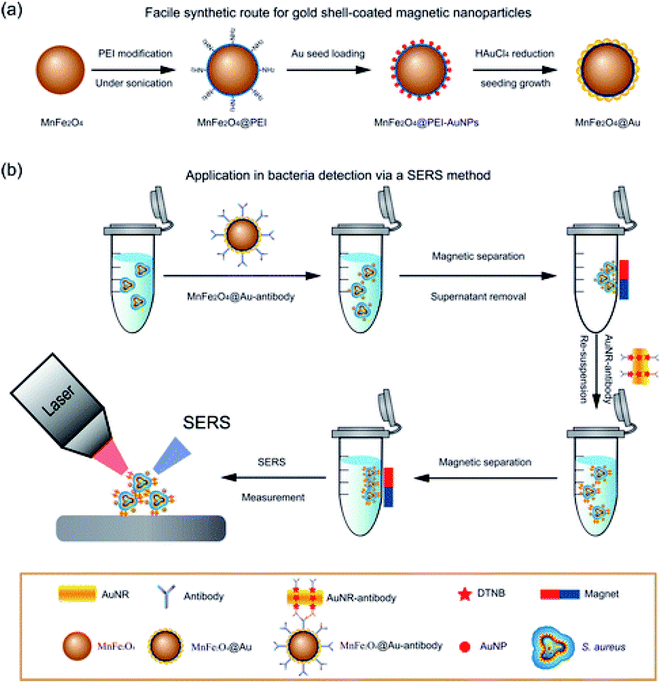 |
| Fig. 9 (a) Synthesis of MnFe2O4@Au core/shell NPSand (b) SERS method of Staphylococcus aureus detection. Adapted with permission from (ref. 32, Copyright (2016) American Chemical Society. | |
2.6 Heavy metals
The contamination of the environment with heavy metals leads to further contamination of food. Another source of heavy metal contamination is the contact of food with materials used in labels and packaging such as text/ink patterns, and images.81 Food processing and the use of processing aids, such as minerals used in the filtration of beverages, may also release trace amounts of heavy metals.82 The presence of trace amounts of heavy metals such as Hg2+ As3+and Pb2+ in food poses significant public health concerns. Conventional methods of analysis involve using Atomic Absorption/Emission Spectroscopy (AAS/AES)83 and Inductively Coupled Plasma Mass Spectroscopy (ICPMS).81,84 These methods provide low detection limits and high sensitivity; however, their high cost and limited availability limit their utilization to testing in centralized laboratories. Cost-effective methods that can be used in the field would increase field-testing enabling large scale monitoring of potential contamination. Nanomaterials provide opportunities for adsorption and pre-concentration of heavy metal. They have also been used as materials in electrochemical sensors, increasing the detection sensitivity of these devices for field analysis.85
Electrochemical sensors with anodic striping voltammetry (ASV) detection provide a simple, user-friendly method for detection of heavy metals contamination. High surface area carbon based nanomaterials such as graphene and CNTs were integrated with various recognition elements such as aptamers,86 antibodies and enzymes87,88 and the composites were used as materials for electrochemical sensors to detect heavy metals. A sensor for detection of Hg2+ was developed using single-walled CNTs functionalized with thiophenol groups (SWCNT-PhSH) over a Au electrode, with a LOD of 3.0 nM.88 Baghayeri M, 2018 used glutathione coated magnetic nanocomposite (GSH@Fe3O4) to detect Pb2+ and Cd2+ ions via anodic stripping voltammetry.89 The magnetic nanocomposite enabled pre-concentration and reduction of metal ions at a modified GCE. LODs of 0.182 μg L−1 and 0.172 μg L−1 were reported. A conjugate of graphene quantum dots (GQDs) and cysteamine-capped AuNPs enabled ultralow detection of Hg2+ and Cu2+.90 The GODs-AuNPs were deposited on a GCE and the carboxyl groups of the modified electrode composite interacted with Hg2+, enabling detection. The high affinity of AuNPs towards Hg2+ helped in concentrating the ions prior to anodic stripping voltammetry. LODs of 0.02 nM for Hg2+ and 0.05 nM for Cu2+ were achieved.
Optical sensors were also designed to detect heavy metals.91–93 The most used platform is based on DNA-functionalized AuNPs (DNA-AuNPs), used to detect a variety of heavy metals, including Hg2+.94 Hg2+ coordinates with thymidine (T) as T–Hg–T creating a purple color due to AuNPs aggregation. A LOD of 100 nM was achieved. Another example is a fluorescent sensor developed for detection of an array of metal ions (Hg2+, Cu2+, Fe3+).95 The method is based on synthesized luminescent nitrogen-doped carbon dots. The carbon dots were decorated with three different amino acids (L-glycine, L-lysine and L-serine) that induced various quenching responses to metal ions, with LODs in the 10 μM range.
Table 1 provides examples of recently developed nanomaterials-based (bio)sensors for detection of pathogens, toxins, allergens and antioxidants in food. The role on nanomaterials in each sensor design is described.
Table 1 Nanomaterials used in food (bio)sensors
Sensor type |
Analyte |
Sample |
Nanomaterial |
Function |
Ref. |
Electrochemical |
Antioxidants ((BHA), (BHT) and (TBHQ) |
Edible oils |
AuNPs electrodeposited on GCE |
Improved conductivity due to surface area enlargement and increase of active sites |
30 |
Antioxidants (ascorbic acid) |
Fruit juice |
Graphene nano ribbons (GNR) |
Enhanced surface area and electrochemical properties |
37 |
Mannitol, sucrose, lactose, glucose, and fructose |
Banana and bovine milk |
Graphene-Cu NPs |
Oxidation of carbohydrate |
42 |
Mannitol, sucrose, glucose, and fructose |
— |
CNTs-Cu NPs |
CNTS provided high surface area. Cu NPs enabled oxidation of carbohydrate |
43 |
Glucose, fructose and sucrose |
Soft drinks |
Cu NPs based ink |
Oxidation of carbohydrate |
44 |
Toxins (BPA) |
|
AuNPs loaded on MWCNT |
Enhanced surface area and conductivity |
27 |
Adulterants (melamine) |
Milk |
|
|
|
Toxins (BPA) |
Baby feeding bottles |
MWCNT |
Conductive and functionalizable layer |
25 |
|
Adulterants, Sudan I |
Tomato sauce |
-GNRs/GO |
GO provided a matrix for antibody immobilization. GNRs/GO enhanced the surface area thus enhanced the signal generation |
22 |
Chili sauce |
-Pd/Au core–shell nanocrystallines (Pd/Au CSNs) |
|
Chili powder |
-CdSe@CdS QDs |
Pd/Au CSNs provides catalytic activity and high surface area |
Electrochemical |
Adulterants (dopamine) |
In vivo rat brain |
TiO2/CeO2 NPs |
Enhanced sensitivity |
59 |
Residual pesticides (chlorpyrifos, fenthion and methyl parathion) |
Cabbage and spinach extract |
CuO nanostructure |
Surface area enhancement |
65 |
Residual pesticides (paraoxon) |
Soil and water |
PtNPs |
Surface area, conductivity and enzyme loading enhancement |
66 |
Pathogens (E. coli O157:H7) |
Milk |
Cd QDs encapsulated in ZIF-8 metal organic framework |
Electrooctochemical signal generation due to Cd etching to Cd(II) |
78 |
Pathogens (Salmonella) |
Skimmed milk |
AuNPs label |
Electrochemical signal generation |
79 |
Heavy metals (Hg2+) |
Water |
SWCNTs gold |
Provides a high surface area of thiophenol-modified SWCNTs gold electrode |
88 |
Heavy metals (Pb2+, Cd2+) |
Water |
GSH@Fe3O4 |
Preconcentration |
89 |
Heavy metals (Hg2+, Cu2+) |
— |
GODs-AuNPs |
Preconcentration |
90 |
Optical |
Antioxidants (ascorbic acid, gallic acid, vanillic acid, quercetin, caffeic acid, and epigallocatechin gallate) |
Tea and medicinal mushroom |
CeO2 NPs |
Catalytic activity |
31 |
Antioxidants (ascorbic acid) |
Orange and orange juice |
MnO2 nanosheets |
Catalytic activity |
50 |
Antioxidants (gallic acid) |
Green tea |
Au nanocage![[thin space (1/6-em)]](https://www.rsc.org/images/entities/char_2009.gif) |
Morphological change induces LSPR change upon seed-mediated growth of Ag |
48 |
Optical |
Mycotoxin (ochratoxin A) |
Milk |
CeO2 NPs |
Catalytic activity |
55 |
Adulterants (melamine) |
Milk powder |
AuNPs |
SPR optical properties |
24 |
Adulterants, Sudan I |
Tomato sauce and chili powder |
Au nanocolloid |
Visible colorimetric signal on nitrocellulose strip |
23 |
Adulterants (dopamine) |
Aqueous media and serum |
CeO2 NPs |
Colorimetric signal |
57 and 58 |
Veterinary antibiotics (kanamycin) |
Milk |
Au@AgNPs |
SERS activity |
62 |
Veterinary antibiotics (26 sulfonamides) |
Honey |
AuNPs |
|
63 |
Pathogens (E. coli and Salmonella sp.) |
Cucumber and hamburger extracts |
AuNPs |
SPR optical properties |
80 |
Pathogens (Staphylococcus aureus) |
— |
AuNR |
• SERS activity |
32 |
MnFe2O4@Au |
• Separation |
Heavy metals (Hg2+) |
— |
DNA–AuNPs |
Colorimetric response due to aggregation |
94 |
Heavy metals (Hg2+, Cu2+, Fe3+) |
— |
Nitrogen-doped carbon dots |
Fluorescent response |
95 |
3 Nanomaterials in biodegradable food packaging
Glass, steel, aluminum, paper and paper board, wood and plastic are examples of materials used for food packaging. Food packaging relies heavily on the use of non-degradable polymers mainly plastics such as polyethylene (PE), polypropylene (PP), polystyrene (PS) and polyethylene terephthalate (PET). These materials have good mechanical strength and thermal stability, and provide high quality protection to food. However, their increased usage and non-biodegradability pose significant environmental risks. Sustainable and environmentally friendly materials are required to replace non-degradable plastics and reach the United Nation sustainability development goal (SDG) to “Ensure sustainable consumption and production patterns”.50
Packaging is important to protect food items, preserve the nutritional value, enable proper handling and facilitate storage and transport through the supply chain. The food sector contributes to around 22% of the total greenhouse gas emissions in which packaging waste reaching landfills is part of the energy consumption and pollution.50 Adapting polices to reduce plastic usage and develop greener and more sustainable environmentally friendly alternatives to currently used plastics is needed. A solution to this problem is to use biodegradable materials and improve their properties (e.g. mechanical strength, gas barrier) to allow for their application in food packaging.96 This can be achieved by incorporation of nanomaterials (e.g. nano-fillers) in biodegradable polymers and form bio-nanocomposites that can provide enhanced mechanical strength and serve as a barrier for microorganisms, moisture and gases (O2 and CO2).97 Examples of biodegradable polymers are polysaccharides (e.g. starch, cellulose, chitosan), proteins, and bio-derived polymers conjugated with synthetic polymers (e.g. poly lactic acid (PLA), polyhydroxybutyrate (PHB)).98 The physical and chemical properties of the biopolymer/nanomaterial composites or films have been significantly different that those of the native biopolymers, and it was demonstrated that these properties can be tuned by the selection of the type, amount, chemical modification and fabrication procedure. Examples of biodegradable polymers that incorporate nanomaterials that have potential for applications in food packaging are summarized in this section, along with their properties and development status.
3.1 Starch
Starch is inexpensive, widely available, renewable and biodegradable, making it one of the most promising biopolymer for food packaging. However, starch in its native form has weak barrier properties, water sensitivity and brittleness.99 Recent research has demonstrated that incorporation of TiO2 NPs, graphene and poly(methyl methacrylate-co-acrylamide) NPs improves the mechanical, UV-protective and water barrier properties of starch.100–103 Goudarzi, V. et al. (2017) fabricated starch/TiO2 bio-nanocomposites with different content of TiO2 (1, 3 and 5 wt%).100 The resulting films showed enhanced hydrophobicity, thermal properties and reduction of water vapor permeability. The addition of TiO2 reduced the tensile strength and Young's modulus, increasing the elongation at break and tensile energy to break, and provided UV-protective properties, demonstrating potential as UV shielding materials. Ashori, A. et al. (2014) fabricated biodegradable blend films from chitosan and tapioca starch loaded with nano graphene.101 The addition of an optimum amount of 0.8 wt% nano graphene enhanced the tensile strength and thermal stability, and reduced water vapor permeability.101 In other works, synthetic poly vinyl alcohol (PVA) loaded with nano-sized poly(methyl methacrylate-co-acrylamide) (PMMA-co-AAm) particles was blended with natural corn starch to improve their water hydrophobicity.102 The film demonstrated a 70–400% enhancement of mechanical properties and water resistance. Moreover, the film showed good degradability of 45–65% after 165 days. Antimicrobial films were also fabricated by using high-amylose starch/PVA blend films with and TiO2 NPs.103 The use of up to 0.6% of TiO2 NPs resulted in a significant improvement in mechanical properties and an increase in tensile strength due to formation of hydrogen and C–O–Ti bonds. Additionally, the films showed antimicrobial activity that were attributed to the loading with TiO2 NPs.
3.2 Chitosan
Chitosan is a highly abundant, biocompatible, biodegradable and antimicrobial polysaccharide104 that has potential for food packaging applications. Nanomaterials can enhance antimicrobial activity and improve mechanical properties of chitosan. Chitosan/nanocomposite films can be prepared by solution casting, coating or layer-by-layer assembly. An antimicrobial bionanocomposite film was synthesized by solution casting using chitosan, ZnO NPs and neem essential oil. A concentration of 0.5% ZnO NPs resulted in improved mechanical properties e.g. tensile strength, film thickness and transparency, and provided gas barrier properties and reduced water solubility. The use of ZnO NPs increased the antimicrobial activity of the film towards E. coli.105 An active ZnO/chitosan nanocomposite coated on plasma-treated polyethylene film (PE), showed enhanced adhesion, increased antimicrobial properties and inhibition of Salmonella enterica, E. coli, and Staphylococcus aureus as compared to a pristine chitosan film.106 A nanocomposite film based on MgO NPs, chitosan and clove oil extract prepared by solution casting demonstrated enhanced mechanical property, film thickness and transparency, and reduced swelling, as well as enhanced antibacterial activity towards Staphylococcus aureus.107 Another study showed significant improvement of mechanical properties of chitosan by adding MgO NPs, which can be attributed to the interaction of hydroxyl and amine groups of chitosan with the MgO surface. The optimum properties were achieved at 5 (w/w)% MgO loading which improved the tensile stress by 86% and the elastic modulus by 38%. The film also displayed thermal stability, flame retardant properties, UV protection and moisture barrier properties.108 Addition of TiO2 NPs to polyvinyl alcohol (PVA)–chitosan film improved the mechanical and gas barrier properties and provided antibacterial activity against E. coli, S. enterica, L. monocytogenes, and S. aureus. The use of a high hydrostatic pressure (HHP) treatment to prepare the film increased the interaction between chitosan and PVA creating a more compact mechanically stable structure. The film was tested for NP migration in distilled water, acetic acid, ethanol and olive oil. Of these, only olive oil showed trace amounts of TiO2 (<4.20 × 10−3‰). The HHP treatment (200–400 MPa) is found to significantly decrease migration of NPs.109 Nanocrystalline cellulose or cellulose nanocrystals (CNC) is another common material known to reinforce and improve mechanical properties of chitosan. A 5% (w/w) amount of CNC dispersed homogeneously in chitosan showed a 26%, improvement in tensile strength and a 27% reduction in water permeability.110
3.3 Cellulose
Cellulose is the most abundant biopolymer in nature; it is produced inexpensively in a large scale from sustainable sources, and is biocompatible and biodegradable.111 Common cellulose does not have sufficient functional properties and cannot be easily processed for food packaging applications in its native form, and therefore has limited value and utility. A more recently developed form of cellulose is nanocrystalline cellulose or cellulose nanocrystals (CNC).112 A fully degradable CNC based nanocomposite film was fabricated using poly(3-hydroxybutyrate-co-3-hydroxyvalerate) (PHBV) and functionalized CNC methyl ester, CNC-me. CNC was esterified to increase hydrophobicity and enhance the dispersion in the hydrophobic PHBV polymer. The film demonstrated significant enhancement in mechanical properties, thermal stability, barrier and migration properties with increasing CMC-me concentrations up to a maximum 20% loading, due to improved interfacial adhesion and increased crystallinity. Moreover, CNC migration was tested in non-polar isooctane and polar (10% ethanol) simulants and the levels were found below the limits imposed for food packaging materials.113 Multifunctional antimicrobial and antioxidant packaging films were developed by incorporating AgNPs (5.77%), grapes seeds extract (GSE) and 2,6,6-tetramethylpiperidine-1-oxyl (TEMPO)-oxidized nano-cellulose TNC (Fig. 10). The films displayed improved mechanical properties, lower water vapor and oxygen permeability and good antimicrobial activity against E. coli and S. aureus as compared to the TNC films in absence of AgNPs. The films could be used as packaging materials to extend food storage.114
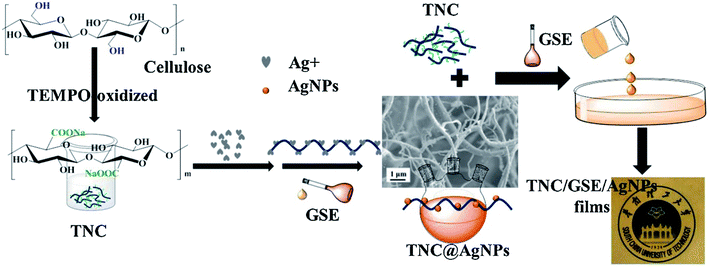 |
| Fig. 10 Preparation of multifunctional TNC/GSE/AgNPs films with antimicrobial and antioxidant activity. Adapted from ref. 114 with permission from Elsevier Ltd, Copyright 2018. | |
Nano-biocomposites were also prepared using CNC and surfactant modified-CNC embedded in a thermoplastic polymer derived from natural sources, polylactic acid (PLA). The surfactant provided a suitable dispersing medium for the CNC, improving homogeneity of the film. The overall performance of the optimized film (1 wt% of surfactant-CNC) showed a 34% reduction in water permeability, and improved oxygen barrier properties when 1 wt% and 5 wt% of CNC and surfactant-CNC were used.115
3.4 Proteins
Proteins added to plasticizers such as glycerol, polyethylene glycol, and sorbitol can be used to produce biodegradable films but these lack strong mechanical properties and water hydrophobicity.116,117 Integrating nanomaterials such as CNC could enhance these properties and render these films suitable for packaging. A gelatin nanocomposite film based on CNC generated from acid hydrolysis of fibrous bacterial cellulose obtained from Gluconacetobacter xylinus demonstrated improved mechanical and thermal properties and reduced moisture affinity of gelatin.118 Whey protein isolates (WPI) nanocomposite embedded with zein NPs (ZNP) fabricated by solution casting provided a packaging material with reduced hydrophilicity and improved moisture barrier. To enhance the dispersion, the ZNP particles were coated with sodium caseinate that has both hydrophobic and hydrophilic ends, creating an efficient interface between the hydrophobic ZNP and hydrophilic WPI matrix, and improved homogeneity of the NP mixture.119 WPI was also used to prepare a nano-clay type composite with improved transparency and antimicrobial properties against Listeria monocytogenes It was found that incorporation of small amounts of nano-clays, e.g. Cloisite Na+, Cloisite 20A, and Cloisite 30B, into WPI films change the film properties, especially the mechanical properties and water vapor barrier.120 ZnO NPs integrated with soybean protein isolate (SPI) produced films showing a 16% increase in microbial activity and a 234% increase in tensile strength.121
3.5 Synthetic polymers
Svagan, Anna J., et al. (2012) reinforced PLA with montmorillonite clay (10–1000 nm) and chitosan to form a polymer layer with improved oxygen barrier properties. The use of PLA reduced the oxygen permeability coefficient by 99% and 96%, respectively at 20 and 50% relative humidity (RH) significantly improving the gas barrier properties for packaging applications.122 In other works, bacterial polyester poly(3-hydroxybutyrate-co-3-hydroxyvalerate) (PHBV) was integrated with ZnO NPs to prepare a biodegradable nanocomposite via casting method.123 The filler interacted with the polymer via hydrogen bonding and showed high dispersion uniformity. This resulted in enhanced thermal stability and mechanical properties e.g. stiffness, strength, toughness, and glass transition temperature. The nanocomposite showed decreased water uptake and gas vapor permeability compared to the bare polymer. (Fig. 11) shows the effect of ZnO NPs content (4.0 wt%) on water uptake and Young's modulus. The film demonstrated antimicrobial activity against E. coli and Staphylococcus aureus with a weaker effect on the latter. Table 2 provides examples of recently developed nanomaterials proposed for use in biodegradable packaging. The type and role of nanomaterials and the packaging application are described.
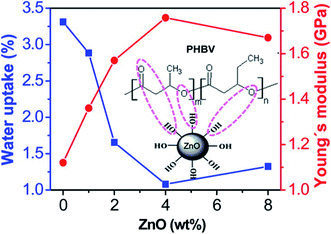 |
| Fig. 11 The effect of ZnO NPs content on water uptake and Young's modulus of a ZnO–PHBV nanocomposite. Reprinted with permission from ref. 123. Copyright (2014) American Chemical Society. | |
Table 2 Nanomaterials in biodegradable food packaging
Polymer |
Nanomaterial |
Type |
Nanomaterial effect |
Ref. |
Starch |
TiO2 |
Starch |
• Improved UV-protection |
100 |
• Improved thermal properties |
• Improved hydrophobicity and reduction of water vapor permeability |
Nano-graphene |
Chitosan–tapioca starch |
• Improved thermal properties |
101 |
• Improved mechanical properties |
• Moderate reduction of water vapor permeability |
TiO2 |
Amylose starch/PVA |
• Improved mechanical properties and water resistance |
103 |
• Antibacterial activity |
Nano-sized poly(methyl methacrylate-co-acrylamide) (PMMA-co-AAm) particles |
Corn starch–PVA |
• Improved mechanical properties and water resistance |
102 |
• Improved degradability up to 45–65% after 165 days |
Chitosan |
ZnO NPs |
Chitosan |
• Improved mechanical properties (tensile strength, film thickness and transparency, barrier properties |
105 |
• Improved antimicrobial activity |
Nano ZnO |
Chitosan coated on PE film |
• Improved antimicrobial activity |
106 |
• Enhanced solubility |
MgO |
Chitosan |
• Improved mechanical properties (tensile strength, film thickness and transparency, barrier properties |
107 |
• Improved antimicrobial activity |
MgO |
Chitosan |
• Improved mechanical properties |
108 |
• Thermal stability and flame retardant properties |
• UV protection |
• Moisture barrier properties |
TiO2 |
Chitosan/PVA |
• Improved mechanical properties (tensile strength, film thickness and transparency, barrier properties |
109 |
• Improved antimicrobial activity |
• No particles migration of the films prepared by high hydrostatic pressure (HHP) treatment |
CNC |
Chitosan |
• Improved mechanical properties (tensile strength and barrier properties) |
110 |
Cellulose |
AgNPs and CNC |
Cellulose |
• Improved mechanical properties |
114 |
• Antibacterial |
• Antioxidant |
CNC |
Cellulose nanocrystals |
• Enhancement in mechanical properties, thermal stability, and barrier migration |
113 |
Proteins |
Bacterial based CNC |
Gelatin |
• Enhanced mechanical properties |
118 |
• Reduced water affinity |
• Edible, biodegradable |
ZnO NPs |
Soybean protein |
• Increase in microbial activity by 16% |
121 |
• Enhance in tensile strength by 234% |
• Improve the thermal and mechanical properties |
Zein NPs |
Whey protein isolate (WPI) |
• A significant improvement of mechanical properties and water vapor barrier |
119 |
Nano clay |
WPI |
• Mechanical properties of tensile strength and water vapor barrier were improved except the case of Cloisite 30B |
120 |
• WPI/Cloisite 30B exhibited antimicrobial activity against Gram-positive bacteria, Listeria monocytogenes unlike other nanoclays |
Synthetic polymers |
ZnO NPs |
Bacterial polyester poly(3-hydroxybutyrate-co-3-hydroxyvalerate) (PHBV) |
• To increase in microbial activity by 16% and to enhance in tensile strength by 234% and to improve the thermal and mechanical properties |
123 |
CNC |
PLA |
• Reduction in water permeability. However, oxygen barrier properties were improved |
115 |
Nanoclay |
Polylactide (PLA) |
• Significant improvement of oxygen barrier |
122 |
4 Nanomaterials in active and functional packaging
Yearly, about one third of the food produced around the world (1.3 billion tons) is wasted due to spoilage.50 Food contamination and spoilage affect human health and has large economic impact, and raising medical care expenses.124 New technology to reduce waste and increase food safety is needed. This include strategies for real time quality monitoring and the development of active and functional materials to increase the product shelf-life through packaging. Traditional packaging materials are made of inactive materials that prevent moisture and O2 penetration, made primarily from non-degradable conventional plastics like PE and PET. With the development of new materials, opportunities exist to create active packaging that provide functional properties to extend shelf-life and improve safety.125 These include oxygen scavengers to minimize fat oxidation,126 moisture absorbers to reduce microbial growth,127 ethylene scavengers to reduce fruits and vegetables ripening,128 carbon dioxide emitters to inhibit spoilage by microbial action. CO2 could also be used in tandem with O2 scavengers to replace the absorbed O2bib129.129 Active packaging could also include emitting sachets or coatings that release antimicrobial agents, antioxidants, flavors and preservatives to maintain or improve food quality. These can be included within conventional non-degradable packaging, and more recently are being used in conjunction with degradable biocompatible substitutes. In addition to adding functional properties, efforts are underway to develop intelligent packaging that, in addition to food preservation, would monitor food and communicate with suppliers or the consumer the quality of packaged food at any point of the supply chain, or at the time of use. Intelligent packaging includes sensors, indicators and data carriers,130 that contain and provide information about the quality characteristics of the packaged product. Main types of chemical and biological sensors of interest in food analysis, active and smart packaging have been discussed in Section 2 of this review and several reviews on sensing technologies can be found in literature.131 Nanomaterials are used to enhance the performance of packaging by offering added functionality such as antimicrobial activity, UV protection, oxidation prevention, etc. They can also be used as a signal generator and sensing element in smart packaging, for example as freshness indicators (Fig. 12). This section provides an overview of the commonly used indicators and materials and describes their development status towards active and intelligent packaging.
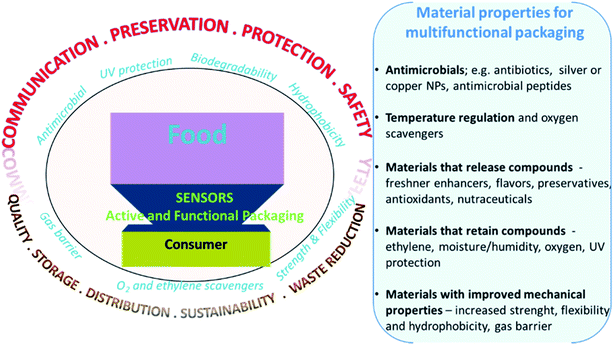 |
| Fig. 12 Illustration of the main properties and applications of active and functional sensors and materials for packaging applications. | |
4.1 Time–temperatures indicators (TTI)
The most common commercially available indicators are time–temperature indicators (TTI) that record the thermal history of the food product during the entire supply chain.132 The device provides a visual signal (e.g. irreversible color change) indicating changes in the storage conditions of the product (time and temperature). The functional principles of these probes rely on chemical reactions such as heat stimulated polymerization, pH, light or photochromic induced reactions,133 or physical changes altering diffusion of colored materials within a porous matrix,134 or change due to the plasmon resonance of metallic NPs.135 Zhang, Chao et al. (2013) created a plasmonic TTI to document the temperature history and indicate the deterioration process of perishable products employing kinetically programmable plasmonic nanoclusters of Ag on AuNPs.135 The design principle is based on assessment of the growth rate of bacteria as a function of temperature, quantified by measuring changes in the chronochromic behavior during the heteroepitaxial growth of Ag on Au nano rods. The growth of Ag on Au nanorods causes a red shift of the extinction band with a concomitant color change from red to green which was correlated with the magnitude of microbial growth. To produce a chemical tag-like indicator the colloidal solution was solidified with agar (Fig. 13). Wang, Yi-Cheng et al. (2015) reported a chemical TTI indicator that uses spherical AuNPs prepared by reducing tetrachloroaurate in gelatin.136 It was found that the indicator changed its color from pink to red within 90 days of storage and that the color change was dependent on the temperature.
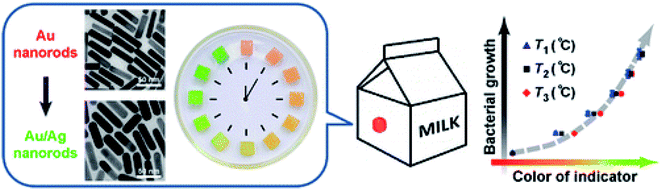 |
| Fig. 13 Time–temperature indicator based on silver growth on gold nanorods creating a change in color related to microbial growth which is governed by time and temperature. The clock illustration shows Ag/Au nanorods-hydrogel cubes arranged in a Petri dish indicating the color change over time. Reprinted with permission from ref. 135. Copyright (2013) American Chemical Society. | |
4.2 Antimicrobial active packaging
Antimicrobial packaging are developed with the aim to preserve food items and prolong shelf-life by inhibiting microbial growth. This is achieved by incorporation of an active agent or deposition of a coating layer onto/within the packaging material.137 These agents include organic acids, enzymes, bacteriocins, fungicides, polymers (e.g. chitosan), and natural extracts. Antimicrobial nanomaterials such as AgNPs have attracted significant attention for this particular type of packing due to their well-known antimicrobial activity. Gu, Rong, et al. (2019) fabricated AgNPs-based antimicrobial biodegradable film with <10% AgNPs leakage.138 The film was prepared by using hyperbranched polyamide-amine (HPAMAM) as a template, reducing agent, and stabilizer to synthesize and prevent leaching of AgNPs. The nanocomposite was embedded into an oxidized cellulosic film via amino-aldehyde bond formation (Fig. 14A). The film showed effective antimicrobial activity towards E. coli and Staphylococcus aureus and an extended shelf life when this was used as packaging for cherry tomatoes (Fig. 14B). While the antimicrobial activity of AgNPs has been widely reported, the toxicity of AgNPs, and their potential leaching from packaging into food, should be tested before their large scale implementation in packaging.
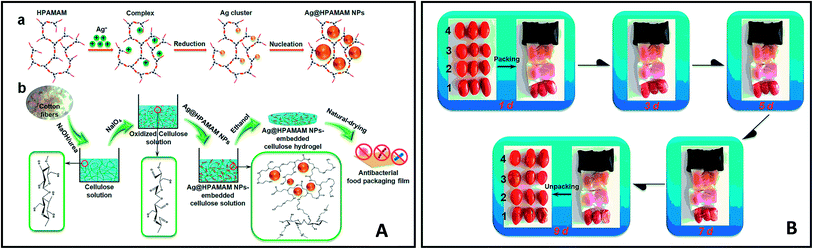 |
| Fig. 14 (A) Synthesis mechanism of AgNPs-based cellulosic antimicrobial film. The film was prepared by using hyperbranched polyamide-amine (HPAMAM) as a template, reducing agent, and stabilizer producing composite Ag@HPAMAM NPs. Oxidized cellulose film was produced by dissolving cotton with alkali hydroxide/urea solution followed by oxidation of hydroxyl groups at C-2 and C-3 of the glucose residues to aldehyde groups by NaIO4. The composite then was embedded into oxidized cellulosic film via amino-aldehyde bond and left to dry. (B) Cherry tomatoes were stores in controlled condition of 25 °C, 75% relative humidity up to 9 days. Tomatoes were wrapped with 1-commercial PE film 2-cellulose film 3-oxidized cellulose film 4-Ag@HPAMAM NPs-embedded cellulose film. The appearance of tomatoes was observed. Reprinted with permission from Copyright (2020) American Chemical Society. | |
Cellulose nanofibrils (CNF) were used as emulsifier for soybean oil to develop an antimicrobial composite film. The film exhibited flexibility, optical transparency, thermal stability and biodegradable properties. A nutraceutical compound, curcumin was encapsulated within the nanocomposite to provide the film with antioxidant (radical scavenging) properties and antibacterial activity against E. coli.139 An antimicrobial coating for meat was developed based on low- and medium-molecular weight chitosan integrated with nano sized commercially available organic acids (benzoic acid and sorbic acid).140 The organic acid coatings showed a significant increase of antimicrobial activity as compared to their non-nano counterparts. In other works, a nanocomposite film of TiO2 nano-powder incorporated in chitosan141 showed improved mechanical properties and a significant increase in antimicrobial activity against E. coli, Staphylococcus aureus, Candida albicans, and Aspergillus niger. The film showed extended product shelf-life when used as packaging for red grapes (Fig. 15).
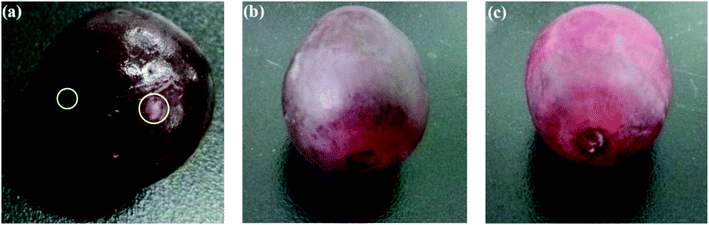 |
| Fig. 15 Extended shelf-life of red grape packed at 37 °C for 6 days in (a) plastic wrap; (b) chitosan film; (c) chitosan–TiO2 film. Adapted from ref. 141 with permission from Elsevier Ltd, Copyright 2017. | |
Flexible nano-ZnO–chitosan pouches with antimicrobial activity were prepared by direct synthesis of ZnO NPs into chitosan. The antimicrobial efficacy was linearly related to the amount of ZnO NPs. The pouches were used as a packaging material for row meat and the results showed increased antimicrobial action and an extended shelf-life when compared to conventional PE materials.142 An active nanocomposite packaging fabricated by coating commercial packaging with layers of CNC and TiO2 NPs mixed with wheat gluten and glycerol processed by casting/evaporation143 showed antimicrobial activity against Saccharomyces cerevisiae, E. coli and Staphylococcus aureus, with 98.5% reduction of microbial growth for three layers coated paper after 2 h exposure to UV illumination as compared to TiO2-free coated paper. The CNC nanocrystals and the TiO2 NPs improved the mechanical and water barrier properties of gluten, also providing good antimicrobial activity against Gram positive and Gram negative bacteria and yeast.
4.3 Active packaging incorporating gas scavengers
The development of gas scavengers and gas absorber materials is important for creating packaging that provides an extended shelf-life, maintains the quality and freshness of food products and contributes to the reduction of food waste. Most research is conducted to develop scavengers to adsorb ethylene and oxygen that permeates into packaging, which can induce degradation and result in spoilage.
Ethylene (CH2CH2) is emitted by many fruit and vegetables. When this is released in excessive amounts, it increases ripening and deterioration rate of the products, reducing their shelf-life. Packages containing ethylene scavengers are used to limit the rate of ethylene accumulation and maintain freshness.144 Ethylene scavengers consist of materials that can oxidize, decompose or adsorb ethylene through chemical or physical processes. Common materials include natural clays, catalytic oxidizers like potassium permanganate and silver nitrate, sorbents like propylene glycol, electron deficient dienes or trienes having electron-withdrawing substitutes like tetrazines and adsorbents like activated carbons, zeolites or silica gel.144 Photocatalytic oxidation of ethylene can also be achieved with photocatalytic materials like TiO2, which can generate reactive oxygen species (ROS) upon UV illumination resulting in species that can oxidize ethylene.145 When TiO2 is activated by UV light, the electrons on the surface move from the valence band to the conductance band creating an electron pair hole (h+). Water or hydroxide ions (OH−) adsorbed on the TiO2 surface hydroxyl radicals (OH˙), undergo reduction to produce superoxide ions (O2−). These highly active radicals of O2− and OH˙ oxidize ethylene producing CO2 and H2O. Nanoscale photocatalysts show enhanced photocatalytic activity and can also be incorporated in conventional plastic or biodegradable packaging materials. A chitosan film containing TiO2 NPs demonstrated both ethylene scavenging activity and antimicrobial activity. The film contained homogenously dispersed TiO2 NPs with enhanced mechanical properties; the enhancement was due to formation of hydrogen bonding between O–Ti–O and chitosan. PAN@TiO2 nanofibers were prepared by electrospinning of polyacrylonitrile (PAN) and TiO2 deposition.146 The nanofibers showed photocatalytic ethylene degradation under low-intensity UV-radiation. As an example of application, the fibers were incorporated into a polypropylene (PP) film and the film was used to cover tomatoes during storage. The results showed effective ethylene degradation and slower softening of the fruit as compared to the PP film only. In another study, TiO2 NPs (1, 5 and 10 wt%) were coated on the surface of PAN nanofibers to delay the softening of bananas during storage. The fibers containing 5% wt TiO2 exhibited the best uniformity and the highest photocatalytic activity for the degradation of ethylene than the PP film without TiO2.147 The bananas were covered with a layer of TiO2 nanofiber film and a layer of PP and the results were compared to the PP film alone.148 Mixed (TiO2/SiO2) materials under UV light irradiation also showed ethylene degradation capabilities and extended tomato shelf-life.149
O2 present in food packaging due to permeation or failure in the packaging process can degrade food due to rapid oxidation of fats or vitamins present in food that can result in the growth of microorganisms. Addition of O2 scavengers to remove O2 and create an O2-free environment is a common practice to reduce spoilage and increase shelf-life.150 Commonly known O2 scavengers are used in the form of sachets or films containing materials such as: metallic (e.g. iron-based), organic or natural reducing agents like ascorbic acid, natural O2 scavengers like α-tocopherol, enzyme catalysts or photosensitive dyes impregnated onto polymeric coatings. Polypropylene nanocomposites containing iron NPs embedded in polymeric films have shown better performance than microsized iron in reducing O2 and water vapor permeability, showing a 77% increase in scavenging capacity.151 Similar characteristics were reported with nanoscale iron particles dispersed in a silicone matrix,152 which showed a 10 times higher adsorption capacity than commercial iron-based scavengers. In other studies, O2 scavenging films were fabricated from electrospun poly(3-hydroxybutyrate) (PHB) fibers containing palladium NPs (PdNPs). Two surfactants, hexadecyltrimethylammonium bromide (CTAB) and tetraethyl orthosilicate (TEOS) were used to enhance dispersion in PHB. Better dispersion and the best O2 scavenging properties were obtained with CTAB-stabilized PHB.153
4.4 Smart and intelligent packaging
A further extension to active packaging is to add the ability to provide information on the freshness status of food, and develop so called smart packaging. This can be achieved by sensing technologies or smart labels that can measure changes in the environmental conditions inside packaging. Meat and seafood deterioration could change the pH inside packaging due to the evolution of volatile organic amines,154,155 biogenic amines156,157 and microbial growth. For fruit ripening, an excessive amount of ethylene is secreted.149 These parameters can be used as degradation markers to assess changes in the quality and freshness of packaged food. A modified environment inside the package can also be monitored by measuring the O2/CO2, microbial growth or moisture content, all of which indicate changes in freshness status or conditions leading to degradation. Therefore developing sensors and labels to assess changes in the pH, O2, CO2, RH, CH2CH2 and the presence of degradation compounds will enable technologies that can provide direct information to the consumer, farmer or retailer regarding the quality of food.
Most currently developed sensors and labels are based on organic dyes designed to measure pH changes, and only few examples demonstrate uses of nanotechnology for such applications. Ammonium molybdate was adsorbed in the nanopores of zeolite158 to prepare zeolite-molybdate tablets used to scavenge and measure ethylene in avocados packages. The tablet undergoes a color change from yellow to blue within 10 days storage due to reduction of Mo(VI) to Mo(V).159 In another work, a reusable O2 sensitive plastic was synthesized by incorporation of a redox dye, methylene blue (MB), an electron donor, DL-threitol coated on TiO2, in low density polyethylene (LDPE) (Fig. 16). The photobleached colorless form of MB, the leuco-methylene blue (BMB) persists in absence of O2, but it is re-oxidized to the blue BM in about 2.5 days under ambient conditions, with a rate of recovery linearly dependent on the O2 level.15,160
Table 3 provides examples of nanomaterials used in smart packaging highlighting the nanomaterial function, the packaging used and food tested.
Table 3 Nanomaterials used in active and smart packaging
Nanomaterial |
Polymer/package material |
Nanomaterial function |
Food tested |
Ref. |
Core–shell Au/Ag nanorods |
Agar hydrogel |
Color change_TTI |
|
135 |
AuNPs |
Gelatin |
Color change-TTI |
|
136 |
AgNPs |
Cellulose films with amino-terminated hyperbranched polyamic |
1-Antibacterial |
Cherry tomatoes |
138 |
2-Increased up to 30% radical scavenging activity) |
Cellulose nanofibril |
Cellulose nanofibril–oil composite films |
Emulsifier |
|
139 |
The encapsulated oil enhanced antioxidant activity |
Nano-sized benzoic- and sorbic-acid solubilisate |
Chitosan |
Preservative (antimicrobial activity) |
|
140 |
TiO2 |
Chitosan |
Preservative (antimicrobial activity) |
Grapes |
141 |
Nano ZnO |
Chitosan |
Antimicrobial |
Meat |
142 |
CNC, and TiO2 NPs |
Wheat gluten |
Enhancing mechanical properties |
|
143 |
Antibacterial activity |
TiO2 NPs |
Chitosan |
Ethylene scavenging and antimicrobial |
|
161 |
TiO2 NPs |
Polyacrylonitrile (PAN) |
Ethylene scavenging |
Tomatoes |
146 |
TiO2 NPs |
Polyacrylonitrile (PAN) |
Photocatalytic ethylene scavenging |
Banana |
147 |
Palladium nanoparticles (PdNPs) |
Poly(3-hydroxybutyrate) (PHB) |
Oxygen scavenging |
|
153 |
Fe particles |
Silicone |
Oxygen scavenging |
|
152 |
Nanoporous-zeolite (molybdate) |
|
Ethylene detection by confined molybdate in the nanopores |
Avocados |
158 |
TiO2 |
LDPE |
O2 detection |
|
15 |
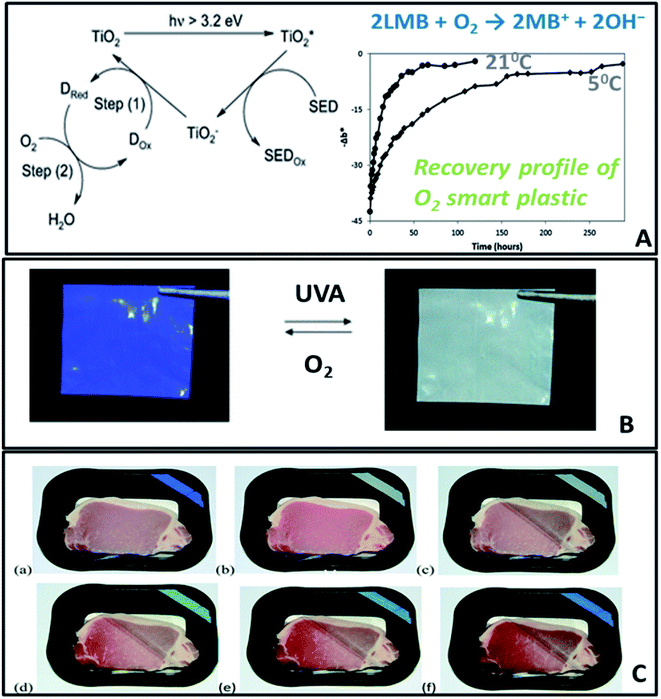 |
| Fig. 16 (A) Mechanism of a UVA-activated O2 indicating plastic, comprising a semiconductor TiO2 photocatalyst, a redox dye (Dox, such as methylene blue) and a sacrificial electron donor (SED, such as DL-threitol). (B) Photographs of a section of extruded O2-indicator film (a) before photoactivation and (b) after 90s exposure to UVA. (C) Photographs of pork, packaged within an O2 smart plastic film, heat sealed to the inside of a PET packaging lid. The package was sealed in the absence of oxygen (a) and the indicator activated (b). The packaging lid was then broken and partly lifted back, and photographed immediately (c), and after 1 day (d), 2 days (e) and 4 days (f) in a fridge at 5 °C. Adapted from ref. 160 with permission from The Royal Society of Chemistry, 2012. | |
5 Safety considerations
It is increasingly clear that nanotechnology offers a wide spectrum of benefits and can be a game changer for the food industry. However, as new materials are developed and new and unique properties are discovered a better understanding of the health and environmental impact of these materials throughout the food chain is needed before their full implementation into applications in the food sector and the consumer market. The use of nanomaterials through the consumption of nano food, nanosensing or through packaging incorporating nano-products could lead to migration of nanomaterials and subsequent human exposure through inhalation, skin penetration, and ingestion. Routes of possible exposure to humans include: (i) leaching of nanocomponents from packaging or sensing elements into the food, (ii) ingestion from intake of nano-food and (iii) disposal of packaging, nanosensors and nanofood in landfills with further release into the environment, air, water and soil (Fig. 17).162
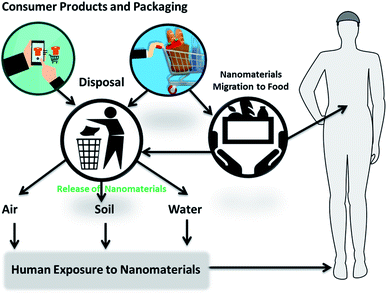 |
| Fig. 17 Potential routes of nanomaterials exposure from food and consumer goods into the environment and humans. | |
Because of their small size, high surface area and increased reactivity, nanomaterials pose unique risks and may cause adverse effects on human health and the environment.163 Upon use, nanomaterials may undergo physicochemical transformations in the local environment (pH, ionic strength, microbial environment, etc.) further changing their fate and transport behavior. While research is growing, the potential implications related to the use of nanomaterials in the food industry are not fully understood and require further evaluation. Furthermore, current regulation on the use of nanomaterials in food industries is also limited.
Migration of nanomaterials from the packaging or the sensing element inside the packaging is one area of potential concern. Garcia, et al. 2018 reviewed applications and migration of metal oxides such as titanium dioxide (TiO2), zinc oxide (ZnO), aluminum oxide (AlOx) and silicon dioxide/silica (SiO2) used in food packaging to improve mechanical, antimicrobial, light-blocking and gas barrier properties of polymers164 and concluded that, based on existing literature, only a very small amount of nanomaterials migrate from packaging into food. They also suggest that the risk of migration could be lowered by applying an additional barrier between the food and the nanocomposite. Lin, Qin-Bao, et al. (2014) studied the migration and dissolution of TiO2 from nano-TiO2-PE composite films used in food packaging.165 The films were immersed in food simulants of either 3% (w/v) aqueous acetic acid or 50% (v/v) aqueous ethanol for several hours at different temperatures and it was found that TiO2 migration was increased with time. A higher migration rate was observed in 3% (w/v) aqueous acetic acid than in 50% (v/v) ethanol, suggesting that migration might occur via dissolution from the solid film into the liquid phase. However, migration of these materials in real samples has not been studied. Zixuan, Lian et al. (2016) investigated the migration of nano-TiO2 from PVA–chitosan nanocomposite films in food simulants such as distilled water, acetic acid, ethanol and olive oil. The release of nano-TiO2 was only detected in trace amounts of <4.20 × 10−3‰ in olive oil.109 In another study, migration of poly(3-hydroxybutyrate)/CNC nanocrystal films was studied in food simulants of ethanol 10% (v/v) and isooctane under standardized conditions based on the Commission Regulation EU No 10/2011 (ref. 166) and the results concluded that the overall migration is within standard regulatory limits as per the EU legislation.
Díez-Pascual, Ana M and Diez-Vicente, Angel L (2014) studied the migration of ZnO NPs from a ZnO NPs-polyester poly(3-hydroxybutyrate-co-3-hydroxyvalerate) (PHBV) nanocomposite in polar and non-polar stimuli (isooctane and 10% (v/v) ethanol). The migration was found negligible and below the limits allowed by current legislation for food packaging.123 The release of nanoclay (organo-modified montmorillonite (O-MMT)) and surfactant from nanoclay fabricated using two polymers, polypropylene (PP) and polyamide 6 (PA6) was assessed in ethanol and at 70 °C. The study found that more nano-clay particles were released from the PP-based (0.15 mg L−1) than from the PA6 nano-clay (0.10 mg L−1), a finding that was explained by the lack of interaction between the nano-clay and PP. Meanwhile, the surfactant release in ethanol was significant: 3.5 mg L−1 from the PP–clay and 16.2 mg L−1 from the PA6, possibly due to exfoliation, indicating potential risks from migration of surfactants or additives used to create nanocomposites.162 Quadros, Marina E., et al. (2013) assessed the transfer of AgNPs from nanotech-based consumer products for children including breast milk storage bags, fabrics, cleaning products and sippy cups.167 The release was assessed in water, orange juice, milk formula, synthetic saliva, sweat, and urine. The highest amount of silver released was observed in sweat and urine, while the lowest amount was in water (<1.5%). Silver bioavailability was mainly in ionic form and exhibited low release (<1 mg Ag kg−1 product) for breast milk storage bags and sippy cups.
Since nanotechnology in the food sector is at an early stage, more research is required to correlate the safety of nanomaterials to factors such as size, shape, surface charge and surface area, purity, stability in different environments, duration of exposure, concentration, state of agglomeration, accumulation, metabolism, biotransformation, and the media in which nanomaterials are exposed.163,168,169 Studies to further understand risks of this technology should be conducted in more complex environments and migration of nanomaterials should be studied in realistic environments (pH, humidity, temperature) and with food products rather than simulants. These studies should provide further guidance on the design and safe implementation of nanomaterials into packaging and food. One other critical issue is the disposal of nano-based packaging and nano-foods and the potential for release of nanomaterials into air, water, soil, and further, to plans, organisms and humans. Currently, there is limited knowledge on the transformation and changes in the physicochemical properties, and the life-cycle assessment (LCA) of nanomaterials over the entire food supply chain. Such studies targeting specific foods could provide guidance to materials, food and environmental scientists and help promote more sustainable and environmental sound development of nanomaterials for the food industry.
6 Regulatory aspects and consumer perception
Several nanotechnology initiatives have been initiated by regulatory agencies worldwide to define safety of nanomaterials, and provide recommendations and future guidance concerning their application in commercial products, such as cosmetics, food ingredients and food contact substances (e.g. materials used in manufacturing, packaging, transportation, or holding food), animal food, and drug products.117 The European Food Safety Authority (EFSA) Scientific Committee released a scientific opinion on nanoscience and highlighted potential risks on food and feed,170 followed by a guidance document highlighting recommendations for industry using engineered nanomaterials (ENMs).171 The guideline discussed (1) the requirement for providing a physico-chemical characterization ENMs e.g. food additives, enzymes, flavorings, food contact materials, novel foods, feed additives and pesticides and (2) testing methodologies for hazard identification of ENMs (e.g. in vitro genotoxicity, absorption, distribution, metabolism and excretion and repeated-dose 90-day oral toxicity studies in rodents). The EFSA Panel on Contaminants in the Food Chain (CONTAM) published a statement about the presence of microplastics and nanoplastics in food, especially seafood172 stressing the lack of toxicity and toxicokinetic data for microplastics and nanoplastics and their effects on human health. Research is needed to develop new standard methodologies for analysis of microplastic and nanoplastic in food and their toxicity evaluation.
Nanotechnology applications in the agricultural, feed and food sector and the regulatory status in EU and non-EU countries has been published in an inventory173 summarizing scientific literature, adhoc literature, company websites and questionnaires. The report identified AgNPs, TiO2 NPs, and nano-encapsulates as the major nano-based materials used in this sector, with main applications as food additives and food contact materials. Several growing areas have been identified with potential for future applications that include the use of nanotechnology as feed additives, biocides, pesticides and food packaging. Limited records of toxicity assessment in agriculture, feed and the food sector have been found. The overall legislation regarding the use of these materials is limited but efforts to define the status of nanotechnology in the agro/feed/food sector continue as evidenced by recent reports.174,175
Society acceptance and willingness to pay (WTP) for nano-food or nano-packaging are other important issues. Bieberstein A. et al.176 evaluated the societal perception towards nano-food and nano-packaging in Germany and France and found that many consumers are reluctant to accept products incorporating nanotechnologies in their food. The study also highlighted the importance of familiarizing consumers with the concept of nanotechnology. Labeling requirements of nanotechnology products in the food sector is another open topic. In the US, the Food and Drug Administration (FDA) supports innovation and promotes the safe use of nanotechnology;125 however, there are no current labeling requirements for “nano” and current regulation states that industry remains the responsible party to ensure that products meet safety standards. In Europe, the European Parliament and the Council have required through Regulation 1169/2011 that foods containing engineered nanomaterials ingredients be labeled.177 Brown & Kuzma (2013) have studied the public attitude towards labeling of food nanotechnologies in the USA.178 Through analysis of focused groups in six US locations, it was found that the majority of participants support the idea of nanotechnology labels but indicated that additional information about nanotechnology should be included. A study assessing consumer attitude towards nanotechnology found that consumers are more likely to accept nanotechnology-based packaging that provide unique benefits to food, rather than integrating nanotechnologies directly into agro-food products.179 Overall, these studies highlight the need to develop transparent regulation and inform consumers about the benefits and risks of nanotechnology.
7 Conclusion
With increased awareness of food safety, nutrition and health, there exists a great demand for new technologies to improve the quality, quantity and safety of food products. This review described the use of nanomaterials in the food sector with focus on sensing technologies and packaging. An overview of the benefits and safety risks, along with the challenges in applying nanotechnologies to food, and a regulatory and consumer perspective were provided.
From the current state of the art, it is clear that nanomaterials have tremendous promise in the food industries, and many interesting uses remain to be explored. The beneficial uses derive from their physicochemical properties, characteristic of their nanoscale, enabling them to be used as antimicrobials, oxygen scavengers, and water repellents, or as reinforcing materials providing gas barriers and increased mechanical properties. Currently investigated materials are metal and metal oxides like TiO2, CeO2, SiO2, ZnO, Au and AgNPs, carbon-based nanomaterials like carbon nanotubes and graphene, and nano cellulose. Nanosized products such as nano-liposomes or nano-capsules carrying active nutraceuticals (e.g. nanofood) are also used to improve the functional properties of food.
In the sensing field, nanomaterials have significantly improved transduction mechanisms and enhanced detection sensitivity of a wide range of targets such as bacterial pathogens, allergens, toxicants like heavy metal and pesticides, antibiotics, freshness and ripening indicators (ethylene and CO2), food antioxidants and nutraceuticals. Challenges remain to (1) integrate sensing technology into food packaging and translate the technology into real world applications, (2) improve selectivity and remove matrix effects due to the complexity of food samples and (3) the need for pre-treatment. In certain cases, continuous real-time monitoring might be needed. An example is a continuous flow sensor for foodstuff such as milk that requires real-time measurement of crude samples without contamination of the stream.180 In packaging, nanomaterials provide active functionalities by delivering antibacterial activity (e.g. AgNPs), UV-protective, moisture and gas barrier properties (e.g. TiO2, ZnO, MgO, CNC).
8 Future outlook
Although a great deal of research has demonstrated benefits of using nanomaterials for improving food monitoring, quality and freshness, studies of their toxicity, fate, transport and degradation is only in their infancy. Before their full potential can be realized, there need to be more studies and a thorough life cycle analysis of nanomaterials in food and the environment. Understanding of their risks requires developments in several areas. First, methods must be developed to characterize and measure the properties of nanomaterials, and their biotransformation in food. Conventional methods have limited use because of their high cost, inability to measure large number of samples and the complexity of food matrices. Second, more studies are needed to evaluate the toxicity of nanomaterials, either added as ingredients to food, or in contact with food (e.g. nanomaterials in packaging). Third, cost, biocompatibility and scale up should be further addressed for successful implementation. Future efforts are needed to ensure long-term stability and storage of sensing elements in field conditions, as well as validation of the technology. Developers of food nanotechnology products should also pay attention to industry and consumer acceptance, and willingness to pay. Migration of nanomaterials from packaging into food and the environment is a further concern that requires a better understanding. These issues should be evaluated as part of the development process. Four, the use of nanotechnologies in food lacks a regulatory framework and creates consumer reluctance to use nano-enabled food. Fifth, standards and a better classification and characterization of nano-products as well as labeling are needed to address consumer demands and regulatory issues, and ultimately accelerate commercial adoption. These topics are the subjects of large scale conferences and discussion forums. The Institute of Food Technologists (IFT) has regular issues and is hosting topical sessions discussing the use and potential implementation of nanotechnologies in food; and such activities are expected to be increased in the future.
In summary, nanotechnology provides innovative solutions to improve quality, produce, protect and analyze food products. However, concerns about toxicity have raised a number of questions regarding the health risks and environmental impact. This review provided an overview of recent advances and potential applications highlighting risks and benefits of these technologies. Efforts should concentrate in the future to ensure the safe use of nanotechnology in the food sector and its possible implications.
Conflicts of interest
There is no conflict of interest to declare.
Acknowledgements
This work was funded by the National Science Foundation under Grant No. 1561491. Any opinions, findings, and conclusions or recommendations expressed in this material are those of the author(s) and do not necessarily reflect the views of the National Science Foundation.
References
- S. Kargozar and M. Mozafari, Nanotechnology and Nanomedicine: Start small, think big, Mater. Today, 2018, 5(7), 15492–15500 CAS
. - A. L. Chun, Will the public swallow nanofood?, Nat. Nanotechnol., 2009, 4(12), 790 CrossRef CAS PubMed
. - X. He, et al., Regulation and safety of nanotechnology in the food and agriculture industry, Food Applications of Nanotechnology, Taylor & Francis Group, Boca Raton, 2019, vol. 12 Search PubMed
. - Q. Chaudhry, et al., Applications and implications of nanotechnologies for the food sector, Food Addit. Contam., Part A, 2008, 25(3), 241–258 CrossRef CAS PubMed
. - F. Gallocchio, S. Belluco and A. Ricci, Nanotechnology and food: brief overview of the current scenario, Procedia Food Sci., 2015, 5, 85–88 CrossRef
. - Commission Regulation (EU) No 10/2011 of 14 January 2011 on plastic materials and articles intended to come into contact with food. Official Journal of the European Union 15.1.2011.
- T. Joseph and M. Morrison, Nanotechnology in agriculture and food, Nanoforum Report, 2006, 2, p. 2–3 Search PubMed
. - M. Fathi, M. R. Mozafari and M. Mohebbi, Nanoencapsulation of food ingredients using lipid based delivery systems, Trends Food Sci. Technol., 2012, 23(1), 13–27 CrossRef CAS
. - M. Fathi, A. Martin and D. J. McClements, Nanoencapsulation of food ingredients using carbohydrate based delivery systems, Trends Food Sci. Technol., 2014, 39(1), 18–39 CrossRef CAS
. - I. Katouzian and S. M. Jafari, Nano-encapsulation as a promising approach for targeted delivery and controlled release of vitamins, Trends Food Sci. Technol., 2016, 53, 34–48 CrossRef CAS
. - M. Haham, et al., Stability and bioavailability of vitamin D nanoencapsulated in casein micelles, Food Funct., 2012, 3(7), 737–744 RSC
. - T. Ghorbanzade, et al., Nano-encapsulation of fish oil in nano-liposomes and its application in fortification of yogurt, Food Chem., 2017, 216, 146–152 CrossRef CAS PubMed
. - M. Carbone, et al., Silver nanoparticles in polymeric matrices for fresh food packaging, J. King Saud Univ., Sci., 2016, 28(4), 273–279 CrossRef
. - K. Majeed, et al., Potential materials for food packaging from nanoclay/natural fibres filled hybrid composites, Mater. Des., 2013, 46, 391–410 CrossRef CAS
. - A. Mills, et al., An O 2 smart plastic film for packaging, Analyst, 2012, 137(1), 106–112 RSC
. - X. B. Liu, et al., Biosensors based on modularly designed synthetic peptides for recognition, detection and live/dead differentiation of pathogenic bacteria, Biosens. Bioelectron., 2016, 80, 9–16 CrossRef CAS PubMed
. - N. Sanvicens, et al., Nanoparticle-based biosensors for detection of pathogenic bacteria, TrAC, Trends Anal. Chem., 2009, 28(11), 1243–1252 CrossRef CAS
. - O. R. Miranda, et al., Colorimetric Bacteria Sensing Using a Supramolecular Enzyme-Nanoparticle Biosensor, J. Am. Chem. Soc., 2011, 133(25), 9650–9653 CrossRef CAS PubMed
. - X. Yan, H. Li and X. Su, Review of optical sensors for pesticides, TrAC, Trends Anal. Chem., 2018, 103, 1–20 CrossRef CAS
. - D. Wu, D. Du and Y. Lin, Recent progress on nanomaterial-based biosensors for veterinary drug residues in animal-derived food, TrAC, Trends Anal. Chem., 2016, 83, 95–101 CrossRef CAS
. - W. Cheung, et al., Quantitative analysis of the banned food dye Sudan-1 using surface enhanced Raman scattering with multivariate chemometrics, J. Phys. Chem. C, 2010, 114(16), 7285–7290 CrossRef CAS
. - C. Wang, et al., Multiple signal amplification electrochemiluminescent immunoassay for Sudan I using gold nanorods functionalized graphene oxide and palladium/aurum core-shell nanocrystallines as labels, Electrochim. Acta, 2018, 278, 352–362 CrossRef CAS
. - J. Wang, et al., Nanocolloidal gold-based immuno-dip strip assay for rapid detection of Sudan red I in food samples, Food Chem., 2013, 136(3–4), 1478–1483 CrossRef CAS PubMed
. - S. Y. Oh, et al., Cuvette-Type LSPR Sensor for Highly Sensitive Detection of Melamine in Infant Formulas, Sensors, 2019, 19(18), 3839 CrossRef CAS PubMed
. - T. Anirudhan, V. Athira and V. C. Sekhar, Electrochemical sensing and nano molar level detection of Bisphenol-A with molecularly imprinted polymer tailored on multiwalled carbon nanotubes, Polymer, 2018, 146, 312–320 CrossRef CAS
. - P. Deng, Z. Xu and Y. Kuang, Electrochemical determination of bisphenol A in plastic bottled drinking water and canned beverages using a molecularly imprinted chitosan–graphene composite film modified electrode, Food Chem., 2014, 157, 490–497 CrossRef CAS PubMed
. - N. B. Messaoud, et al., Electrochemical sensor based on multiwalled carbon nanotube and gold nanoparticle modified electrode for the sensitive detection of bisphenol A, Sens. Actuators, B, 2017, 253, 513–522 CrossRef
. - Y. Zhu, et al., Building an aptamer/graphene oxide FRET biosensor for one-step detection of bisphenol A, ACS Appl. Mater. Interfaces, 2015, 7(14), 7492–7496 CrossRef CAS PubMed
. - S. Neethirajan, et al., Nano-biosensor platforms for detecting food allergens–New trends, Sensing and Bio-Sensing Research, 2018, 18, 13–30 CrossRef
. - X. Lin, Y. Ni and S. Kokot, Glassy carbon electrodes modified with gold nanoparticles for the simultaneous determination of three food antioxidants, Anal. Chim. Acta, 2013, 765, 54–62 CrossRef CAS PubMed
. - E. Sharpe, et al., Portable ceria nanoparticle-based assay for rapid detection of food antioxidants (NanoCerac), Analyst, 2013, 138(1), 249–262 RSC
. - J. Wang, et al., Facile synthesis of Au-coated magnetic nanoparticles and their application in bacteria detection via a SERS method, ACS Appl. Mater. Interfaces, 2016, 8(31), 19958–19967 CrossRef CAS PubMed
. - R. S. J. Alkasir, et al., Enzyme functionalized nanoparticles for electrochemical biosensors: a comparative study with applications for the detection of bisphenol A, Biosens. Bioelectron., 2010, 26(1), 43–49 CrossRef CAS PubMed
. - K. S. Abhijith, et al., Immobilised tyrosinase-based biosensor for the detection of tea polyphenols, Anal. Bioanal. Chem., 2007, 389(7–8), 2227–2234 CrossRef CAS PubMed
. - M. Sys, et al., Improvement of enzyme carbon paste-based biosensor using carbon nanotubes for determination of water-soluble analogue of vitamin E, Chem. Pap., 2015, 69(1), 150–157 CAS
. - V. Andrei, et al., A single use electrochemical sensor based on biomimetic nanoceria for the detection of wine antioxidants, Talanta, 2016, 156, 112–118 CrossRef PubMed
. - Y. Yang, et al., Electrochemical evaluation of total antioxidant capacities in fruit juice based on the guanine/graphene nanoribbon/glassy carbon electrode, Talanta, 2013, 106, 206–211 CrossRef CAS PubMed
. - J. F. Liu, et al., Antioxidant redox sensors based on DNA modified carbon screen-printed electrodes, Anal. Chem., 2006, 78(19), 6879–6884 CrossRef CAS
. - L. Augustin, et al., Glycemic index in chronic disease: a review, Eur. J. Clin. Nutr., 2002, 56(11), 1049–1071 CrossRef CAS PubMed
. - L. C. Clark Jr and C. Lyons, Electrode systems for continuous monitoring in cardiovascular surgery, Ann N. Y. Acad. Sci., 1962, 102(1), 29–45 CrossRef CAS PubMed
. - K. E. Toghill and R. G. Compton, Electrochemical non-enzymatic glucose sensors: a perspective and an evaluation, Int. J. Electrochem. Sci., 2010, 5(9), 1246–1301 CAS
. - Q. Chen, L. Zhang and G. Chen, Facile preparation of graphene-copper nanoparticle composite by in situ chemical reduction for electrochemical sensing of carbohydrates, Anal. Chem., 2012, 84(1), 171–178 CrossRef CAS PubMed
. - Y. Fu, L. Zhang and G. Chen, Preparation of a carbon nanotube-copper nanoparticle hybrid by chemical reduction for use in the electrochemical sensing of carbohydrates, Carbon, 2012, 50(7), 2563–2570 CrossRef CAS
. - F. Terzi, et al., Amperometric paper sensor based on Cu nanoparticles for the determination of carbohydrates, Sens. Actuators, B, 2017, 245, 352–358 CrossRef CAS
. - D. Vilela, M. C. Gonzalez and A. Escarpa, Sensing colorimetric approaches based on gold and silver nanoparticles aggregation: chemical creativity behind the assay. A review, Anal. Chim. Acta, 2012, 751, 24–43 CrossRef CAS PubMed
. - A. Szydlowska-Czerniak, A. Tulodziecka and E. Szlyk, A silver nanoparticle-based method for determination of antioxidant capacity of rapeseed and its products, Analyst, 2012, 137(16), 3750–3759 RSC
. - A. Szydłowska-Czerniak, A. Tułodziecka and E. Szłyk, A silver nanoparticle-based method for determination of antioxidant capacity of rapeseed and its products, Analyst, 2012, 137(16), 3750–3759 RSC
. - Y. Wang, et al., Morphological control of nanoprobe for colorimetric antioxidant detection, Biosens. Bioelectron., 2018, 122, 183–188 CrossRef CAS PubMed
. - E. Sharpe, et al., Metal oxide based multisensor array and portable database for field analysis of antioxidants, Sens. Actuators, B, 2014, 193, 552–562 CrossRef CAS PubMed
. - L. He, et al., Rapid and sensitive colorimetric detection of ascorbic acid in food based on the intrinsic oxidase-like activity of MnO2 nanosheets, Luminescence, 2018, 33(1), 145–152 CrossRef CAS PubMed
. - D. S. Lim, et al., Potential risk of bisphenol A migration from polycarbonate containers after heating, boiling, and microwaving, J. Toxicol. Environ. Health, Part A, 2009, 72(21–22), 1285–1291 CrossRef CAS PubMed
. - F. Cheli, et al., Mycotoxin Analysis, Mycotoxin-Producing Fungi Assays and Mycotoxin Toxicity Bioassays in Food Mycotoxin Monitoring and Surveillance, Ital. J. Food Sci., 2008, 20(4), 447–462 CAS
. - A. Hayat, et al., Recent advances in ochratoxin A-producing fungi detection based on PCR methods and ochratoxin A analysis in food matrices, Food Control, 2012, 26(2), 401–415 CrossRef CAS
. - E. Petzinger and K. Ziegler, Ochratoxin A from a toxicological perspective, J. Vet. Pharmacol. Therapeut., 2000, 23(2), 91–98 CrossRef CAS PubMed
. - G. Bülbül, A. Hayat and S. Andreescu, ssDNA-Functionalized Nanoceria: A Redox-Active Aptaswitch for Biomolecular Recognition, Adv. Healthcare Mater., 2016, 5(7), 822–828 CrossRef PubMed
. - L. Zhihua, et al., A novel sensor for determination of dopamine in meat based on ZnO-decorated reduced graphene oxide composites, Innovative Food Sci. Emerging Technol., 2015, 31, 196–203 CrossRef
. - A. Hayat, et al., Redox reactivity of cerium oxide nanoparticles against dopamine, J. Colloid Interface Sci., 2014, 418, 240–245 CrossRef CAS PubMed
. - G. Bülbül, et al., Reactivity of nanoceria particles exposed to biologically relevant catechol-containing molecules, RSC Adv., 2016, 6(65), 60007–60014 RSC
. - J. Njagi, et al., Amperometric detection of dopamine in vivo with an enzyme based carbon fiber microbiosensor, Anal. Chem., 2010, 82(3), 989–996 CrossRef CAS PubMed
. - Y. Li, J. Xu and C. Sun, Chemical sensors and biosensors for the detection of melamine, RSC Adv., 2015, 5(2), 1125–1147 RSC
. - J.-S. Chen, A worldwide food safety concern in 2008—melamine-contaminated infant formula in China caused urinary tract stone in 290 000 children in China, LWW, 2009 Search PubMed
. - Y. Jiang, et al., Ultrasensitive analysis of kanamycin residue in milk by SERS-based aptasensor, Talanta, 2019, 197, 151–158 CrossRef CAS PubMed
. - Y. Chen, et al., Gold immunochromatographic sensor for the rapid detection of twenty-six sulfonamides in foods, Nano Res., 2017, 10(8), 2833–2844 CrossRef CAS
. - W. Aktar, D. Sengupta and A. Chowdhury, Impact of pesticides use in agriculture: their benefits and hazards, Interdiscipl. Toxicol., 2009, 2(1), 1–12 Search PubMed
. - M. M. Tunesi, et al., Functionalised CuO nanostructures for the detection of organophosphorus pesticides: a non-enzymatic inhibition approach coupled with nano-scale electrode engineering to improve electrode sensitivity, Sens. Actuators, B, 2018, 260, 480–489 CrossRef CAS
. - J. A. Hondred, et al., Printed graphene electrochemical biosensors fabricated by inkjet maskless lithography for rapid and sensitive detection of organophosphates, ACS Appl. Mater. Interfaces, 2018, 10(13), 11125–11134 CrossRef CAS PubMed
. - A. N. Ivanov, et al., Acetylcholinesterase biosensor based on single-walled carbon nanotubes-Co phtalocyanine for organophosphorus pesticides detection, Talanta, 2011, 85(1), 216–221 CrossRef CAS PubMed
. - D. Rawtani, et al., Nanotechnology-based recent approaches for sensing and remediation of pesticides, J. Environ. Manage., 2018, 206, 749–762 CrossRef CAS
. - F. Arduini, et al., Nanomaterials in electrochemical biosensors for pesticide detection: advances and challenges in food analysis, Microchim. Acta, 2016, 183(7), 2063–2083 CrossRef CAS
. - M. Labib, E. H. Sargent and S. O. Kelley, Electrochemical Methods for the Analysis of Clinically Relevant Biomolecules, Chem. Rev., 2016, 116(16), 9001–9090 CrossRef CAS
. - WHO Global burden of foodborne diseases, 2015 Search PubMed.
- Centers for Disease Control and Prevention, C., Surveillance for foodborne disease outbreaks--United States, 2009-2010. MMWR. Morbidity and mortality weekly report, 2013, vol. 62(3), p. 41 Search PubMed
. - Centers for Disease Control and Prevention, Surveillance for Foodborne Disease Outbreaks, United States, 2011, Annual Report, US Department
of Health and Human Services, Atlanta, Georgia, editor, 2014 Search PubMed
. - Centers for Disease Control and Prevention, Surveillance for Foodborne Disease Outbreaks, United States, 2012, Annual Report, US Department of Health and Human Services, Atlanta, Georgia, editor, 2014 Search PubMed
. - Centers for Disease Control and Prevention, Surveillance for Foodborne Disease Outbreaks, United States, 2013, Annual Report, US Department of Health and Human Services, Atlanta, Georgia, editor, 2015 Search PubMed
. - E. de Boer and R. R. Beumer, Methodology for detection and typing of foodborne microorganisms, Int. J. Food Microbiol., 1999, 50(1–2), 119–130 CrossRef CAS PubMed
. - F. Mustafa, R. Y. A. Hassan and S. Andreescu, Multifunctional Nanotechnology-Enabled Sensors for Rapid Capture and Detection of Pathogens, Sensors, 2017, 17(9), 2121 CrossRef
. - M. Zhong, et al., An electrochemical immunobiosensor for ultrasensitive detection of Escherichia coli O157:H7 using CdS quantum dots-encapsulated metal-organic frameworks as signal-amplifying tags, Biosens. Bioelectron., 2019, 126, 493–500 CrossRef CAS
. - A. S. Afonso, et al., Electrochemical detection of Salmonella using gold nanoparticles, Biosens. Bioelectron., 2013, 40(1), 121–126 CrossRef CAS
. - H. Vaisocherová-Lísalová, et al., Low-fouling surface plasmon resonance biosensor for multi-step detection of foodborne bacterial pathogens in complex food samples, Biosens. Bioelectron., 2016, 80, 84–90 CrossRef
. - A. Turner, Heavy metals in the glass and enamels of consumer container bottles, Environ. Sci. Tech., 2019, 53(14), 8398–8404 CrossRef CAS
. - B. W. Redan, Processing aids in food and beverage manufacturing: Potential source of elemental and trace metal contaminants, J. Agric. Food Chem., 2020 Search PubMed
. - M. A. Baki, et al., Concentration of heavy metals in seafood (fishes, shrimp, lobster and crabs) and human health assessment in Saint Martin Island, Bangladesh, Ecotoxicol Environ Saf., 2018, 159, 153–163 CrossRef
. - E. P. Nardi, et al., The use of inductively coupled plasma mass spectrometry (ICP-MS) for the determination of toxic and essential elements in different types of food samples, Food Chem., 2009, 112(3), 727–732 CrossRef CAS
. - A. A. Gouda and S. M. Al Ghannam, Impregnated multiwalled carbon nanotubes as efficient sorbent for the solid phase extraction of trace amounts of heavy metal ions in food and water samples, Food Chem., 2016, 202, 409–416 CrossRef CAS
. - Y. Zhou, et al., Current progress in biosensors for heavy metal ions based on DNAzymes/DNA molecules functionalized nanostructures: A review, Sens. Actuators, B, 2016, 223, 280–294 CrossRef CAS
. - J. Xu, et al., A review of functionalized carbon nanotubes and graphene for heavy metal adsorption from water: Preparation, application, and mechanism, Chemosphere, 2018, 195, 351–364 CrossRef CAS
. - J. Wei, et al., Stripping voltammetric determination of mercury (II) based on SWCNT-PhSH modified gold electrode, Sens. Actuators, B, 2014, 190, 968–974 CrossRef CAS
. - M. Baghayeri, et al., A simple approach for simultaneous detection of cadmium (II) and lead (II) based on glutathione coated magnetic nanoparticles as a highly selective electrochemical probe, Sens. Actuators, B, 2018, 273, 1442–1450 CrossRef CAS
. - S. L. Ting, et al., Graphene quantum dots functionalized gold nanoparticles for sensitive electrochemical detection of heavy metal ions, Electrochim. Acta, 2015, 172, 7–11 CrossRef CAS
. - N. Ullah, et al., Nanomaterial-based optical chemical sensors for the detection of heavy metals in water: Recent advances and challenges, TrAC, Trends Anal. Chem., 2018, 100, 155–166 CrossRef CAS
. - K. A. Kirk and S. Andreescu, Easy-to-Use Sensors for Field Monitoring of Copper Contamination in Water and Pesticide-Sprayed Plants, Anal. Chem., 2019, 91(21), 13892–13899 CrossRef CAS
. - T. Rasheed, et al., Fluorescent sensor based models for the detection of environmentally-related toxic heavy metals, Sci. Total Environ., 2018, 615, 476–485 CrossRef CAS
. - J. S. Lee, M. S. Han and C. A. Mirkin, Colorimetric detection of mercuric ion (Hg2+) in aqueous media using DNA-functionalized gold nanoparticles, Angew. Chem., Int. Ed., 2007, 46(22), 4093–4096 CrossRef CAS PubMed
. - Z. Wang, et al., Fluorescence sensor array based on amino acid derived carbon dots for pattern-based detection of toxic metal ions, Sens. Actuators, B, 2017, 241, 1324–1330 CrossRef CAS
. - I. S. Arvanitoyannis, Totally and partially biodegradable polymer blends based on natural and synthetic macromolecules: preparation, physical properties, and potential as food packaging materials. 1999 Search PubMed
. - C. Sharma, et al., Nanotechnology: An Untapped Resource for Food Packaging, Front Microbiol., 2017, 8, 1735 CrossRef PubMed
. - S. Lambert and M. Wagner, Environmental performance of bio-based and biodegradable plastics: the road ahead, Chem. Soc. Rev., 2017, 46(22), 6855–6871 RSC
. - S. Flores, et al., Physical properties of tapioca-starch edible films: Influence of filmmaking and potassium sorbate, Food Res Int., 2007, 40(2), 257–265 CrossRef CAS
. - V. Goudarzi, I. Shahabi-Ghahfarrokhi and A. Babaei-Ghazvini, Preparation of ecofriendly UV-protective food packaging material by starch/TiO2 bio-nanocomposite: Characterization, Int. J. Biol. Macromol., 2017, 95, 306–313 CrossRef CAS PubMed
. - A. Ashori and R. Bahrami, Modification of physico-mechanical properties of chitosan-tapioca starch blend films using nano graphene, Polym.-Plast. Technol. Eng., 2014, 53(3), 312–318 CrossRef CAS
. - S.-D. Yoon, M.-H. Park and H.-S. Byun, Mechanical and water barrier properties of starch/PVA composite films by adding nano-sized poly(methyl methacrylate-co-acrylamide) particles, Carbohydr. Polym., 2012, 87(1), 676–686 CrossRef CAS
. - C. Liu, et al., Effects of nano-TiO2 on the performance of high-amylose starch based antibacterial films, J. Appl. Polym. Sci., 2015, 132(32), 477–486 Search PubMed
. - H. Wang, J. Qian and F. Ding, Emerging chitosan-based films for food packaging applications, J. Agric. Food Chem., 2018, 66(2), 395–413 CrossRef CAS PubMed
. - S. Sanuja, A. Agalya and M. J. Umapathy, Synthesis and characterization of zinc oxide–neem oil–chitosan bionanocomposite for food packaging application, Int. J. Biol. Macromol., 2015, 74, 76–84 CrossRef CAS
. - L. Al-Naamani, S. Dobretsov and J. Dutta, Chitosan-zinc oxide nanoparticle composite coating for active food packaging applications, Innovative Food Sci. Emerging Technol., 2016, 38, 231–237 CrossRef CAS
. - S. Sanuja, A. Agalya and M. Umapathy, Studies on magnesium oxide reinforced chitosan bionanocomposite incorporated with clove oil for active food packaging application, Int. J. Polym. Mater. Polym. Biomater., 2014, 63(14), 733–740 CrossRef CAS
. - R. De Silva, et al., Nano-MgO reinforced chitosan nanocomposites for high performance packaging applications with improved mechanical, thermal and barrier properties, Carbohydr. Polym., 2017, 157, 739–747 CrossRef CAS
. - Z. Lian, Y. Zhang and Y. Zhao, Nano-TiO2 particles and high hydrostatic pressure treatment for improving functionality of polyvinyl alcohol and chitosan composite films and nano-TiO2 migration from film matrix in food simulants, Innovat Food Sci Emerg Techol., 2016, 33, 145–153 CrossRef CAS
. - A. Khan, et al., Mechanical and barrier properties of nanocrystalline cellulose reinforced chitosan based nanocomposite films, Carbohydr. Polym., 2012, 90(4), 1601–1608 CrossRef CAS PubMed
. - K. A. Kirk, A. Othman and S. Andreescu, Nanomaterial-functionalized Cellulose: Design, Characterization and Analytical Applications, Anal. Sci., 2018, 34(1), 19–31 CrossRef CAS
. - Y. Habibi, L. A. Lucia and O. J. Rojas, Cellulose nanocrystals: chemistry, self-assembly, and applications, Chem. Rev., 2010, 110(6), 3479–3500 CrossRef CAS PubMed
. - H. Yu, C. Yan and J. Yao, Fully biodegradable food packaging materials based on functionalized cellulose nanocrystals/poly(3-hydroxybutyrate-co-3-hydroxyvalerate) nanocomposites, RSC Adv., 2014, 4(104), 59792–59802 RSC
. - Z. Wu, et al., Multifunctional nano-cellulose composite films with grape seed extracts and immobilized silver nanoparticles, Carbohydr. Polym., 2019, 205, 447–455 CrossRef CAS
. - E. Fortunati, et al., Effects of modified cellulose nanocrystals on the barrier and migration properties of PLA nano-biocomposites, Carbohydr. Polym., 2012, 90(2), 948–956 CrossRef CAS PubMed
. - H. Zhang and G. Mittal, Biodegradable protein-based films from plant resources: A review, Environ. Prog. Sustainable Energy, 2010, 29(2), 203–220 CrossRef CAS
. - Y. Zhang, Q. Liu and C. Rempel, Processing and characteristics of canola protein-based biodegradable packaging: A review, Crit. Rev. Food Sci. Nutr., 2018, 58(3), 475–485 CAS
. - J. George, High performance edible nanocomposite films containing bacterial cellulose nanocrystals, Carbohydr. Polym., 2012, 87(3), 2031–2037 CrossRef CAS
. - P. Oymaci and S. A. Altinkaya, Improvement of
barrier and mechanical properties of whey protein isolate based food packaging films by incorporation of zein nanoparticles as a novel bionanocomposite, Food Hydrocolloids, 2016, 54, 1–9 CrossRef CAS
. - R. Sothornvit, J.-W. Rhim and S.-I. Hong, Effect of nano-clay type on the physical and antimicrobial properties of whey protein isolate/clay composite films, J. Food Eng., 2009, 91(3), 468–473 CrossRef CAS
. - S. Tang, et al., Ecofriendly and Biodegradable Soybean Protein Isolate Films Incorporated with ZnO Nanoparticles for Food Packaging, ACS Appl. Bio Mater., 2019, 2(5), 2202–2207 CrossRef CAS
. - A. J. Svagan, et al., Transparent films based on PLA and montmorillonite with tunable oxygen barrier properties, Biomacromolecules, 2012, 13(2), 397–405 CrossRef CAS PubMed
. - A. M. Díez-Pascual and A. L. Diez-Vicente, ZnO-reinforced poly(3-hydroxybutyrate-co-3-hydroxyvalerate) bionanocomposites with antimicrobial function for food packaging, ACS Appl. Mater. Interfaces, 2014, 6(12), 9822–9834 CrossRef PubMed
. - R. L. Scharff, The Economic Burden of Foodborne Illness in the United States, in Food Safety Economics, Springer, 2018, pp. 123–142 Search PubMed
. - J. Wyrwa and A. Barska, Innovations in the food packaging market: Active packaging, Eur. Food Res. Technol., 2017, 243(10), 1681–1692 CrossRef CAS
. - K. K. Gaikwad, S. Singh and Y. S. Lee, Oxygen scavenging films in food packaging, Environ. Chem. Lett., 2018, 16(2), 523–538 CrossRef CAS
. - K. K. Gaikwad, S. Singh and A. Ajji, Moisture absorbers for food packaging applications, Environ. Chem. Lett., 2019, 17(2), 609–628 CrossRef CAS
. - K. Sadeghi, Y. Lee and J. Seo, Ethylene Scavenging Systems in Packaging of Fresh Produce: A Review, Food Rev. Int., 2019, 1–22 CrossRef
. - J. W. Han, et al., Food packaging: A comprehensive review and future trends, Compr. Rev. Food Sci. Food Saf., 2018, 17(4), 860–877 CrossRef
. - K. L. Yam, P. T. Takhistov and J. Miltz, Intelligent packaging: concepts and applications, J. Food Sci., 2005, 70(1), R1–R10 CrossRef CAS
. - F. Mustafa and S. Andreescu, Chemical and biological sensors for food-quality monitoring and smart packaging, Foods, 2018, 7(10), 168 CrossRef CAS PubMed
. - S. Wang, et al., Review of time temperature indicators as quality monitors in food packaging, Packag. Technol. Sci., 2015, 28(10), 839–867 CrossRef CAS
. - V. A. Pereira Jr, I. N. Q. de Arruda and R. Stefani, Active chitosan/PVA films with anthocyanins from Brassica oleraceae (Red Cabbage) as time–temperature indicators for application in intelligent food packaging, Food Hydrocolloids, 2015, 43, 180–188 CrossRef
. - J. U. Kim, et al., Kinetic modeling and characterization of a diffusion-based time-temperature indicator (TTI) for monitoring microbial quality of non-pasteurized angelica juice, LWT--Food Sci. Technol., 2016, 67, 143–150 CrossRef CAS
. - C. Zhang, et al., Time–Temperature indicator for perishable products based on kinetically programmable Ag overgrowth on Au nanorods, ACS Nano, 2013, 7(5), 4561–4568 CrossRef CAS PubMed
. - Y.-C. Wang, L. Lu and S. Gunasekaran, Gold nanoparticle-based thermal history indicator for monitoring low-temperature storage, Microchim. Acta, 2015, 182(7–8), 1305–1311 CrossRef CAS
. - J. H. Han, Antimicrobial food packaging, Novel Food Packag. Tech., 2003, 8, 50–70 Search PubMed
. - R. Gu, et al., Regenerated Cellulose Films with Amino-terminated Hyperbranched Polyamic Anchored Nano-silver for Active Food Packaging, ACS Appl. Bio Mater., 2020, 3(1), 602–610 CrossRef CAS
. - L. Valencia, et al., Biobased Cellulose Nanofibril–Oil Composite Films for Active Edible Barriers, ACS Appl. Mater. Interfaces, 2019, 11(17), 16040–16047 CrossRef CAS PubMed
. - M. Cruz-Romero, et al., Antimicrobial activity of chitosan, organic acids and nano-sized solubilisates for potential use in smart antimicrobially-active packaging for potential food applications, Food Control, 2013, 34(2), 393–397 CrossRef CAS
. - X. Zhang, et al., Preparation of chitosan-TiO2 composite film with efficient antimicrobial activities under visible light for food packaging applications, Carbohydr. Polym., 2017, 169, 101–107 CrossRef CAS PubMed
. - P. M. Rahman, V. A. Mujeeb and K. Muraleedharan, Flexible chitosan-nano ZnO antimicrobial pouches as a new material for extending the shelf life of raw meat, Int. J. Biol. Macromol., 2017, 97, 382–391 CrossRef CAS
. - N. A. El-Wakil, et al., Development of wheat gluten/nanocellulose/titanium dioxide nanocomposites for active food packaging, Carbohydr. Polym., 2015, 124, 337–346 CrossRef CAS PubMed
. - K. K. Gaikwad, S. Singh and Y. S. Negi, Ethylene scavengers for active packaging of fresh food produce, Environ. Chem. Lett., 2020, 269–284 CrossRef CAS
. - M. Hussain, et al., Photocatalytic degradation of ethylene emitted by fruits with TiO2 nanoparticles, Ind. Eng. Chem. Res., 2010, 50(5), 2536–2543 CrossRef
. - Z. Zhu, et al., Preparation of PAN@TiO2 Nanofibers for Fruit Packaging Materials with Efficient Photocatalytic Degradation of Ethylene, Materials, 2019, 12(6), 896 CrossRef CAS PubMed
. - Z. Zhu, et al., Electrospun nanofibers containing TiO2 for the photocatalytic degradation of ethylene and delaying postharvest ripening of bananas, Food Bioprocess Technol., 2019, 12(2), 281–287 CrossRef CAS
. - Z. Zhu, et al., Electrospun Nanofibers Containing TiO2 for the Photocatalytic Degradation of Ethylene and Delaying Postharvest Ripening of Bananas, Food Bioprocess Technol., 2019, 12(2), 281–287 CrossRef CAS
. - M. L. V. de Chiara, et al., Photocatalytic degradation of ethylene on mesoporous TiO2/SiO2 nanocomposites: Effects on the ripening of mature green tomatoes, Biosyst. Eng., 2015, 132, 61–70 CrossRef
. - A. Dey and S. Neogi, Oxygen scavengers for food packaging applications: A review, Trends Food Sci. Technol., 2019, 90, 26–34 CrossRef CAS
. - M. J. Khalaj, et al., Study of physical and mechanical properties of polypropylene nanocomposites for food packaging application: Nano-clay modified with iron nanoparticles, Trends Food Sci. Technol., 2016, 51, 41–48 CrossRef CAS
. - Z. Foltynowicz, et al., Nanoscale, zero valent iron particles for application as oxygen scavenger in food packaging, Food Packaging and Shelf Life, 2017, 11, 74–83 CrossRef
. - A. Cherpinski, et al., Electrospun oxygen scavenging films of poly(3-hydroxybutyrate) containing palladium nanoparticles for active packaging applications, Nanomaterials, 2018, 8(7), 469 CrossRef PubMed
. - B. Kuswandi, et al., A novel on-package sticker sensor based on methyl red for real-time monitoring of broiler chicken cut freshness, Packag. Technol. Sci., 2014, 27(1), 69–81 CrossRef CAS
. - B. Kuswandi and A. Nurfawaidi, On-package dual sensors label based on pH indicators for real-time monitoring of beef freshness, Food Control, 2017, 82, 91–100 CrossRef CAS
. - C. Schaude, et al., Developing a sensor layer for the optical detection of amines during food spoilage, Talanta, 2017, 170, 481–487 CrossRef CAS PubMed
. - K. El-Nour, et al., Gold nanoparticles as a direct and rapid sensor for sensitive analytical detection of biogenic amines, Nanoscale Res. Lett., 2017, 12(1), 231 CrossRef CAS PubMed
. - V. Putri, et al., Application Nano Zeolite-Molybdate For Avocado Ripeness Indicator, in IOP Conference Series: Earth and Environmental Science, IOP Publishing, 2019 Search PubMed
. - C. Lang and T. Hübert, A colour ripeness indicator for apples, Food Bioprocess Technol., 2012, 5(8), 3244–3249 CrossRef CAS
. - A. Mills, et al., An O-2 smart plastic film for packaging, Analyst, 2012, 137(1), 106–112 RSC
. - U. Siripatrawan and P. Kaewklin, Fabrication and characterization of chitosan-titanium dioxide nanocomposite film as ethylene scavenging and antimicrobial active food packaging, Food Hydrocolloids, 2018, 84, 125–134 CrossRef CAS
. - Y. N. Xia, M. Rubino and R. Auras, Release of Nanoclay and Surfactant from Polymer-Clay Nanocomposites into a Food Simulant, Environ. Sci. Technol., 2014, 48(23), 13617–13624 CrossRef CAS PubMed
. - A. Martirosyan and Y.-J. Schneider, Engineered nanomaterials in food: implications for food safety and consumer health, Int. J. Environ. Res. Public Health, 2014, 11(6), 5720–5750 CrossRef PubMed
. - C. V. Garcia, G. H. Shin and J. T. Kim, Metal oxide-based nanocomposites in food packaging: Applications, migration, and regulations, Trends Food Sci. Technol., 2018, 82, 21–31 CrossRef CAS
. - Q.-B. Lin, et al., Migration of Ti from nano-TiO2-polyethylene composite packaging into food simulants, Food Addit. Contam., Part A, 2014, 31(7), 1284–1290 CAS
. - P. Dhar, et al., Poly(3-hydroxybutyrate)/cellulose nanocrystal films
for food packaging applications: Barrier and migration studies, Polymer Eng. Sci., 2015, 55(10), 2388–2395 CrossRef CAS
. - M. E. Quadros, et al., Release of silver from nanotechnology-based consumer products for children, Environ. Sci. Technol., 2013, 47(15), 8894–8901 CrossRef CAS PubMed
. - Y. Teow, et al., Health impact and safety of engineered nanomaterials, Chem. Commun., 2011, 47(25), 7025–7038 RSC
. - S. Sharifi, et al., Toxicity of nanomaterials, Chem. Soc. Rev., 2012, 41(6), 2323–2343 RSC
. - E. F. S. Authority, The Potential Risks Arising from Nanoscience and Nanotechnologies on Food and Feed Safety, EFSA J., 2009, 7(3), 958 Search PubMed
. - E. S. Committee, Guidance on the risk assessment of the application of nanoscience and nanotechnologies in the food and feed chain, EFSA J., 2011, 9(5), 2140 CrossRef
. - Chain, E. P. o.C. i.t.F., Presence of microplastics and nanoplastics in food, with particular focus on seafood, EFSA J., 2016, 14(6), e04501 Search PubMed
. - R. Peters, et al., Inventory of Nanotechnology applications in the agricultural, feed and food sector, EFSA Supporting Publications, 2014, 11(7), 621E Search PubMed
. - E. F. S. Authority, R. Schoonjans and B. Eryasa, Annual report of the EFSA Scientific Network of Risk Assessment of Nanotechnologies in Food and Feed for 2018, EFSA Supporting Publications, 2019, 16(4), 1626E Search PubMed
. - V. Amenta, et al., Regulatory aspects of nanotechnology in the agri/feed/food sector in EU and non-EU countries, Regul. Toxicol. Pharmacol., 2015, 73(1), 463–476 CrossRef PubMed
. - A. Bieberstein, et al., Consumer choices for nano-food and nano-packaging in France and Germany, European Review of Agricultural Economics, 2012, 40(1), 73–94 CrossRef
. - K. D. Grieger, et al., International implications of labeling foods containing engineered nanomaterials, J. Food Prot., 2016, 79(5), 830–842 CrossRef CAS PubMed
. - J. Brown and J. Kuzma, Hungry for information: public attitudes toward food nanotechnology and labeling, Rev. Pol. Res., 2013, 30(5), 512–548 CrossRef
. - E. L. Giles, et al., Consumer acceptance of and willingness to pay for food nanotechnology: a systematic review, J. Nanopart. Res., 2015, 17(12), 467 CrossRef PubMed
. - H. Li, et al., Electrochemical DNA-based sensors for molecular quality control: continuous, real-time melamine detection in flowing whole milk, Anal. Chem., 2018, 90(18), 10641–10645 CrossRef CAS PubMed
.
|
This journal is © The Royal Society of Chemistry 2020 |
Click here to see how this site uses Cookies. View our privacy policy here.