DOI:
10.1039/D1NH00459J
(Minireview)
Nanoscale Horiz., 2022,
7, 31-40
Rare earth element based single-atom catalysts: synthesis, characterization and applications in photo/electro-catalytic reactions
Received
1st September 2021
, Accepted 9th November 2021
First published on 9th November 2021
Abstract
Rare earth elements play an important role in various fields, which has attracted increasing interest from the scientific community. Meanwhile, single-atom catalysts show huge advantages in many aspects compared with traditional nanomaterials due to their 100% atomic utilization efficiency. Thus, the combination of the two concepts has yielded an efficient way to realize the high-value utilization of rare earth elements. In this mini-review, rare earth-based single-atom catalysts including their synthesis methods, characterization means and corresponding applications are constructively summarized and discussed. In particular, the important roles of rare earth elements as active centers in photo/electrocatalytic reactions are focused on. Finally, future prospects are also provided.
1. Introduction
For traditional nanomaterials, only the outermost surface metal atoms are involved in the catalytic redox reactions, whereas most inner metal atoms cannot make contact with the target molecules of the reactants, leading to them being regarded as waste in catalysts. Single-atom catalysts (SACs) have received increasing attention in recent years due to their 100% atomic utilization efficiency.1–3 In 2011, Zhang and co-workers defined single-atom catalysts as materials consisting of only atomically isolated active sites.4 When the materials are limited to the atomic scale, they tend to show different activity, selectivity and stability from that of nanomaterials. Single-atom catalysis has evenly distributed undercoordinated sites, providing a model platform to bridge the gap between heterogeneous and homogeneous catalysis. The isolated metal atom supported on a suitable substrate has a relatively definite geometric configuration and electronic structure, which are beneficial for the exploration of the reaction mechanism.1 Lots of catalysts with single-atom active sites, including Ni, Fe, Co, Zn and so on, have been reported with unique performances.5–10 And more attention has been devoted to the limitation of atomic-level adsorption through the use of reaction intermediates, which has led to different catalytic behaviors.11–20
Rare earth elements are widely used in agriculture, military and industrial fields with indispensable roles. Product development and technological development will make full use of rare earth resources and greatly increase the added value of rare earth resources, especially the highly enriched rare earth elements such as La, Ce and Y. Therefore, the high-value utilization of rare earth resources has always been an important research direction for scientists.21–25 Rare earth single-atom catalysts (RE-SACs) are an effective way to realize the efficient utilization of rare earth resources. Meanwhile, rare earth elements may exhibit special reactivity at the single atomic scale, which is helpful to explore the special reactivity of rare earth elements and deepen the understanding of rare earth elements.1,26–29
Preparing catalysts with single atomic rare earth elements as active sites is an effective and more meaningful way to make full use of rare earth resources. Single atomic rare earth catalysts supported in different supports may exhibit different performances including activity, selectivity or stability compared with rare earth nanomaterials.27–29
So far, several important studies and reviews on single-atom catalysts have been published in different research fields including energy conversion, electrocatalysis and photocatalysis.1,26,30,31 For instance, Zhang et al. discussed the use of rare earth metals and their oxides in single-atom catalysts and the results.32 However a detailed discussion of the means of characterization of catalysts and a complete summary of this research area are lacking. Hence, our review will discuss the synthesis and characterization methods of rare earth based single-atom catalysts and their applications (Fig. 1). In particular, we will focus on the evaluation of the important roles of rare earth atoms as active centres for effective catalytic performance.
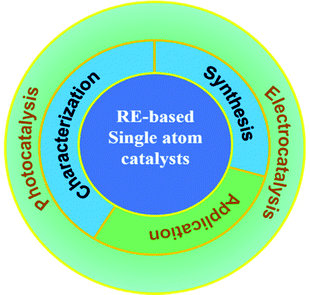 |
| Fig. 1 Scheme of synthesis, characterization, and applications of rare earth-based single-atom catalysts. | |
2. Synthesis
It has always been a challenge to find a suitable method for preparing single-atom catalysts, since the aggregation of metal atoms during synthesis or subsequent processing impedes the deposition of single-atoms on the surface of an appropriate support.1,31 In addition, the easier combination between rare earth elements and oxygen makes it harder to prepare RE-SACs.33 Therefore, various methods for the preparation of rare earth based single atomic materials were developed, which are summarized below.
2.1 Impregnation method
The impregnation method is the most applied strategy for the synthesis of rare earth based single-atom catalysts. In this method of synthesis, the metal precursor is mixed with the supports (usually metal oxides or carbon-based materials), and then the metal atoms or ions are gradually immersed into the surface of supports or entire frameworks, and finally, a heat treatment is performed to obtain the products. However, even after calcination at a moderate temperature, isolated atoms can easily be transformed into small clusters or nanoparticles on the supports. Thus, a low concentration of metal precursors is usually applied during the preparation of single-atom catalysts.31
For example, in Wang's report, atomically dispersed Pt species were formed on the surface of CeO2 nanoparticles with a mass loading of 0.05 wt%, whereas nanoclusters appeared with a larger mass loading of 2 wt%. The single atomic Pt/CeO2 also showed higher reactivity than nanoclustered Pt/CeO2 during the CO2 reduction process.34 Jiang et al. synthesized single-atom Pd deposited on CeO2 through impregnation and a further calcination process under an air atmosphere, which showed good performance in CO oxidation under low temperature.35 Besides the substrates of metal oxides, many single-atom catalysts anchored on carbon materials were prepared by the impregnation method. Li et al. synthesized highly dispersive cerium atoms on carbon nanowires by immersing Ce3+ into the black polymerization precipitate of cetyltrimethyl ammonium bromide (CTAB), ammonium peroxydisulfate (APS) and pyrrole, followed by a further high temperature treatment under a nitrogen atmosphere.36 Similarly, the authors also prepared Fe–N–C materials through the stabilization of ceria using the same method, which exhibited a high activity in oxygen reduction reaction (ORR).37
2.2 Solid-phase synthesis
Solid-phase synthesis is a widely applied method for the synthesis of SACs.38,39 Ball milling is usually applied in the process of solid-phase synthesis. During the process of ball milling, the required precursors are mixed thoroughly by the method of mechanical and physical mixing without solvents or other additives. Ball milling is a very versatile and extensible method for the preparation of heterogeneous catalysts, even single-atom catalysts. Meanwhile, mass production of single-atom catalysts can be realized through this method.40
Gan et al. prepared the single atomic Au1/CeO2 through ball-milling of cerium(III) acetate tetrahydrate and gold(III) acetate with further calcination in an air atmosphere. It is worth noting that the authors could obtain catalysts scaling up to 1.025 kg with a uniform grey white color. Ir1/CeO2, Au1/NiO, and Au1/ZnO were also synthesized successfully by the same method with different precursors, validating the applicability of this approach.40 Meanwhile, Liu et al. successfully synthesized Y1/NC and Sc1/NC catalysts via ball milling as electrocatalysts for N2 and CO2 reduction.27 Xu et al. synthesized single-atom Pd on (CeZrHfTiLa)Ox (HEFO) by ball milling and calcination, which showed a high activity for low temperature CO oxidation and excellent resistance to thermal and hydrothermal degradation.19
In addition, solid materials can be directly converted to single-atom catalysts through the gas-migration strategy. For example, Wu et al. reported a hard-template approach to synthesize the rare-earth single cerium-atom, wherein CeO2 was introduced in the gas-migration process. At 1150 °C in flowing N2, the surface Ce atoms of CeO2 were initially evaporated to form a possible volatile Ce species, which could be transported and trapped by the defective N-rich porous carbon, forming an atomically dispersed Ce SAS catalyst.29
2.3 Coprecipitation method
During the process of coprecipitation, two or more cations in the solution are precipitated after adding the precipitant. This method has the advantage of easy preparation, especially the preparation of single-atom catalysts with uniform distribution. For instance, Derevyannikova et al. mixed Ce(NO3)3 with Pt(NO3)4 solutions, then added 1 M KOH to the mixture to form a precipitate, and subsequently conducted calcination in air to obtain Pt–CeO2.11 In the synthesis of single-atom Cu–CeO2, CeO2 as a support was prepared through hydrothermal synthesis; the single atom catalysts were fabricated with Cu(NO3)2·3H2O as the precursor and Na2CO3 as the precipitant. The final catalyst showed high selectivity of methane with a Faradaic efficiency of 58% during the electrochemical CO2 reduction.41 The Ce single-atom catalysts loaded on NC were fabricated successfully through the coprecipitation of Zn(NO3)2·6H2O and 0.18 g of Ce(NO3)3·6H2O and 2-methylimidazole.29
2.4 Atomic layer deposition
Atomic layer deposition (ALD) is an emerging technology for synthesizing single-atom catalysts. In a typical ALD process, a metal precursor in the form of gas flows into the reaction chamber and is exposed to a second gas reactant to form an atomic layer on the pre-support substrate. During this process, the type, size density of nucleation sites and thickness can be tuned by controlling the type of precursor, deposition temperature and the number of ALD cycles.42,43 Ye et al. fabricated atomically dispersed Pt on the CeO2 nanorod using trimethyl(methylcyclopentadienyl)-platinum(IV) as the Pt precursor and ozone as the oxidant for one cycle.42 Similarly, Wang et al. prepared single atomic Pt1/CeO2 through the same method.44
2.5 Perspectives on the synthesis of RE-SACs
Among the many synthetic methods mentioned, the method proposed by Ji's report is a feasible method for the synthesis of RE-SACs. In this method, due to the abundant coordination nitrogen atoms provided by the sponge and urea and the ultralow temperature of liquid nitrogen, plenty of RE atoms are fixed in the sponge firmly to limit their migration randomly.28,45
At present, the vast majority of rare earth single atoms are supported on carbon-based supports. However, some metal oxides, such as CeO2, TiO2, Al2O3 and other oxides, with abundant surface defects can promote the loading and dispersion of single atoms on their surfaces or generate strong metal–support interactions. This may be beneficial to improve the thermal stability of the catalysts in some thermocatalytic reactions, thereby enhancing their catalytic activity, which is supposed to become a research topic in the future.46
Although there have been a lot of valuable explorations on the preparation process of rare earth-based single atom electrocatalysts, according to our thinking, accurate preparation of single-atom active sites at the molecular level based on appropriate precursors may be a fruitful solution. The key to address this challenge lies in the effective single atomization strategy and suitable scaffolds. As far as we know, metal–organic frameworks (MOFs) have been demonstrated to be great precursors/templates to produce single atomic materials, featured with diverse and tailorable structures and functionalities.47 For example, organic ligands with different coordination environments (such as tetrakis (4-carboxyphenyl)porphyrin) – TCPP are selected to pre-modify the active centre, and then rare earth metals are considered to coordinate with the modified organic ligands to generate MOF materials due to their better oxygen affinity.48 This method may achieve a high uniform dispersion of rare earth-based single-atoms, as well as a controllable and precise regulation of the type of active centres.
3. Characterization methods
Single-atom catalysts possess atomically dispersed metal atoms supported on substrates of metal oxide or carbon-based materials.49 In addition to the traditional characterization techniques including XRD, SEM, N2 adsorption and TEM which can provide the fundamental physicochemical properties of SACs, some emerging advanced techniques have been developed to better understand their fine structures. To date, the advanced characterization strategies including scanning tunneling microscopy (STM), high-angle annular dark-field scanning transmission electron microscopy (HAADF-STEM), synchrotron radiation photoelectron spectroscopy (SRPES), X-ray absorption spectroscopy (XAS) and some other characterization methods have been applied to characterize the atomic structures of single-atom catalysts. In the following discussion, we aim to discuss the related characterization methods with some detailed examples.30
3.1 Visual characterization techniques
3.1.1 Scanning tunneling microscopy (STM).
STM is a characterization technique for ultrahigh resolution imaging of surface and absorbing species at the atomic level with a lateral resolution of 0.1 nm and a depth resolution of 0.01 nm (10 pm). STM can be used in modern surface science to explore conducting surfaces and the adsorption processes on these surfaces.50,51 For example, Gao et al. utilized STM to reveal the role of the Au–CeOx interface in CO2RR, as shown in Fig. 2. At the same time, using in situ STM it was revealed that the adsorption process of CO2 on carrier CeOx is different from the conventionally assumed random adsorption model.52 The average particle size was estimated from the particle shape through STM, and more reliable data were obtained under the limit of larger metal loading, which better revealed the particle size effect and metal–support interactions in nanomaterials.53
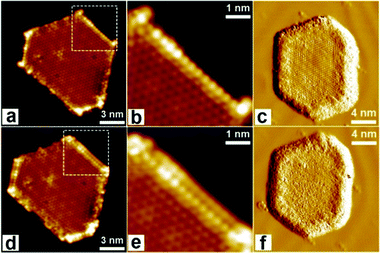 |
| Fig. 2 Interaction between CeOx/Au(111) and CO2. (a–d) STM images of the CeOx–Au(111) interface before (a and d) and after (b and e) CO2 adsorption at 78 K. The squared areas in (a and d) are magnified in (b and e), respectively. (c and f) Sequential STM images of the CeOx island upon extended CO2 exposure at 78 K. CO2 adsorbates propagate from the interface to the surface of CeOx, until the entire ceria terrace is covered with CO2. Scanning parameters: (b and e) Vs = 600 mV; It = 0.2 nA. Reprinted with permission.52 Copyright 2017, American Chemical Society. | |
3.1.2 High-angle annular dark-field scanning transmission electron microscopy (HAADF-STEM).
HAADF-STEM is a common visual way to preliminarily characterize the distribution state and distribution density of atoms on the substrate. In HAADF-STEM, the higher the Z value of a single atom, the more electrons are scattered at higher angles because of greater electrostatic interactions between the nucleus and the scanning electron beam. Therefore, the higher Z contrast of the target metal than the substrate results in more obvious images of the single atoms. The noble metal atoms that appear as bright dots can be ascribed to their much higher Z contrast than the oxide substrates. Meanwhile, due to the huge difference between the atomic weight of metal centers and carbon-based materials, metal single atoms can be clearly distinguished from carbon-based substrates.1
Lots of single-atom catalysts were explored deposited on carbon-based supports or metal oxides. In Fig. 3a, the single Pt atoms highlighted by red circles can be distinguished clearly from CeO2.34 Ir single atoms with the scale of 10 g (Fig. 3b) and 1000 g (Fig. 3c) loaded on CeO2 were also identified by HAADF-STEM, indicating that Ir species prepared at the 1000 g scale were still atomically dispersed over CeO2 with a similar structure of samples prepared at the 10 g scale.40 Meanwhile, single-atom catalysts with rare earth elements as active sites deposited on CN were characterized by HAADF-STEM. Fig. 3d and e are the images of high metal loaded Er atoms.28Fig. 3f displays the atomically dispersed Y on CN prepared by ball milling.6 In these figures, it is easy to distinguish the rare earth atomically dispersed.27
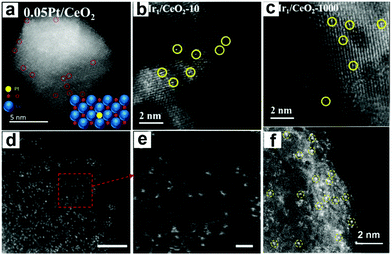 |
| Fig. 3 HAADF-STEM images of single-atom catalysts. (a) HAADF-STEM image of single atomic 0.05 Pt/CeO2. Reprinted with permission.34 Copyright 2018, American Chemical Society. (b and c) Images of atomically dispersed Ir atoms with different scales deposited on CeO2. Reprinted with permission.40 Copyright 2020, Elsevier B.V. HAADF-STEM image (d) and enlarged images (e) of Er1/CN-NT (carbon nitride nanotubes). Reproduced with permission.28 Copyright 2020, Wiley-VCH. (f) HAADF-STEM image of Y1/NC. Reprinted with permission.27 Copyright 2020, American Chemical Society. | |
3.2 Characterization techniques to provide indirect evidence
3.2.1 X-Ray absorption spectroscopy (XAS).
X-Ray absorption spectroscopy (XAS) is the measurement of transitions from the core electronic states of a metal to the excited electronic states and the continuum, which can be divided into X-ray absorption near-edge structure (XANES) and X-ray absorption fine structure (EXAFS) according to the energy range of spectroscopy. XANES can provide the average oxidation states of active sites and a further sophisticated data fitting process for EXAFS can acquire the structural parameters including the bond length and coordination structure. XAS is a unique technique that can detect the interactions between metal sites and supports, and is advantageous to explore the coordination of single atoms with the substrate in single-atom catalysts.
In Li's report, Fe single atoms with the coexistence of Fe2+ and Fe3+ were identified from XANES results, since their absorption edge is located between that of standard FeO and Fe2O3 (Fig. 4a). The EXAFS curve of Ce/Fe NCNW shows that Fe exists as dispersed and isolated atoms without Fe–Fe scattering peaks at ∼2.1 Å (Fig. 4b).37 Meanwhile, in Chen's report, the atomically dispersed sate and coordination enviroment of La sites were identified based on the analysis of k3-weighted EXAFS at the La LIII edge (Fig. 4c).54 Ce single atoms deposited on microporous N-doped carbon were also characterized by XAS. Ce L3-edge XANES spectroscopy and the corresponding spectra of standard samples are compared in Fig. 4d. Ce SAS/HPNC shows that the maximum peak is similar to Ce3+ rather than Ce4+, which shows two separated peaks with relatively low intensity. The Fourier-transformed (FT) EXAFS analysis of Ce SAS/HPNC is displayed in Fig. 4e. The broad peak located at 2.09 Å is assigned to Ce–N/O. The FT-EXAFS fitting curve of the Ce SAS/HPNC and proposed architectures are shown in Fig. 4f and the proposed architecture is Ce–N4/O6.29
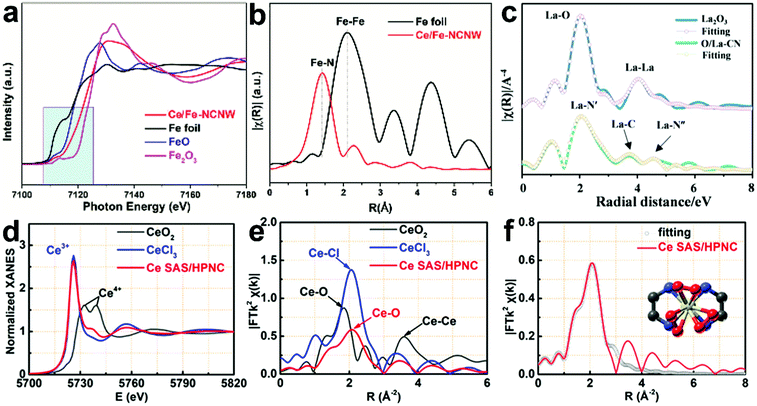 |
| Fig. 4 (a) Fe K-edge XANES and (b) Fourier-transform EXAFS spectra of Ce/Fe NCNW (N-doped carbon) nanowire and reference samples. Reprinted with permission.37 Copyright 2020, American Chemical Society. (c) The normalized XANES spectra at the La L3 edge of La2O3 and O/La-CN. Reprinted with permission.54 Copyright 2020, American Chemical Society (d) XANES of Ce SAS/HPNC (hierarchically macro–meso–microporous N-doped carbon), CeCl3, and CeO2 at the Ce L3-edge. (e) Fourier-transformed extended X-ray absorption fine structure (FT-EXAFS) spectra of Ce SAS/HPNC, CeCl3, and CeO2 at the Ce L3-edge. (f) FT-EXAFS fitting curve of the sample and proposed architectures of Ce–N4/O6. Reprinted with permission.29 Copyright 2020, American Chemical Society. | |
3.2.2 Other characterization techniques.
In addition to the methods stated above, diffuse reflectance infrared Fourier transform spectroscopy (DRIFTS) and temperature programmed reduction (TPR) are also used to prove the existence of a single atom, to gain surface information and for the recognition of single atom active sites.
DRIFTS is an infrared spectroscopy technique, in which the diffusely reflected light collected from the catalyst surface carries information about both the absorption of the surface layer and the scattering properties. In situ and ex situ DRIFTS techniques have been used to gain insight into the local geometry, reactivity, stability and homogeneity of the active species.55–57 CO is often used as a probe molecule in DRIFTS studies because it possesses several advantages in the characterization of exposed noble metal sites on supported catalysts.58In situ DRIFTS is a convincing characterization technique that could be applied to discriminate the different species and monitor the variation of Pt species in the reaction. For example, Wang et al. identified the single-atom Pt1/CeO2 catalyst and a sample of CeO2-supported Pt nanoparticles through DRIFTS (Fig. 5a). On Pt1/CeO2, only a peak at 2089 cm−1 was observed, which is very close to the value on isolated Pt atoms, and there was a blue shift of 15 cm−1 compared with the linear CO on Pt nanoparticles (2074 cm−1).44 In addition, Tan et al. identified the active sites through in situ DRIFTS of CO adsorption and subsequent oxidation. As shown in Fig. 5b, after activation, in addition to the bands at ca. 2100 cm−1 (CO on Pt single sites), two distinctive bands were observed, which could be assigned to CO adsorbed on ionic Pt at the interface. These two bands were consumed gradually with the introduction of O2 and experienced minimal further change after 10 min on stream, indicating that both sites were active in CO oxidation.59
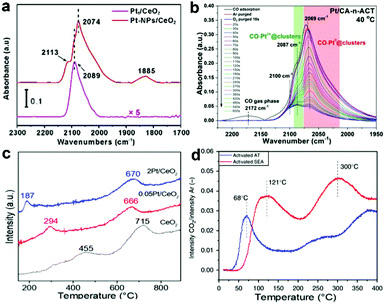 |
| Fig. 5 (a) DRIFTS of CO chemisorption on Pt1/CeO2 and Pt-NPs/CeO2. Reprinted with permission.44 Copyright 2017, American Chemical Society. (b) CO adsorption and reaction between O2 and adsorbed CO monitored by in situ DRIFTS on Pt/CA-n-ACT (40 °C). Reproduced with permission.59 Copyright 2020, Wiley-VCH. (c) H2-TPR profiles of neat CeO2 and Pt/CeO2 catalysts. Reprinted with permission.34 Copyright 2020, American Chemical Society. (d) CO-TPR of 1 wt% Pt/CeO2 catalysts. The reducibility of the catalysts synthesized by AT and SEA methods was monitored by observing the formation of CO2. Reproduced with permission.60 Copyright 2019 Nature Publishing Group. | |
Hydrogen temperature-programmed reduction (H2-TPR) or CO-TPR is usually used to analyse the reducibility and adsorption capacity of single-atom Pt catalysts. Amal et al. studied the reducibility of the as-obtained CeO2 and Pt/CeO2 samples by H2-TPR. Upon loading single-atom Pt onto CeO2, reduction peaks shifted to a lower temperature, which illustrated the promotional effect of single-atom Pt on the reducibility of CeO2 (Fig. 5c).34 Besides, Pereira-Hernández et al. further confirmed the facile reactivity of surface oxygen by the lower reduction peak during CO-TPR for the catalysts (Fig. 5d).60
3.3 Perspectives on the characterization of RE-SACs
Although some emerging advanced characterization techniques have been used for single-atom catalysts, there are still some limitations. A recent report by Zhong and his colleagues pointed out that EXAFS cannot effectively distinguish between tiny oxide clusters (2.8 nm) and single atoms.61 And the interaction between substrates and metal active sites is not clear enough. However, the combination of microscopic, spectroscopic, and other techniques can explicitly resolve the atomic structures.1 This will be a good assistance for studying the mechanism of the reactions. Meanwhile, some in situ techniques should be applied to help in monitoring and understanding the change of catalysts and its effect on reaction pathways under specific reaction conditions.
4. Application of RE-SACs
Using rare earth single-atom sites in various reactions is the most efficient way for the effective utilization of rare earth resources. So far, there have been relatively few studies on rare earth elements as the active centres, mainly focusing on photocatalytic or electrocatalytic reactions. Rare earth single sites play indispensable roles in these processes.
4.1 Photocatalytic reactions
Rare earth elements especially La and Er have been reported as active sites for photocatalytic reduction recently, showing outstanding performance. In Ji's report, single atomic Er sites were obtained and demonstrated outstanding photocatalytic CO2 reduction performance in the pure water system for the first time. Under light conditions, the high loading single atomic Er catalyst (HD-Er1/CN-NT) exhibits more CH4 and CO yields compared with the low loading single atomic Er catalyst (LD-Er1/CN-NT) and CN-NT. The yields of CO and CH4 also show a good linear relationship with time (Fig. 6a and b). Fig. 6c shows the calculated free energy diagram of the reduction of CO2 to CO and CH4. During the CO2 reduction process, the hydrocarbons go through CO. The formation of CH4 is limited by the reduction of CH3O to CH4 and O with a theoretical limiting potential of 0.39 V, which is more difficult than adsorption of CO (0.30 V). This is in good agreement with the experiments.28
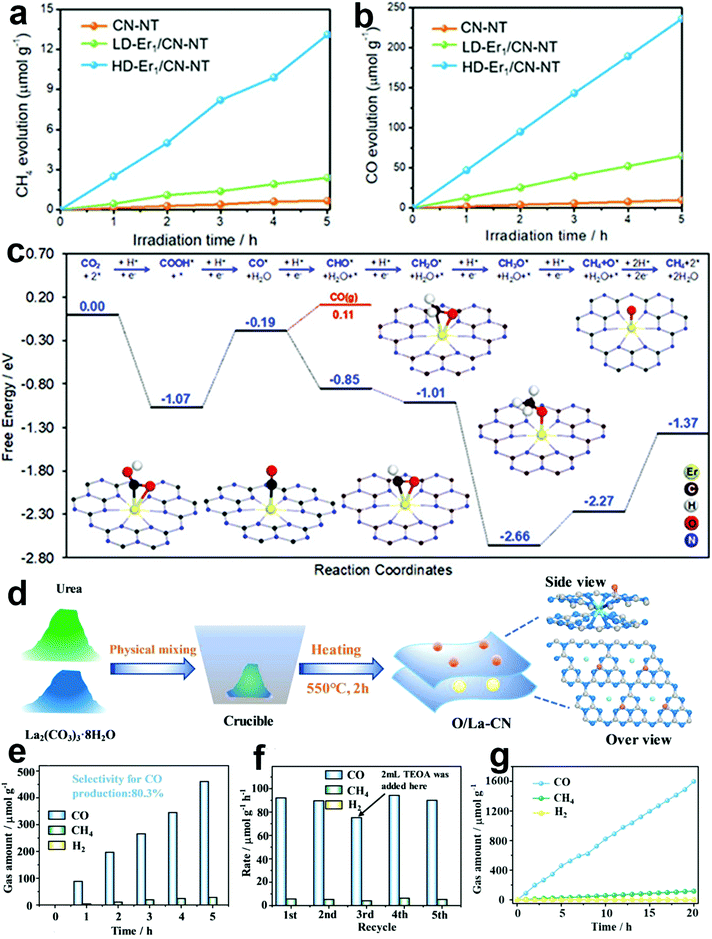 |
| Fig. 6 Rare earth element as active sites in photocatalysis. (a and b) Photocatalytic CO2 reduction performance of high and low loading single atomic Er catalysts. (c) The calculated free energy diagram of the reduction of CO2 to CO and CH4 and the corresponding adsorption configuration of the Er1/CN-NT catalyst and key intermediates. Reproduced with permission.28 Copyright 2020, Wiley-VCH. (d) Schematic diagram of the catalyst synthesis process. (e) Photocatalytic CO2 reduction performance of the single-atom La catalyst. Cycling experiments (f) and stability experiment (g) of the sample. Reprinted with permission.54 Copyright 2020, American Chemical Society. | |
The preparation process of single-atom La catalysts is illustrated in Fig. 6d, which were obtained by ball milling and calcination. O/La-CN shows a high CO selectivity of 80.3% and little CH4 and H2 was detected (Fig. 6e). After the 5th cycle, no obvious reduction in CO production was observed (Fig. 6f). The long-time catalytic test of 20 h also shows a fine linear relationship with time. Few CH4 and H2 were detected even after 20 h (Fig. 6g). The formed La–N charge bridge for the transfer of electrons to the surface is essential for CO2 activation, COOH* formation and CO desorption.54
On the other hand, rare earth elements combined with some traditional photocatalysts can yield some unexpected results. In Han's report, a novel photocatalyst through rare-earth single-atom bridging g-C3N4 and Zn2GeO4 shows high performance for CO2 reduction. This is attributed to the Er atoms acting as electron transport bridges in the interface, facilitating charge separation, and as CO2 molecular activation centers.62 In Chen's research, introducing single La and Er sites can provide additional adsorption sites and simplify the reaction route in the photodegradation of gaseous O-xylene, which expanded the application range of rare earth single atoms.63
4.2 Electrocatalytic reactions
Rare earth single atomic sites also play important roles in some electrocatalytic reactions. Ce based electrocatalysts have been explored in various reactions including HER, OER, NRR and CO2RR.64 Single-atom Ce species reveal excellent ORR performances in acid (0.1 M HClO4) and basic electrolytes (0.1 M KOH). In Zhu's report, Ce single atoms deposited on meso–microporous N-doped carbon (Ce SAS/HPNC) were prepared. As shown in Fig. 7a, ORR performances were tested in 0.1 M HClO4 at a scan rate of 10 mV s−1. Ce SAS/HPNC shows higher ORR activity than referenced catalysts. Specifically, Ce SAS/HPNC exhibits an onset potential (Eonset) of 1.04 V, a half-wave potential (E1/2) of 0.862 V, and a kinetic current density (K) at 0.9 V of 2.673 mA cm−2, as shown in Fig. 7b. The reaction mechanism for ORR is proposed in Fig. 7c. The authors also used the first principles calculations to calculate the ORR on the surface of the catalyst. The influence of voltage for free energy diagrams is displayed in Fig. 7d. With the increase of potential, free-energy change from *O2 to *OOH becomes positive first. The free energy diagrams for the reduction of O2 are shown in Fig. 7e.29 Meanwhile, in Li's report, Ce single sites deposited on carbon nanowires show high ORR properties with an E1/2 of 0.88 V in alkaline media and 0.75 V in acidic electrolytes (Fig. 7f). Fig. 7g indicates that this single-atom catalyst has high selectivity for the 4e− process. Gibbs free energy diagrams of different catalysts shown in Fig. 7h indicate SA Ce-N/PC with comparable performance to single-atom Fe catalysts. The corresponding reaction mechanism is also proposed as shown in Fig. 7i.36 The RE-SACs also show high activity in electrochemical N2 reduction and CO2 reduction. In Liu's report, Y/NC and Sc/NC show relatively high selectivity of NH3 and a high selectivity of CO at specific voltages (Fig. 7j and k).27 In general, single sites of La, Ce, Y, and Sc can play an important role in some electrocatalytic reactions. Therefore, exploring the role of rare earth single-atom sites in electrocatalytic reactions with more kinds of RE will be a promising research direction.
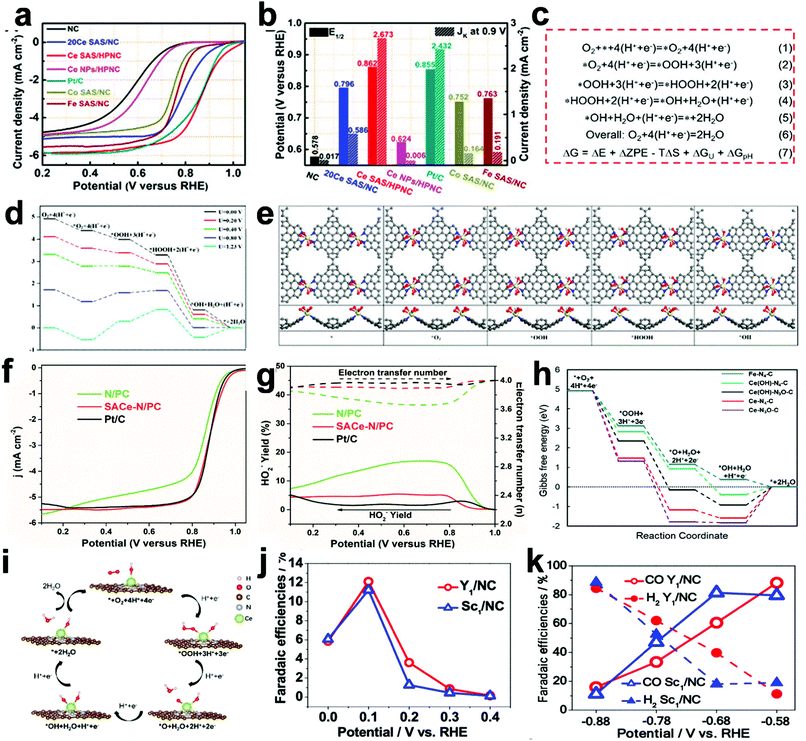 |
| Fig. 7 (a) LSV curves of the single atomic Ce catalyst and the corresponding samples in 0.1 M HClO4. (b) Comparison of E1/2 (half-wave potential) and JK (kinetic current density) of different catalysts. The calculation results based on the first principles calculations. (c) Proposed reaction mechanism for ORR. (d) The free-energy diagrams in different potentials. (e) The intermediate structure during the reaction process and the corresponding free-energy diagrams of O2 reduction. Reprinted with permission.29 Copyright 2020, American Chemical Society. (f) Disk current curves of single-atom Ce–N–C and other samples. (g) HO2− yields and electron transfer numbers. (h) Free-energy diagrams of different catalysts. (i) Reaction mechanism for ORR on single-atom Ce–N–C sites. Reprinted with permission.36 Copyright 2021, American Chemical Society. (j) NRR performances of single atomic Y1/NC and Sc1/NC. (k) CRR performances of Y/NC and Sc/NC. Reprinted with permission.27 Copyright 2020, American Chemical Society. | |
4.3 Perspectives on the applications of RE-SACs
From the above discussion, the RE-SACs have been successfully developed for some photo/electro-catalysis based energy conversion applications. Beyond this, other applications, such as thermal catalysis, photothermal catalysis, environmental decontamination, etc., are also promising to construct RE-SAC based material systems to meet the demand in customized reactions. For this, the rational design and accurate fabrication of RE-SACs are critical, deserving further effort in future.
5. Summary and outlook
In this mini-review, various synthetic and characterization methods, and applications in photo/electrocatalysis for rare earth based single-atom catalysts were summarized along with providing constructive discussion and future prospects, which is supposed to benefit researchers in this community. Up to now, the exploration of the special role of rich rare earth in the catalytic reaction is still not sufficient. Further development in the following aspects is envisioned.
(1) We think that there is a need for researchers to develop more promising synthetic methods of RE-SACs, especially large-scale preparation methods, which will benefit the practical application of rare earth based single-atom catalysts. The method based on TCPP proposed above will be highly feasible. Meanwhile, a series of ultra-thin colloidal nanostructures may become a very promising single-atom carrier for their distinctive advantages of large surface areas and conformal coating onto electrodes. In addition, surface ligands offer abundant bonding sites which provide another potential avenue for coordination of target single atoms. Last but not the least, the ultra-small confinement effect arising from the thickness of only a few atomic layers in a certain dimension may be beneficial to the formation of single atoms.
(2) Some in situ strategies should also be developed and applied in this field such as the operando X-ray absorption fine structure (XAFS) technique, in situ Fourier-transform infrared spectroscopy (FITR), etc. This will help to monitor and understand the reaction mechanism, reaction pathways and the changes of catalysts during reactions. These are critical to unveil the co-relationships among them and hence enable better co-support between theoretical design and experimentation.
(3) More application areas should be expanded beyond the above discussed, such as biological applications or some organic catalytic reactions. The unique 4f electron configurations endow rare earth elements with rich spectroscopic properties and excellent magnetic resonance imaging capability, which makes them attractive for bio-applications, including multimodal bioimaging, biosensing, imaging-guided therapy and monitoring of human health. Reducing rare earth to the atomic scale can further enlarge their spectroscopic properties and greatly enhance their potential in precise localization and synergistic treatment. At the same time, because of the high oxygen-affinity ability of rare earth metals, they can activate the carbonyl group by coordinating with the oxygen atom, making it easier to be attacked by the nucleophilic reagent to cause nucleophilic substitution reaction. However, the high oxygen-affinity of rare earth elements may induce the low stability of RE-SACs in ambient conditions and the activity decreases during long-time reactions, which is an important aspect to be considered and resolved when expanding the application areas.
(4) Finally, the emerging high throughput computation and experimentation can be applied in this field to accelerate the discovery and screening of RE-SAC based materials for various reactions. To assist with machine learning, which will provide constructive guidance and feedback based on the available and self-developed database, the fast, precise, intelligent research strategies as pursued by scientists will become highly promising in near future, which is believed to effectively drive advanced science and technology development from the proof-of-concepts in labs towards the practice in industry.
Conflicts of interest
There are no conflicts to declare.
Acknowledgements
Financial support from the National Natural Science Foundation of China (21971117), Functional Research Funds for the Central Universities, Nankai University (63186005), Tianjin Key Lab for Rare Earth Materials and Applications (ZB19500202), the Open Funds (No. RERU2019001) of the State Key Laboratory of Rare Earth Resource Utilization, the National Key R&D Program of China (No. 2017YFA0208000), 111 Project (No. B18030) from China, Beijing-Tianjin-Hebei Collaborative Innovation Project (19YFSLQY00030), the Outstanding Youth Project of Tianjin Natural Science Foundation (20JCJQJC00130), and the Key Project of Tianjin Natural Science Foundation (20JCZDJC00650) is acknowledged.
Notes and references
- Y. Wang, H. Su, Y. He, L. Li, S. Zhu, H. Shen, P. Xie, X. Fu, G. Zhou, C. Feng, D. Zhao, F. Xiao, X. Zhu, Y. Zeng, M. Shao, S. Chen, G. Wu, J. Zeng and C. Wang, Chem. Rev., 2020, 120, 12217–12314 CrossRef CAS PubMed.
- H. Lu, J. Tournet, K. Dastafkan, Y. Liu, Y. H. Ng, S. K. Karuturi, C. Zhao and Z. Yin, Chem. Rev., 2021, 102, 13517–13530 Search PubMed.
- L. Wang, W. Chen, D. Zhang, Y. Du, R. Amal, S. Qiao, J. Wu and Z. Yin, Chem. Soc. Rev., 2019, 48, 5310–5349 RSC.
- B. Qiao, A. Wang, X. Yang, L. F. Allard, Z. Jiang, Y. Cui, J. Liu, J. Li and T. Zhang, Nat. Chem., 2011, 3, 634–641 CrossRef CAS.
- K. Jiang, S. Siahrostami, T. Zheng, Y. Hu, S. Hwang, E. Stavitski, Y. Peng, J. Dynes, M. Gangisetty, D. Su, K. Attenkofer and H. Wang, Energy Environ. Sci., 2018, 11, 893–903 RSC.
- J. Du, G. Wu, K. Liang, J. Yang, Y. Zhang, Y. Lin, X. Zheng, Z. Q. Yu, Y. Wu and X. Hong, Small, 2021, 17, e2007264 CrossRef.
- H. Su, L. Chen, Y. Chen, R. Si, Y. Wu, X. Wu, Z. Geng, W. Zhang and J. Zeng, Angew. Chem., Int. Ed., 2020, 59, 20411–20416 CrossRef CAS PubMed.
- L. Han, S. Song, M. Liu, S. Yao, Z. Liang, H. Cheng, Z. Ren, W. Liu, R. Lin, G. Qi, X. Liu, Q. Wu, J. Luo and H. L. Xin, J. Am. Chem. Soc., 2020, 142, 12563–12567 CrossRef CAS.
- F. Yang, P. Song, X. Liu, B. Mei, W. Xing, Z. Jiang, L. Gu and W. Xu, Angew. Chem., Int. Ed., 2018, 57, 12303–12307 CrossRef CAS.
- P. Lu, X. Tan, H. Zhao, Q. Xiang, K. Liu, X. Zhao, X. Yin, X. Li, X. Hai, S. Xi, A. T. S. Wee, S. J. Pennycook, X. Yu, M. Yuan, J. Wu, G. Zhang, S. C. Smith and Z. Yin, ACS Nano, 2021, 15, 5671–5678 CrossRef CAS.
- E. A. Derevyannikova, T. Y. Kardash, A. I. Stadnichenko, O. A. Stonkus, E. M. Slavinskaya, V. A. Svetlichnyi and A. I. Boronin, J. Phys. Chem. C, 2018, 123, 1320–1334 CrossRef.
- J. Chen, Y. Wanyan, J. Zeng, H. Fang, Z. Li, Y. Dong, R. Qin, C. Wu, D. Liu, M. Wang, Q. Kuang, Z. Xie and L. Zheng, ACS Sustainable Chem. Eng., 2018, 6, 14054–14062 CrossRef CAS.
- H. Wang, J. X. Liu, L. F. Allard, S. Lee, J. Liu, H. Li, J. Wang, J. Wang, S. H. Oh, W. Li, M. Flytzani-Stephanopoulos, M. Shen, B. R. Goldsmith and M. Yang, Nat. Commun., 2019, 10, 3808 CrossRef.
- M. Yoo, Y.-S. Yu, H. Ha, S. Lee, J.-S. Choi, S. Oh, E. Kang, H. Choi, H. An, K.-S. Lee, J. Y. Park, R. Celestre, M. A. Marcus, K. Nowrouzi, D. Taube, D. A. Shapiro, W. Jung, C. Kim and H. Y. Kim, Energy Environ. Sci., 2020, 13, 1231–1239 RSC.
- Y. Tang, Y.-G. Wang and J. Li, J. Phys. Chem. C, 2017, 121, 11281–11289 CrossRef CAS.
- Y. Feng, Q. Wan, H. Xiong, S. Zhou, X. Chen, X. I. Pereira Hernandez, Y. Wang, S. Lin, A. K. Datye and H. Guo, J. Phys. Chem. C, 2018, 122, 22460–22468 CrossRef CAS.
- J. Qi, L. Gao, F. Wei, Q. Wan and S. Lin, ACS Appl. Mater. Interfaces, 2019, 11, 47525–47534 CrossRef CAS.
- J. Li, Z. Liu, D. A. Cullen, W. Hu, J. Huang, L. Yao, Z. Peng, P. Liao and R. Wang, ACS Catal., 2019, 9, 11088–11103 CrossRef CAS.
- H. Xu, Z. Zhang, J. Liu, C. L. Do-Thanh, H. Chen, S. Xu, Q. Lin, Y. Jiao, J. Wang, Y. Wang, Y. Chen and S. Dai, Nat. Commun., 2020, 11, 3908 CrossRef CAS.
- F. He, H. Li, Y. Ding, K. Li, Y. Wang and Z. Wu, Carbon, 2018, 130, 636–644 CrossRef CAS.
- J. Xia, H. Zhao, W. K. Pang, Z. Yin, B. Zhou, G. He, Z. Guo and Y. Du, Chem. Sci., 2018, 9, 3421–3425 RSC.
- J. Wang, X. Sun, L. Xu, J. Xia, Y. Yang, Z. Yin, F. Luo and Y. Du, Adv. Mater. Interfaces, 2020, 7 Search PubMed.
- S. Zhang, S. E. Saji, Z. Yin, H. Zhang, Y. Du and C. Yan, Adv. Mater., 2021, 33, e2005988 CrossRef PubMed.
- Z. Zeng, Y. Xu, Z. Zhang, Z. Gao, M. Luo, Z. Yin, C. Zhang, J. Xu, B. Huang, F. Luo, Y. Du and C. Yan, Chem. Soc. Rev., 2020, 49, 1109–1143 RSC.
- Z. Liu, N. Li, H. Zhao, Y. Zhang, Y. Huang, Z. Yin and Y. Du, Chem. Sci., 2017, 8, 3211–3217 RSC.
- J.-F. Sun, Q.-Q. Xu, J.-L. Qi, D. Zhou, H.-Y. Zhu and J.-Z. Yin, ACS Sustainable Chem. Eng., 2020, 8, 14630–14656 CrossRef CAS.
- J. Liu, X. Kong, L. Zheng, X. Guo, X. Liu and J. Shui, ACS Nano, 2020, 14, 1093–1101 CrossRef CAS.
- S. Ji, Y. Qu, T. Wang, Y. Chen, G. Wang, X. Li, J. Dong, Q. Chen, W. Zhang, Z. Zhang, S. Liang, R. Yu, Y. Wang, D. Wang and Y. Li, Angew. Chem., Int. Ed., 2020, 59, 10651–10657 CrossRef CAS PubMed.
- M. Zhu, C. Zhao, X. Liu, X. Wang, F. Zhou, J. Wang, Y. Hu, Y. Zhao, T. Yao, L.-M. Yang and Y. Wu, ACS Catal., 2021, 11, 3923–3929 CrossRef CAS.
- M. Babucci, A. Guntida and B. C. Gates, Chem. Rev., 2020, 120, 11956–11985 CrossRef CAS PubMed.
- S. Ji, Y. Chen, X. Wang, Z. Zhang, D. Wang and Y. Li, Chem. Rev., 2020, 120, 11900–11955 CrossRef CAS.
- N. Zhang, H. Yan, L. Li, R. Wu, L. Song, G. Zhang, W. Liang and H. He, J. Rare Earths, 2021, 39, 233–242 CrossRef CAS.
-
D. S. Cotton, Lanthanide and Actinide Chemistry, John Wiley & Sons Ltd, Chichester, 2006 Search PubMed.
- Y. Wang, H. Arandiyan, J. Scott, K.-F. Aguey-Zinsou and R. Amal, ACS Appl. Energy Mater., 2018, 1, 6781–6789 CrossRef CAS.
- D. Jiang, G. Wan, C. E. García-Vargas, L. Li, X. I. Pereira-Hernández, C. Wang and Y. Wang, ACS Catal., 2020, 10, 11356–11364 CrossRef CAS.
- J. C. Li, X. Qin, F. Xiao, C. Liang, M. Xu, Y. Meng, E. Sarnello, L. Fang, T. Li, S. Ding, Z. Lyu, S. Zhu, X. Pan, P. X. Hou, C. Liu, Y. Lin and M. Shao, Nano Lett., 2021, 21, 4508–4515 CrossRef CAS.
- J.-C. Li, S. Maurya, Y. S. Kim, T. Li, L. Wang, Q. Shi, D. Liu, S. Feng, Y. Lin and M. Shao, ACS Catal., 2020, 10, 2452–2458 CrossRef CAS.
- Q. Liu, Y. Li, L. Zheng, J. Shang, X. Liu, R. Yu and J. Shui, Adv. Energy Mater., 2020, 10, 2000689 CrossRef CAS.
- Q. Liu, X. Liu, L. Zheng and J. Shui, Angew. Chem., Int. Ed., 2018, 57, 1204–1208 CrossRef CAS PubMed.
- T. Gan, Q. He, H. Zhang, H. Xiao, Y. Liu, Y. Zhang, X. He and H. Ji, Chem. Eng. J., 2020, 389, 124490 CrossRef CAS.
- Y. Wang, Z. Chen, P. Han, Y. Du, Z. Gu, X. Xu and G. Zheng, ACS Catal., 2018, 8, 7113–7119 CrossRef CAS.
- X. Ye, H. Wang, Y. Lin, X. Liu, L. Cao, J. Gu and J. Lu, Nano Res., 2019, 12, 1401–1409 CrossRef CAS.
- H. Yan, H. Cheng, H. Yi, Y. Lin, T. Yao, C. Wang, J. Li, S. Wei and J. Lu, J. Am. Chem. Soc., 2015, 137, 10484–10487 CrossRef CAS.
- C. Wang, X.-K. Gu, H. Yan, Y. Lin, J. Li, D. Liu, W.-X. Li and J. Lu, ACS Catal., 2016, 7, 887–891 CrossRef.
- Z. Liang, L. Song, M. Sun, B. Huang and Y. Du, Sci. Adv., 2021, 7, eabl4915 CrossRef.
- R. Lang, X. Du, Y. Huang, X. Jiang, Q. Zhang, Y. Guo, K. Liu, B. Qiao, A. Wang and T. Zhang, Chem. Rev., 2020, 120, 11986–12043 CrossRef CAS PubMed.
- L. Jiao, G. Wan, R. Zhang, H. Zhou, S. H. Yu and H. L. Jiang, Angew. Chem., Int. Ed., 2018, 57, 8525–8529 CrossRef CAS.
- Q. Zuo, T. Liu, C. Chen, Y. Ji, X. Gong, Y. Mai and Y. Zhou, Angew. Chem., Int. Ed., 2019, 58, 10198–10203 CrossRef CAS.
- Y. Chen, S. Ji, C. Chen, Q. Peng, D. Wang and Y. Li, Joule, 2018, 2, 1242–1264 CrossRef CAS.
- K. Morgenstern, N. Lorente and K.-H. Rieder, Phys. Status Solidi B, 2013, 250, 1671–1751 CrossRef CAS.
- Q. Liu and Z. Zhang, Catal. Sci. Technol., 2019, 9, 4821–4834 RSC.
- D. Gao, Y. Zhang, Z. Zhou, F. Cai, X. Zhao, W. Huang, Y. Li, J. Zhu, P. Liu, F. Yang, G. Wang and X. Bao, J. Am. Chem. Soc., 2017, 139, 5652–5655 CrossRef CAS.
- Y. Lykhach, S. M. Kozlov, T. Skala, A. Tovt, V. Stetsovych, N. Tsud, F. Dvorak, V. Johanek, A. Neitzel, J. Myslivecek, S. Fabris, V. Matolin, K. M. Neyman and J. Libuda, Nat. Mater., 2016, 15, 284–288 CrossRef CAS.
- P. Chen, B. Lei, X. Dong, H. Wang, J. Sheng, W. Cui, J. Li, Y. Sun, Z. Wang and F. Dong, ACS Nano, 2020, 14, 15841–15852 CrossRef PubMed.
- J. A. Rodriguez, D. C. Grinter, Z. Liu, R. M. Palomino and S. D. Senanayake, Chem. Soc. Rev., 2017, 46, 1824–1841 RSC.
- Q. Zhang, X.-X. Qin, F.-P. Duan-Mu, H.-M. Ji, Z.-R. Shen, X.-P. Han and W.-B. Hu, Angew. Chem., Int. Ed., 2018, 57, 9351–9356 CrossRef CAS.
- K. Yang, Y. Liu, J. Deng, X. Zhao, J. Yang, Z. Han, Z. Hou and H. Dai, Appl. Catal., B, 2019, 244, 650–659 CrossRef CAS.
- K. Ding, A. Gulec, A. M. Johnson, N. M. Schweitzer, G. D. Stucky, L. D. Marks and P. C. Stair, Science, 2015, 350, 189–192 CrossRef CAS.
- W. Tan, H. Alsenani, S. Xie, Y. Cai, P. Xu, A. Liu, J. Ji, F. Gao, L. Dong, E. Chukwu, M. Yang and F. Liu, ChemNanoMat, 2020, 6, 1797–1805 CrossRef CAS.
- X. I. Pcrcira-Hcrnandcz, A. DeLaRiva, V. Muravev, D. Kunwar, H. Xiong, B. Sudduth, M. Engelhard, L. Kovarlk, E. J. M. Hcnscn, Y. Wang and A. K. Datye, Nat. Commun., 2019, 10, 1358 CrossRef.
- K. Feng, H. Zhang, J. Gao, J. Xu, Y. Dong, Z. Kang and J. Zhong, Appl. Phys. Lett., 2020, 116, 191903 CrossRef CAS.
- Z. Han, Y. Zhao, G. Gao, W. Zhang, Y. Qu, H. Zhu, P. Zhu and G. Wang, Small, 2021, 17, e2102089 CrossRef.
- J. Chen, L. Chen, X. Wang, Z. Rao, J. Sun, A. Chen and X. Xie, J. Colloid Interface Sci., 2021, 605, 674–684 CrossRef PubMed.
- Q. Li, L. Song, Z. Liang, M. Sun, T. Wu, B. Huang, F. Luo, Y. Du and C. Yan, Adv. Energy Sustainability Res., 2021, 2, 2000063 CrossRef.
|
This journal is © The Royal Society of Chemistry 2022 |
Click here to see how this site uses Cookies. View our privacy policy here.