DOI:
10.1039/C7RA05468H
(Review Article)
RSC Adv., 2017,
7, 37887-37897
Recent advances in the rational design of copper chalcogenide to enhance the photothermal conversion efficiency for the photothermal ablation of cancer cells
Received
15th May 2017
, Accepted 17th July 2017
First published on 1st August 2017
Abstract
Copper chalcogenide nanoparticles have received increased attention as photothermal agents due to their low cost and easy preparation. Nevertheless, the underdeveloped photothermal conversion efficiency of copper chalcogenide has limited its further application for cancer therapy. Thus, enhancing the photothermal conversion efficiency of copper chalcogenide through rational design is desired. In this review, we have discussed three kinds of rational design and a mechanism for copper chalcogenide to enhance the heat conversion efficiency in detail and briefly introduced their application in photothermal therapy. First is the design and synthesis of the superstructure nanoparticle agents to enhance photon refraction. Then, tuning the degree of copper deficiency enhances the localized surface plasmon resonance absorption caused by copper chalcogenide. The rest is constructing the hybrid composite to further enhance the localized surface plasmon resonance absorption through the coupling effect of different functional units. Despite higher photothermal conversion efficiencies and better therapeutic effects for cancer achieved through rational design, there still exists a challenge to be addressed, as well as promise for rational design facing the keen research and discuss before clinical applications of copper chalcogenide.
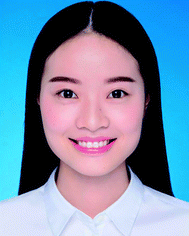 Chenglin Yan | Chenglin Yan received her BE degree from Shanghai Normal University in 2012. She is now a master's degree candidate in Prof. Shiping Yang's group. Her current research directions include nanomaterial-based cancer detection and photothermal therapy. |
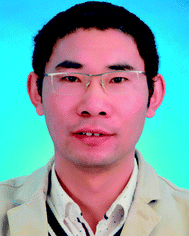 Qiwei Tian | Qiwei Tian obtained his Ph.D. degree from materials science, Donghua University, China, in 2012, and worked as a postdoctoral fellow at King Abdullah University of Science and Technology during 2012–2015. He is currently working as an associate professor in Shanghai Normal University and is focused on developing multi-functional nanomaterials for cancer theranostics. |
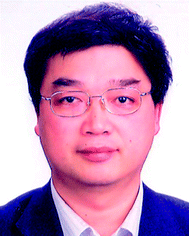 Shiping Yang | Shiping Yang is a Professor of the Key Laboratory of Resource Chemistry of Ministry of Education, Shanghai Normal University, P. R. China. He received his PhD in Sun Yat-Sen University, P. R. China in 2000. His research interest is in the fields of biomedical applications of nanomaterials for novel biomedical imaging and cancer therapy. |
1. Introduction
As we all know, cancer and malignant tumor are the leading causes of death worldwide.1 Over the past few decades, nanotechnology for the detection,2,3 diagnosis4,5 and treatment of cancer6 and other diseases, has undergone rapid development and innovation. Along with the improvement of the therapeutic progress and prospect, significant efforts have been devoted to exploring the improved therapy method,7–9 expecting to achieve better therapeutic outcomes and minimal side effects or physical pain towards the ultimate aim. Thus, except for traditional chemotherapy, photothermal ablation therapy has captured increasing attention, owing to its minimally invasive nature.10
Specifically, photothermal ablation therapy, induced by laser in the near-infrared (NIR 700–1100 nm) region,11,12 gradually holds an important status in the nanotechnology therapy field because of the low scattering and small absorption of NIR light by tissue and blood and the ability to reach several centimeters inside biological tissues.13,14 Subsequently, a key determinant in the applications of photothermal ablation therapy is exactly the exploitation of novel photothermal agents, which possess attractive advantages such as strong NIR absorption ability and/or excellent photothermal conversion efficiency (PTCE).15–17 Generally, photothermal agents will produce the photothermal coupling effect, which gives rise to the absorption of NIR light, and then results in the transformation to thermal energies that will burn out nearby cancerous cells.18 To paraphrase the great importance of photothermal agents, higher PTCE will equally induce the death of cancerous cells with shorter irradiation time, smaller concentration of nanoparticles (NPs), or lower power density of the NIR laser to ensure the safety of healthy tissues in the human body.19
Copper chalcogenide is a p-type semiconductor and has been widely explored in energy,20–23 catalysis-related applications,24,25 and most especially in tumor theranostics.26–29 Compared with carbon,30–33 noble metal,34–36 and organic materials,37–40 the merits of using copper chalcogenide NPs in the diagnosis and therapy of disease include not only the low cost and toxicity, but also the intrinsic strong NIR absorption, rendering a promising alternative for photothermal ablation of cancerous cells.41,42 However, it is noted that the unsatisfactory PTCE limits their applications in photothermal therapy to a large extent;43 for instance, CuS NPs with an ultrasmall size of ∼3 nm require increased laser power intensity or particle concentration to obtain the same therapeutic result due to the low PTCE.43 According to the Safe Use of Lasers of the American National Standard, to cause sufficient death of cancerous cells, the excessively small CuS NPs require as high as 16 and 24 W cm−2, which is ∼48 and 72 times higher than the conservative limit (∼0.33 W cm−2) of the laser intensity setting for human skin exposure.44,45 Thus, it is important to design and synthesize copper chalcogenide NPs which are capable of enhancing PTCE particularly in the photothermal therapeutic field.
As usual, for the simple purpose of enhancing PTCE, three routes of designing copper sulfide NPs can be classified. The first is tuning the structure of the isolated nanoparticle to enhance photon refraction, coupling with stronger NIR absorption. Or by changing the synthesis condition to regulate the isolated nanoparticle to assemble into a different structure, leading to enhanced NIR absorption. The second is synthesizing non-stoichiometric copper chalcogenide NPs. And the rest is constructing the composite bimetallic nanomaterial. In this short review, we will provide an overview of the chemical mechanism of rational design for copper chalcogenide to enhance the heat conversion efficiency and their biomedical application for photothermal ablation of cancerous cells.
2. Typical synthetic procedures for copper chalcogenide
In general, it is paramount to properly manage the morphology, size and phase of NPs, which play vital roles in their optical and electronic properties.46 Specially, the absorption of copper chalcogenide is more dependent on their size, morphology and phase.47 In the past few years, several methods, such as hydrothermal method and solvothermal method,48 thermal decomposition method19 and hard template method,49 have been developed to control size, morphology and phase of copper chalcogenide. And we will introduce how to use the three main synthesis approaches for copper chalcogenide in this section.
2.1 Hydrothermal method and solvothermal method
Hydrothermal method50 and solvothermal method51 are the two most frequently used preparation techniques in the synthesis of copper chalcogenide NPs due to their high efficiency and simplicity. They are both synthesized in a high pressure reactor, the only difference is that the former uses an aqueous solvent, and the latter uses a non-aqueous organic compound as the solvent in the process of reaction.52 For these two reaction methods, it is easier to control the growth direction and morphology of nanocrystals by simply tuning the temperature, reaction time and surfactant. At present, a variety of copper chalcogenide nanocrystals with different morphology have been obtained by these two methods. The flower-like CuS was synthesized by our group through the hydrothermal method using PVP as the surfactant.48 In the study of Zhao et al.,53 nanowires, nanotubes and nano-sized vesicles of copper sulfide were obtained by hanging the copper ligand at a lower temperature (90 °C to 110 °C) with an organic amine-assisted. High-quality isolated crystal Cu2−xSe nanosheets and nanodisks were prepared by Zhang et al.54 through a green and simple solvothermal method using paraffin oil as a solvent. For solvothermal and hydrothermal methods, they are relatively simple processes, and more importantly, the nanocrystals obtained by these two methods have good crystal structure and different morphologies of nanocrystals can be attained by only changing part of the reaction conditions. However, large size and wide size distribution19 of copper chalcogenide synthesized by both methods limit their future biomedical application.
2.2 Thermal decomposition method
The thermal decomposition method is based on copper precursor, X = S, Se, Te precursor, and long-chain hydrocarbon compounds (oleic acid, oleylamine, octadecene and so on) as the surfactant and solvent, using the metal organic chemistry mechanism to prepare chalcopyrite nanocrystals under the condition of anaerobic water and high temperature (T > 200 °C).55–57 And subsequently, by instantaneous nucleation under high temperature condition and further heat treatment process, copper chalcogenide nanocrystals with good crystallinity and homogeneous dimension are obtained. The Kolny-Olesiak group were able to synthesis uniform, high-quality Cu2S nanorods with octadecene as a solvent in the process of pyrolysis, and by controlling the nucleation temperature, they achieved nanorods with different radial ratios.58 Due to faster growth speed of the crystal nucleus at the higher temperature, more lattice imperfections will be formed. Thus, most of the copper chalcogenide nanocrystals achieved by the thermal decomposition method are non-stoichiometric (Cu2−xS) nanocrystals with deficiency, which brings about localized surface plasmon resonances (LSPRs) that resulted in tunable NIR absorption. Various shapes of Cu2−xS nanocrystals, such as nanorods and nanosheets have been prepared by Korgel et al.59 Also in our previous studies, we succeeded to synthesize some kinds of non-stoichiometric copper chalcogenide with strong NIR absorption.19,60
2.3 Hard template method
The sacrificial templates method, in which the template is also used as the precursor, is an effective approach to synthesize the nanostructure materials,61,62 especially for the hybrid nanostructure. For copper chalcogenide NPs, it is difficult to prepare the hybrid nanostructure directly, due to the issue of large lattice mismatch.63 In this regard, the sacrificial template is frequently utilized to prepare the copper chalcogenide hybrid nanostructure. Based on the sacrificial templates, there are two different mechanisms, one is cation exchange63 and the other is the Kirkendall effect,64 which were developed to prepare the copper chalcogenide hybrid nanostructure. The cation exchange method,65 in which the cation located in the nanocrystal template is substituted by another one in solution with the same template morphology, has been developed as a particularly powerful strategy to prepare nanocrystals with morphology and composition that are accessed difficultly by conventional methods. Depending on precise control of the stoichiometry over the reaction extent, the Au@Cu2−xS hybrid nanostructure with flexible shape, crystallization and composition tailoring was prepared by Zhang et al.,63 starting from the Au@CdS core–shell nanostructure (Fig. 1A). In the Kirkendall effect method66 the template, such as Cu2O, reacts with the new molecular or ionic species (S, Se, Te precursor) by atomic or ionic diffusion and then a new shape and composition is formed. Using Au@Cu2O as a hard template, Yu et al.49 and Zhang et al.67 have developed two different Au@Cu2−xS yolk–shell nanostructures (Fig. 1B).
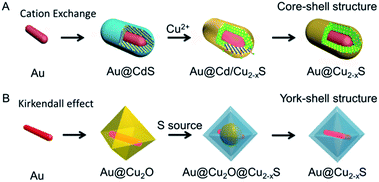 |
| Fig. 1 Scheme of synthesizing the Au–Cu2−xS hybrid based on the template method.49,63 (A) Reprinted with permission from ref. 63. Copyright 2016 Wiley-VCH Verlag & Co. KGaA. (B) Reprinted with permission from ref. 49. Copyright 2016 American Chemical Society. | |
3. Copper chalcogenide with enhanced PTCE for cancer therapy
Cytotoxicity limitation is a major concern for a novel well-designed nanomaterial, particularly in the application of biomedical and clinical treatments.43 To be specific, to achieve the same therapy outcome, a higher concentration of nanomaterial will result in more efficient photothermal therapy to treat cancer cells or tumor, as an increasing number of nanomaterial particles will be absorbed by cancerous cells. In the meantime, high cellular uptake signifies that the cells accumulate a larger amount of nanomaterial, which leads to increased cytotoxicity.35,68 Aimed at the above contradiction between cellular uptake of nanomaterial and photothermal ablation of cancerous cells or tumor, recently, some groups began to throw light on how to improve the PTCE of the nanomaterial and further minimize the shortcomings brought from this contradiction as far as possible. In this section, we will introduce three routes of design and discuss the mechanisms on how to enhance the PTCE through the well-designed copper sulfide NPs in detail, as well as briefly present their applications in the photothermal ablation of cancerous cells.
3.1 Nanostructure of copper chalcogenide
Complex superstructures with enhanced and synergistic property, which are composited with simple building blocks, have attracted widespread interest owing to their great potential applications in biomedicine,69 energy storage70 and catalysis.71 Up to now, two different kinds of mechanisms have been proposed for the superstructure to enhance the PTCE. One kind is the building blocks of the superstructure aligned with photonic-crystal microstructures and/or the faceted end planes of well-shaped crystals, showing their ability to be utilized for good laser-cavity mirrors, which helps to effectively improve light absorption.48 The other kind is the superstructures that greatly influence the electronic structures of nanomaterials and significantly improve the molar extinction coefficient in the NIR region.72 For the photothermal agents, the more light is absorbed the more heat will be generated. Thus, constructing a superstructure for CuS NPs is a simple and effective method to increase the obtained heat when using the same amount of nanomaterials. In conclusion, the design direction of constructing a special nanostructure could be divided into two types, the straightforward strategy of the synthesis of the superstructure and the particle-based assembly three-dimensional structure. Here, we would illustrate the absorption and therapeutic effect of the CuS superstructure, compared with the normal structure.
3.1.1 CuS superstructure as laser-cavity mirrors. Most of the obtained CuS superstructures are constructed by small building blocks with regular shapes. If the building blocks are aligned with photonic-crystal microstructures and/or the faceted end planes of well-shaped crystals, the synthesized CuS superstructures can be employed as good laser-cavity mirrors, resulting in the promotion of light absorption,73–75 which further enhances the PTCE and ultimately improves the therapeutic effects. As in our previous study,48 we have designed the novel hydrophilic flower-like CuS superstructures by a one pot hydrothermal route and then demonstrated that CuS superstructures can be employed as an efficient laser-driven photothermal agent, which is promising for the ablation of cancerous cells (Fig. 2A). Most importantly, compared with hexagonal nanoplates (their corresponding building blocks) (Fig. 2B), the as-synthesized CuS superstructure exhibited high absorption intensity especially in the NIR region, which was improved by 50% of magnitude through constructing photonic-crystal microstructures and/or laser-cavity mirrors that conduced to greatly elevate the absorption and reflection ability for laser, thus offering an improved PTCE as well. Further in our investigations, under the irradiation of a 980 nm laser with a conservative and safe power density of 0.51 W cm−2, CuS superstructures can efficiently kill cancer cells packaged by chicken skin (Fig. 2C) or in vivo with a short period (5–10 min), consequently showing the superior therapy outcome (Fig. 2D).
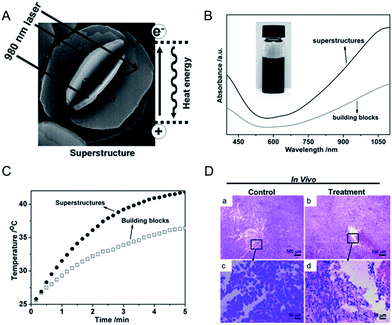 |
| Fig. 2 (A) Schematic representation of a CuS superstructure serving as laser-cavity mirrors for a 980 nm laser and its photothermal conversion. (B and C) The absorption and temperature elevation of the CuS superstructures and their building blocks, respectively. (D) The representative H & E stained histological images of ex vivo tumor sections treated by water (a and c) and CuS superstructure (b and d), respectively.48 Reprinted with permission from ref. 49. Copyright 2011 Wiley-VCH Verlag & Co. KGaA. | |
By a similar approach, Wu et al.76 reported the hollow and hierarchical CuInS2 microspheres, which were constructed separately from the sacrificial templates of solid and hollow CuS microspheres (Fig. 3). The as-synthesized hollow and hierarchical CuInS2 microspheres with close cavities and open cavities, respectively, presented a rosy photothermal conversion effect. To be specific, it is noted that an amount equal to 30% and 20% incremental of PTCE was achieved, respectively, mainly attributing to the approach of constructing a special nanostructure. Ground on this intense photothermal conversion effect of the hollow CuS originated from its unique optical property that displayed strong absorption in the NIR region, Samy Ramadan et al.77 testified that the permeability of human growth hormone can be increased through disrupting the skin by using the hollow CuS to mediate photothermal ablation. This technique has shown encouraging application in macromolecular drug and vaccine delivery. Therefore, designing a highly meaningful special nanostructure as photothermal conversion agents is supposedly a promising direction for enhancing the PTCE and is further being applied to photothermal ablation therapy.
 |
| Fig. 3 (A) Schematic demonstration of photothermal conversion enhancement and temperature elevation based on the cavity-mirror effect and interband transitions. (B) Temperature elevation of water, PBS and different superstructures under laser irradiation.76 Reprinted with permission from ref. 76. Copyright 2013 American Chemical Society. | |
By assembly of oppositely charged CuS NPs and then calcination at elevated temperature, Tan et al.78 have successfully fabricated unique honeycomb-like CuS mesostructures which are composed of densely packed CuS NPs. In this research, based on the intrinsic ability of CuS to absorb light which originates from the d–d energy band transition of Cu2+ ions,79 the unique honeycomb-like CuS mesoporous nanospheres with high specific surface area80,81 can efficiently absorb irradiation light (Fig. 4), leading to enhanced PTCE. As shown in Fig. 4B, under similar irradiation conditions, the 24.4% PTCE of the CuS mesostructures was proved, compared with their CuS NP precursors, which only displayed 7.5%. Further in vitro experiments (Fig. 4C), when heated in a short time, the CuS mesostructures were able to kill cancerous cells under the irradiation of the NIR laser (980 nm, 0.51 W cm−2) in 5 minutes. This result clearly explained that the assembly of CuS greatly improved its capability to absorb light from small particles to big architectures.
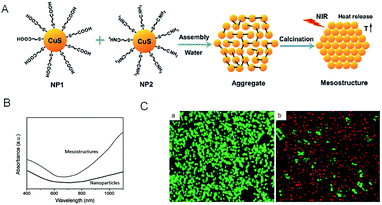 |
| Fig. 4 (A) Schematic illustration of the synthetic procedure of CuS mesostructures. (B) Absorption spectra of the aqueous dispersion of as-synthesized CuS mesostructures and comparable CuS NPs. (C) HeLa cell viability before (a) and after (b) treatment with CuS mesostructures. The living cells were labeled by calcein AM (green emission), and the dead cells were labeled by propidium iodide (red emission).78 Reprinted with permission from ref. 78. Copyright 2015 Royal Society of Chemistry. | |
3.2 CuS superstructure with changed electronic structures
The CuS superstructures in previous reports will exert a great effect on the electronic structures of nanomaterials that can significantly improve the molar extinction coefficient in the NIR region,82,83 which favors energy conversion through the photothermal pathway. In this route, accounting for constructing the superstructure of CuS NPs, the distance of neighboring CuS NPs will shorten, not only allowing for forming new electronic structures of the CuS indirect transition, but also enlarging the entire material near the irradiation absorption cross. Accordingly, improved photothermal performance is therewith realized. Therefore, to be inspiring, this route marks a success on the design and construction of a novel nanostructure and it provides a great kind of potential photothermal therapeutic agent for promoting the development of correlated diagnostic and therapeutic nanotechnologies.
In order to profile the chemism of CuS superstructures that greatly influence the electronic structures, Bu et al.72 developed a room-temperature one-pot route to produce water-dispersible shuttle-like CuS superstructures (Fig. 5). In this work, they demonstrated that the shuttle-like CuS superstructures, compared with CuS NPs, can increase the molar extinction coefficient up to 9.7 × 1016 cm−1 M−1 through shortening the distance of neighboring CuS NPs. Differing from the small CuS NPs, when in the shuttle-like CuS superstructures, these sub-bands based on Brillouin zone folding were deduced for the close-packed CuS NPs with specific dipole orientation.84 And further, generating such new electronic structures offered strong absorption of CuS nanostructures in the NIR region. Most encouragingly, the study noted that the PTCE of the shuttle-like CuS superstructures was calculated to be 72.6%, with the highest photothermal transduction efficiency among the nanomaterials.85 So, it is the electronic structures of the CuS indirect transition that serves as the key determination of optimization for the regular self-assembly structures, which favors energy conversion through a photothermal pathway, further improving NIR laser absorption.
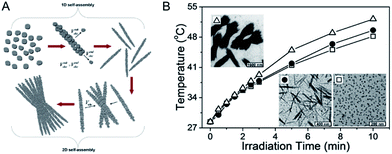 |
| Fig. 5 (A) Schematic illustration of the stepwise self-assembly of CuS NPs to primary nanorods and secondary shuttle-like bundles. (B) Photothermal effect of the aqueous dispersion of CuS NPs (white hollow square), nanorods (black solid round), and bundles (white hollow triangle) under laser irradiation.72 Reprinted with permission from ref. 72. Copyright 2014 American Chemical Society. | |
Similarly, in the work of Cui et al.,86 they demonstrated that the electronic structures would be changed from the non-stoichiometry Cu7S4 NPs to Cu7S4 nano-superlattices by both simulation and experiment (Fig. 6). The changed electronic structures will further influence the LSPR, which is closely related to the PTCE. Through the simulation of LSPR for the dispersed NPs, rod-like alignments and nano-superlattices, respectively, they first found that the LSPR feature of nano-superlattices is far stronger than that of dispersed NPs and rod-like alignments, which is highly desirable for a better PTCE (Fig. 6A). And then, to further verify the aforementioned results of the simulation experiment, Cu7S4 nano-crystals, the rod-like alignments and self-assembled nano-superlattices were prepared by using partly different reaction parameters. As shown in Fig. 6C, under the identical conditions of illumined laser (1 W cm−2 and 808 nm) in a short period, by tuning surface properties of Cu7S4 nano-crystals, the PTCE were 48.62%, 56.32%, 65.7% for dispersed NPs, rod-like alignments, and self-assembled nano-superlattices, respectively. Subsequently, the experiments in vitro suggested that these obtained nano-crystals were quite desirable for synergetic photothermal therapy as well. Thus, this research clearly proved that the assembled nanostructure was beneficial to enhance the NIR absorption and PTCE.
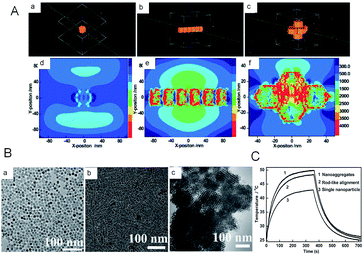 |
| Fig. 6 (A) Simulation of the LSPR for (a, d) single nanoparticles, (b, e) rod-like self-assembly, and (c, f) nanosuperlattices, respectively. (B) TEM images of the prepared nanocomposites. (C) Temperature profiles of obtained nanocomposites irradiated with and without laser.86 Reprinted with permission from ref. 86. Copyright 2015 Wiley-VCH Verlag & Co. KGaA. | |
3.3 Non-stoichiometric copper chalcogenide
LSPRs generally derive from nanostructures of noble metals (such as Au, Pd),34,87 rendering a multitude of possibilities to enhance and tune geometrically their absorption and scattering resonances for different ends. Due to the intense LSPRs absorption, the noble metal NPs exhibit good PTCE and have been widely used for photothermal therapy.88 But not being limited to that, LSPRs are also available from the semiconductor nano-crystals with appreciable deficiencies that will generate a free carrier concentration.89 Differing from the noble metal, whose LSPRs response is permanently locked in and engineered by the choice of nanostructure parameters (such as shape, size, or metal), semiconductor nanostructures could dynamically modulate its LSPRs absorption by doping. The non-stoichiometric copper chalcogenide is exactly one of the typical self-doped semiconductors containing lots of free carriers. As illustrated by Burda et al.90 and Luther et al.,89 non-stoichiometric copper chalcogenide, if its carrier density locates in 1019 to 1022 cm−3, will display a strong absorption in the NIR region, as a result of excess holes in the valence band,89 and meanwhile, no absorption is seen for perfectly stoichiometric Cu2S.91 In addition, not only does copper sulphide nano-crystals possess good absorption characteristics similar to those of metals, but also they present low cost, synthetic simplicity, good photostability, as well as low toxicity, which make them become good potential photothermal agents in nanotechnology photothermal therapeutic platforms.41 Therefore, designing and synthesizing non-stoichiometric copper chalcogenide are assumed to be another promising direction for enhancing the PTCE of photothermal agents and for further use in photothermal ablation therapy.
The first example of non-stoichiometric copper chalcogenide serving as a photothermal agent was Cu2−xSe NPs reported by Hessel et al.15 The obtained Cu2−xSe NPs, about 16 nm coated with an amphiphilic polymer, exhibited a strong NIR absorption in water due to LSPRs (Fig. 7). The molar extinction coefficient of Cu2−xSe arrived at a peak of 7.7 × 107 cm−1 M−1 at 970 nm and was much higher than the indirect optical transition, which was similar to the gold nanostructure. The strong LSPRs absorption in the NIR region resulted in the higher photothermal transduction efficiency of Cu2−xSe NPs (22%), which was almost as high as Au nanorods (21%) and obviously higher than Au nanoshells (13%). Additionally, the significant cell death caused by the photothermal effect of Cu2−xSe NPs, confirmed the good potential applications of the nanoparticle agents for photothermal therapy. Therefore, for better use of copper sulphide NPs in photothermal therapy, one of the key determinants is promoting their PTCE by tuning of the LSPR frequency through controlling the self-doping density.
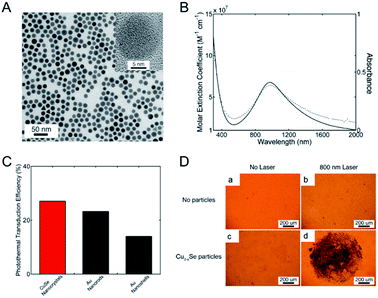 |
| Fig. 7 (A) Typical image of the Cu2−xSe NPs. (B) Molar extinction coefficient (solid) and absorption (dot) for the prepared Cu2−xSe NPs. (C) Plot of photothermal transduction efficiency for Cu2−xSe NPs, gold nanorods and gold nanoshells, respectively. (D) Photothermal destruction of HCT-116 cells without (a and b) and with (c and d) the Cu2−xSe nanocrystals under the irradiation of an 800 nm laser. The dead cell was stained with trypan blue.15 Reprinted with permission from ref. 15. Copyright 2011 American Chemical Society. | |
Obviously, the integral absorption intensity is correlated with the characteristic of the material.89 In order to generate more Cu deficiencies, Tian et al.19 developed a new photothermal agent (plate-like Cu9S5 nano-crystals) on the basis of the thermal decomposition and ligand exchange route (Fig. 8). On account of LSPR triggered by the p-type carriers, the aqueous dispersion of the prepared Cu9S5 NPs exhibited a strong absorption in the NIR region and the calculated molar extinction coefficient at 980 nm is ∼1.2 × 109 M−1 cm−1, which was similar to the Au nanorods (∼1.1 × 109 M−1 cm−1). The strong NIR absorption also resulted in higher heat conversion efficiency for the Cu9S5 nano-crystals (25.7%) compared with the Au nanorods (23.7% nm laser). Further in vivo experiments demonstrated that the high PTCE of Cu9S5 nano-crystals irradiated by a 980 nm laser at the low and safe power density, made it efficiently kill cancerous cells in 10 minutes. Thus, the enhanced PTCE endowed Cu9S5 NPs with the promising potential of being an ideal photothermal agent.
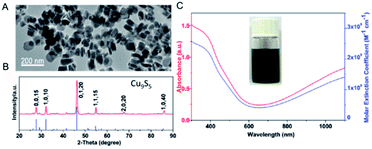 |
| Fig. 8 TEM image (A), and XRD (B), absorption (C, red) and molar extinction coefficient (C, blue) of the prepared Cu9S5 NPs.19 Reprinted with permission from ref. 19. Copyright 2011 American Chemical Society. | |
To further improve the PTCE, it is of great importance to tune or enhance the NIR absorption of non-stoichiometric copper chalcogenide. With the same mechanism, several works have been reported to tune the NIR absorption by controlling copper deficiency in the NPs.92,93 For example, through simple oxidation and reduction, the stoichiometric copper chalcogenide and nonstoichiometric counterparts can transform mutually, permitting the dynamic regulation of the degree of copper deficiency in the copper chalcogenide NPs, along with the changing of the LSPRs absorption of copper chalcogenide.93 All of these demonstrate that we are able to get higher LSPRs absorption in the NIR region of copper chalcogenide, as well as the higher PTCE, through manipulating the degree of copper deficiency. For this purpose, Li et al.60 first reported the preparation of p-type cubic Cu7.2S4 NCs with the free holes that induced the LSPR, showing the appearance of broad and extremely strong peaks in the NIR region, as well as 56.7% PTCE and good photostability when excited by an NIR laser at 980 nm (Fig. 9). To be noted, the peak position of the achieved Cu7.2S4 NCs was tuned to 968 nm as a consequence of the higher degree of copper deficiency compared with the previously reported Cu9S5 NPs, which proved in Luther's study89 that the degree of crystal phase can effectively affect and regulate the LSPR spectroscopy. Also, because the maximum plasmon resonance absorption wavelength of the as-synthesized nano-crystals was tuned by self-doping to be equal to the illumination laser wavelength, the enhanced PTCE of Cu7.2S4 nano-crystals was realized, which was higher than that of Au nanorods, Cu9S5 NCs, Cu2−xSe NCs.94 More importantly, the obtained Cu7.2S4 nano-crystals with higher PTCE were fairly compatible for efficient photothermal ablation therapy both in vitro and in vivo.
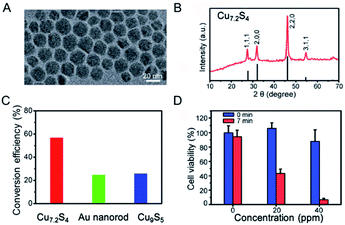 |
| Fig. 9 (A) TEM image and (B) XRD of the obtained Cu7.2S4 NPs. (C) Comparison of PTCE for Cu7.2S4, gold nanorod and Cu9S5 under the irradiation of a 980 nm laser, respectively. (D) Cell viability after treatment with Cu7.2S4 NPs at different concentration before and after the irradiation of the 980 nm laser.60 Reprinted with permission from ref. 60. Copyright 2014 Royal Society of Chemistry. | |
3.4 Copper chalcogenide hybrid nanostructures
Recently, multifunctional theragnostic agents, based on hybrid nanostructures through conjugating with separate different functional NPs, have attracted extensive attention.95–97 These multifunctional photothermal agents can be applied for image contrast and cancer therapy according to the various properties of NPs, including optical and magnetic properties.98–100 As an example, our group proposed the ultrasmall (<10 nm) Fe3O4@Cu2−xS core–shell NPs with rational design and a good synthesis method which possess both super paramagnetic properties and high photothermal stability to attain the combined magnetic and optical functionalities.101 These core–shell NPs not only provided the effective probes for T2-weighted magnetic resonance imaging and infrared thermal imaging, but also could be exerted in the further application for photothermal ablation therapy of cancerous cells. However, the PTCE of the obtained core–shell NPs is lower than the previous report of non-stoichiometric copper chalcogenide. Thus, it is important to understand and explain the synergistic effect resulting from the integration of different functional units, to propel the development of the enhanced PTCE of copper chalcogenide.
On account of the collective oscillation of the large amount of hole carriers in the NPs generated from the copper deficiency, the heavily doped copper chalcogenide shows similar LSPRs absorption with the noble metal, in which the intensity and peak for LSPRs absorption are not merely depended on the material, shape, size, and crystalline structure of the noble metal, but also the surrounding environment.102 As a consequence, the collective oscillation in the copper chalcogenide will be easily influenced by the surface-enhanced near-field at the noble metal NPs surface, realizing the changed LSPRs absorption and the PTCE.103 Based on this, Ding et al.104 reported a dual plasmonic hybrid nanosystem Au–Cu9S5 with well controlled interfaces to investigate the coupling effect of LSPR, determined by the collective electron and hole oscillations (Fig. 10). In their study, the synthesized high-purity Au–Cu9S5 hybrid NPs exhibited a remarkably enhanced LSPR in both visible and NIR regions, and surprisingly achieved ∼50% enhanced absorption at 1064 nm compared with the Au and Cu9S5 NPs when synthesized nearly under the same conditions. Evidently, with the enhanced optical absorption cross section, high photothermal transduction efficiency (37%) helps Au–Cu9S5 hybrids to be a kind of robust photothermal agent in the second NIR window with a low nanomaterial concentration versus their individual component Au and Cu9S5 NPs. Therefore, it is a good way to design copper chalcogenide hybrid nanostructures with enhanced LSPRs absorption and higher PTCE by utilizing surface plasmon resonance enhancement phenomena.
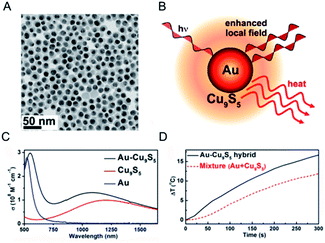 |
| Fig. 10 (A) Typical TEM image of the obtained Au–Cu9S5 hybrid nanostructure. (B) Schematic illustration of a Au–Cu9S5 hybrid to enhance the local field and photothermal performance for Cu9S5. (C) LSPRs absorption of the prepared Au–Cu9S5 hybrid nanostructure (black line) compared with the individual components Au (blue line) and Cu9S5 NPs (red line). (D) Photothermal performance for the Au–Cu9S5 hybrid nanostructure (black solid line) and mixture of Au and Cu9S5 NPs (red dot line).104 Reprinted with permission from ref. 104. Copyright 2014 American Chemical Society. | |
And latterly, several hybrid nanostructures for copper and gold NPs49,67 were designed and synthesized to demonstrate that the PTCE of the hybrid nanostructures can be apparently enhanced by increased LSPRs absorption, due to the coupling effect of LSPRs caused by the collective oscillations of the electron or hole in the Au and Cu2−xS NPs. Based on the coupling effect of LSPRs in the hybrid nanostructures and full utilization of light energy by the yolk–shell structure which greatly improves the multiple refractions of light between cores and shells within the interior voids, Zhang et al.67 has uncovered the unique Au@Cu7S4 yolk–shell structure by an inward replacement strategy according to the Kirkendall effect. As expected, the nanomaterials possessed a powerful photothermal performance with 63% PTCE under the irradiation of 980 nm light and power density of 0.51 W cm−2. Subsequently, Yu et al.49 have further investigated the geometric factors of the hybrid nanostructures acting on their LSPRs absorption and photothermal conversion behavior by using the Au nanorod@Cu2−xS yolk–shell. They regarded that the thinner shell and smaller inner void showed a stronger surface plasmon resonance absorption and a higher PTCE (Fig. 11). In accordance with the experiment result as well as theory simulation, they explained that the electrical field was closely influenced by the shell thickness and the distance between the inner Au nanorods core and the outer Cu7S4 shell. For instance, the electrical field on the surface of the Au nanorods decreased once the Cu7S4 shell thickness increased, whereas electrical field intensity around the Cu7S4 became obviously stronger, along with the reduction in shell size and also a shorter distance between the Au nanorod and Cu7S4 according to the theory simulation results. Based on this mechanism, the optimum PTCE of yolk–shell NPs could be obtained up to 68.6%. Thus, the design and synthesis of the hybrid nanostructure with the synergistic effect of metal and copper chalcogenide is a highly effective approach to enhance the photothermal property of the copper chalcogenide photothermal agents.
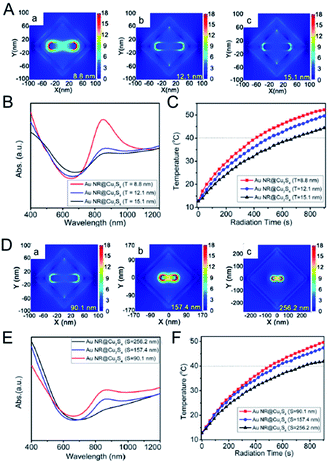 |
| Fig. 11 Electric field distribution of Au nanorod@Cu7S4 hybrid with different shell thickness ((A) a, 8.8 nm; b, 12.1 nm; c, 15.1 nm) and size ((D) a, 90.1 nm; b, 157.4 nm; c, 256.2 nm) in FDTD simulation. (B, E) The LSPRs absorption spectra and (C, F) photothermal profiles for Au nanorod@Cu7S4 hybrid with different shell thickness and size.49 Reprinted with permission from ref. 49. Copyright 2016 American Chemical Society. | |
To sum up, these studies for the copper chalcogenide-based composite could be greatly beneficial to the further design of new kinds of hybrid nanostructures that will exhibit superior properties or functions to enhance NIR absorption and improve the PTCE.
4. Conclusion and outlook
Over the past few years, the near-infrared laser driven photothermal therapy for cancer based on nanoparticle agents has been of particular interest on the spur of its minimally invasive nature compared with traditional therapy. Amongst the nanoparticle agents, such as gold nanoparticle agents and carbon agents, copper chalcogenide nanoparticle agents have attracted more attention recently due to the low cost and easy preparation. Nevertheless, the underdeveloped PTCE of the copper chalcogenide originally limited their wide application for cancer therapy. In this review, we have discussed three kinds of rational design and mechanisms for copper chalcogenide to enhance the heat conversion efficiency in detail and briefly introduced their application in photothermal therapy. First is the design and synthesis of the superstructure nanoparticle agents to enhance photon refraction. Then, tuning the degree of copper deficiency enhances the LSPRs absorption caused by the copper chalcogenide. The rest is constructing the hybrid composite to further enhance the LSPRs absorption through the coupling effect of different functional units. Some typical PTCE of the copper chalcogenide nanoparticles obtained from different design routes are summarized in Table 1 for easy reference. In spite of the higher PTCE and the better treatment effects for cancer that have been achieved by the above three mechanisms, copper chalcogenide nanoparticle agents must be further explored prior to clinical applications.
Table 1 A summary of various copper chalcogenide nanoparticles with enhanced photothermal conversion efficiency
Copper chalcogenide nanoparticle |
PTCE |
Irradiation |
Routes of enhancing PTCE |
Reference |
Flower-like CuS superstructures |
50% enhancement |
980 nm, 0.51 W cm−2 |
CuS superstructure |
48 |
Hollow CuInS2 microspheres |
30% enhancement |
1064 nm, 0.05 W cm−2 |
CuS superstructure |
76 |
CuS mesoporous nanospheres |
24.4% |
980 nm, 0.51 W cm−2 |
CuS superstructure |
78 |
Shuttle-like CuS superstructures |
72.6% |
980 nm, 1 W cm−2 |
CuS superstructure |
72 |
Cu7S4 self-assembled nano-superlattices |
65.7% |
808 nm, 1 W cm−2 |
CuS superstructure |
86 |
Cu2−xSe nanocrystals |
22% |
800 nm, 2 W cm−2 |
Non-stoichiometric copper chalcogenide |
15 |
Plate-like Cu9S5 nano-crystals |
25.7% |
980 nm, 0.51 W cm−2 |
Non-stoichiometric copper chalcogenide |
19 |
Cu7.2S4 nano-crystals |
56.7% |
980 nm, 0.29 W cm−2 |
Non-stoichiometric copper chalcogenide |
60 |
Au–Cu9S5 hybrid nanostructure |
37% |
1064 nm, 0.7 W cm−2 |
Copper chalcogenide hybrid nanostructures |
104 |
Au nanorod@Cu2−xS yolk–shell |
68.6% |
915 nm, 0.42 W cm−2 |
Copper chalcogenide hybrid nanostructures |
49 |
We believe that copper chalcogenide nanoparticle agents have a bright future and will be adopted in clinical applications, provided that several developments are realized as described below: (i) developing a simple and scalable method to synthesize copper chalcogenide nanoparticle agents with uniform and ultra-small size, good stability and bio-compatibility, (ii) further improving the therapeutic effect with rational design and composition of different functional units, (iii) clearly investigating the side-effect of copper chalcogenide nanoparticle agents on the human body, such as potential long-term toxicity in vivo and the additional barrier(s) that may influence the PTCE at the intact animal (organisms) level. Once such challenging problems are addressed, copper chalcogenide nanoparticle agents will become a widely used theranostics platform for cancer in the future.
Acknowledgements
This work was partially supported by National Natural Science Foundation of China (No. 21601124 and 21671135), Program of Young Eastern Scholar from Shanghai Institutions of Higher Learning (QD2015038), Shanghai Rising-Star Program (17QA1402600), the Natural Science Foundation of Shanghai (16ZR1424700), Ministry of Education of China (PCSIRT_IRT_16R49), and International Joint Laboratory on Resource Chemistry (IJLRC).
Notes and references
- R. L. Siegel, K. D. Miller and A. Jemal, Ca-Cancer J. Clin., 2017, 67, 7–30 CrossRef PubMed.
- K. Pu, A. J. Shuhendler, J. V. Jokerst, J. Mei, S. S. Gambhir, Z. Bao and J. Rao, Nat. Nanotechnol., 2014, 9, 233–239 CrossRef CAS PubMed.
- J. Cui, S. Xu, C. Guo, R. Jiang, T. D. James and L. Wang, Anal. Chem., 2015, 87, 11592–11598 CrossRef CAS PubMed.
- X. L. Dean-Ben, S. Gottschalk, B. Mc Larney, S. Shoham and D. Razansky, Chem. Soc. Rev., 2017, 46, 2158–2198 RSC.
- X. Zheng, H. Mao, D. Huo, W. Wu, B. Liu and X. Jiang, Nat. Biomed. Eng., 2017, 1, 0057 CrossRef.
- C. Chu, H. Lin, H. Liu, X. Wang, J. Wang, P. Zhang, H. Gao, C. Huang, Y. Zeng, Y. Tan, G. Liu and X. Chen, Adv. Mater., 2017, 1605928 CrossRef PubMed.
- C. Zhang, D. Ni, Y. Liu, H. Yao, W. Bu and J. Shi, Nat. Nanotechnol., 2017, 12, 378–386 CrossRef CAS PubMed.
- Z. Zhou, J. Song, L. Nie and X. Chen, Chem. Soc. Rev., 2016, 45, 6597–6626 RSC.
- D. Peer, J. M. Karp, S. Hong, O. C. Farokhzad, R. Margalit and R. Langer, Nat. Nanotechnol., 2007, 2, 751–760 CrossRef CAS PubMed.
- L. Cheng, C. Wang, L. Feng, K. Yang and Z. Liu, Chem. Rev., 2014, 114, 10869–10939 CrossRef CAS PubMed.
- L. Cheng, K. Yang, Y. Li, J. Chen, C. Wang, M. Shao, S.-T. Lee and Z. Liu, Angew. Chem., 2011, 123, 7523–7528 CrossRef.
- Y. Huang, Y. Lai, S. Shi, S. Hao, J. Wei and X. Chen, Chem.–Asian J., 2015, 10, 370–376 CrossRef CAS PubMed.
- R. Weissleder, Nat. Biotechnol., 2001, 19, 316–317 CrossRef CAS PubMed.
- W. R. Chen, R. L. Adams, R. Carubelli and R. E. Nordquist, Cancer Lett., 1997, 115, 25–30 CrossRef CAS PubMed.
- C. M. Hessel, V. P. Pattani, M. Rasch, M. G. Panthani, B. Koo, J. W. Tunnell and B. A. Korgel, Nano Lett., 2011, 11, 2560–2566 CrossRef CAS PubMed.
- Z. Chen, Q. Wang, H. Wang, L. Zhang, G. Song, L. Song, J. Hu, H. Wang, J. Liu, M. Zhu and D. Zhao, Adv. Mater., 2013, 25, 2095–2100 CrossRef CAS PubMed.
- H. Yuan, A. M. Fales and T. Vo-Dinh, J. Am. Chem. Soc., 2012, 134, 11358–11361 CrossRef CAS PubMed.
- X. Huang, P. K. Jain, I. H. El-Sayed and M. A. El-Sayed, Lasers Med. Sci., 2007, 23, 217 CrossRef PubMed.
- Q. Tian, F. Jiang, R. Zou, Q. Liu, Z. Chen, M. Zhu, S. Yang, J. Wang, J. Wang and J. Hu, ACS Nano, 2011, 5, 9761–9771 CrossRef CAS PubMed.
- M. Kim, A. Ochirbat and H. J. Lee, Langmuir, 2015, 31, 7609–7615 CrossRef CAS PubMed.
- H. Rao, W. Sun, S. Ye, W. Yan, Y. Li, H. Peng, Z. Liu, Z. Bian and C. Huang, ACS Appl. Mater. Interfaces, 2016, 8, 7800–7805 CAS.
- Y. Wu, C. Wadia, W. Ma, B. Sadtler and A. P. Alivisatos, Nano Lett., 2008, 8, 2551–2555 CrossRef CAS PubMed.
- Y. Zhao and C. Burda, Energy Environ. Sci., 2012, 5, 5564–5576 CAS.
- Z. Li, L. Mi, W. Chen, H. Hou, C. Liu, H. Wang, Z. Zheng and C. Shen, CrystEngComm, 2012, 14, 3965–3971 RSC.
- W. Xu, S. Zhu, Y. Liang, Z. Li, Z. Cui, X. Yang and A. Inoue, Sci. Rep., 2015, 5, 18125 CrossRef CAS PubMed.
- M. Zhou, J. Li, S. Liang, A. K. Sood, D. Liang and C. Li, ACS Nano, 2015, 9, 7085–7096 CrossRef CAS PubMed.
- A. Riedinger, T. Avellini, A. Curcio, M. Asti, Y. Xie, R. Tu, S. Marras, A. Lorenzon, S. Rubagotti, M. Iori, P. C. Capponi, A. Versari, L. Manna, E. Seregn and T. Pellegrino, J. Am. Chem. Soc., 2015, 137, 15145–15151 CrossRef CAS PubMed.
- M. Zhou, R. Zhang, M. Huang, W. Lu, S. Song, M. P. Melancon, M. Tian, D. Liang and C. Li, J. Am. Chem. Soc., 2010, 132, 15351–15358 CrossRef CAS PubMed.
- S. Wang, A. Riedinger, H. Li, C. Fu, H. Liu, L. Li, T. Liu, L. Tan, M. J. Barthel, G. Pugliese, F. De Donato, M. Scotto D'Abbusco, X. Meng, L. Manna, H. Meng and T. Pellegrino, ACS Nano, 2015, 9, 1788–1800 CrossRef CAS PubMed.
- J. T. Robinson, S. M. Tabakman, Y. Liang, H. Wang, H. Sanchez Casalongue, D. Vinh and H. Dai, J. Am. Chem. Soc., 2011, 133, 6825–6831 CrossRef CAS PubMed.
- K. Yang, S. Zhang, G. Zhang, X. Sun, S.-T. Lee and Z. Liu, Nano Lett., 2010, 10, 3318–3323 CrossRef CAS PubMed.
- F. Zhou, S. Wu, B. Wu, W. R. Chen and D. Xing, Small, 2011, 7, 2727–2735 CrossRef CAS PubMed.
- D. Li, D. Han, S.-N. Qu, L. Liu, P.-T. Jing, D. Zhou, W.-Y. Ji, X.-Y. Wang, T.-F. Zhang and D.-Z. Shen, Light: Sci. Appl., 2016, 5, e16120 CrossRef CAS.
- X. Huang, S. Tang, X. Mu, Y. Dai, G. Chen, Z. Zhou, F. Ruan, Z. Yang and N. Zheng, Nat. Nanotechnol., 2011, 6, 28–32 CrossRef CAS PubMed.
- X. Huang, I. H. El-Sayed, W. Qian and M. A. El-Sayed, J. Am. Chem. Soc., 2006, 128, 2115–2120 CrossRef CAS PubMed.
- J. Song, X. Yang, O. Jacobson, P. Huang, X. Sun, L. Lin, X. Yan, G. Niu, Q. Ma and X. Chen, Adv. Mater., 2015, 27, 4910–4917 CrossRef CAS PubMed.
- L. Cheng, W. He, H. Gong, C. Wang, Q. Chen, Z. Cheng and Z. Liu, Adv. Funct. Mater., 2013, 23, 5893–5902 CrossRef CAS.
- Z. Zha, X. Yue, Q. Ren and Z. Dai, Adv. Mater., 2013, 25, 777–782 CrossRef CAS PubMed.
- P. Huang, P. Rong, A. Jin, X. Yan, M. G. Zhang, J. Lin, H. Hu, Z. Wang, X. Yue, W. Li, G. Niu, W. Zeng, W. Wang, K. Zhou and X. Chen, Adv. Mater., 2014, 26, 6401–6408 CrossRef CAS PubMed.
- K. Yang, H. Xu, L. Cheng, C. Sun, J. Wang and Z. Liu, Adv. Mater., 2012, 24, 5586–5592 CrossRef CAS PubMed.
- M. Zhou, M. Tian and C. Li, Bioconjugate Chem., 2016, 27, 1188–1199 CrossRef CAS PubMed.
- Z. Wang, A. von dem Bussche, P. K. Kabadi, A. B. Kane and R. H. Hurt, ACS Nano, 2013, 7, 8715–8727 CrossRef CAS PubMed.
- Y. Li, W. Lu, Q. Huang, C. Li and W. Chen, Nanomedicine, 2010, 5, 1161–1171 CrossRef CAS PubMed.
- J. T. Robinson, K. Welsher, S. M. Tabakman, S. P. Sherlock, H. Wang, R. Luong and H. Dai, Nano Res., 2010, 3, 779–793 CrossRef CAS PubMed.
- Z. Chen, L. Zhang, Y. Sun, J. Hu and D. Wang, Adv. Funct. Mater., 2009, 19, 3815–3820 CrossRef CAS.
- X. Yang, M. Yang, B. Pang, M. Vara and Y. Xia, Chem. Rev., 2015, 115, 10410–10488 CrossRef CAS PubMed.
- S.-W. Hsu, K. On and A. R. Tao, J. Am. Chem. Soc., 2011, 133, 19072–19075 CrossRef CAS PubMed.
- Q. Tian, M. Tang, Y. Sun, R. Zou, Z. Chen, M. Zhu, S. Yang, J. Wang, J. Wang and J. Hu, Adv. Mater., 2011, 23, 3542–3547 CrossRef CAS PubMed.
- X. Yu, J. Bi, G. Yang, H. Tao and S. Yang, J. Phys. Chem. C, 2016, 120, 24533–24541 CAS.
- P. Roy and S. K. Srivastava, Cryst. Growth Des., 2006, 6, 1921–1926 CAS.
- X.-S. Hu, Y. Shen, L.-H. Xu, L.-M. Wang and Y.-J. Xing, J. Alloys Compd., 2016, 674, 289–294 CrossRef CAS.
- J. Li, Q. Wu and J. Wu, in Handbook of Nanoparticles, 2015, pp. 1–28 Search PubMed.
- Q. Lu, F. Gao and D. Zhao, Nano Lett., 2002, 2, 725–728 CrossRef CAS.
- A. Zhang, Q. Ma, M. Lu, G. Zhou, C. Li and Z. Wang, J. Phys. Chem. C, 2009, 113, 15492–15496 CAS.
- W. Bryks, M. Wette, N. Velez, S.-W. Hsu and A. R. Tao, J. Am. Chem. Soc., 2014, 136, 6175–6178 CrossRef CAS PubMed.
- F. Wang, Q. Li, L. Lin, H. Peng, Z. Liu and D. Xu, J. Am. Chem. Soc., 2015, 137, 12006–12012 CrossRef CAS PubMed.
- W. Li, R. Zamani, P. Rivera Gil, B. Pelaz, M. Ibáñez, D. Cadavid, A. Shavel, R. A. Alvarez-Puebla, W. J. Parak, J. Arbiol and A. Cabot, J. Am. Chem. Soc., 2013, 135, 7098–7101 CrossRef CAS PubMed.
- M. Kruszynska, H. Borchert, A. Bachmatiuk, M. H. Rümmeli, B. Büchner, J. Parisi and J. Kolny-Olesiak, ACS Nano, 2012, 6, 5889–5896 CrossRef CAS PubMed.
- M. B. Sigman, A. Ghezelbash, T. Hanrath, A. E. Saunders, F. Lee and B. A. Korgel, J. Am. Chem. Soc., 2003, 125, 16050–16057 CrossRef CAS PubMed.
- B. Li, Q. Wang, R. Zou, X. Liu, K. Xu, W. Li and J. Hu, Nanoscale, 2014, 6, 3274–3282 RSC.
- C. Wu, S.-H. Yu, S. Chen, G. Liu and B. Liu, J. Mater. Chem., 2006, 16, 3326–3331 RSC.
- Y. Sun and Y. Xia, Adv. Mater., 2003, 15, 695–699 CrossRef CAS.
- M. Ji, M. Xu, W. Zhang, Z. Yang, L. Huang, J. Liu, Y. Zhang, L. Gu, Y. Yu, W. Hao, P. An, L. Zheng, H. Zhu and J. Zhang, Adv. Mater., 2016, 28, 3094–3101 CrossRef CAS PubMed.
- C.-H. Kuo, Y.-T. Chu, Y.-F. Song and M. H. Huang, Adv. Funct. Mater., 2011, 21, 792–797 CrossRef CAS.
- C. Dong and F. C. J. M. van Veggel, ACS Nano, 2009, 3, 123–130 CrossRef CAS PubMed.
- H. Jin fan, M. Knez, R. Scholz, K. Nielsch, E. Pippel, D. Hesse, M. Zacharias and U. Gosele, Nat. Mater., 2006, 5, 627–631 CrossRef PubMed.
- J. Zhang, G. Liu, F. He, L. Chen and Y. Huang, RSC Adv., 2015, 5, 87903–87907 RSC.
- E. C. Cho, L. Au, Q. Zhang and Y. Xia, Small, 2010, 6, 517–522 CrossRef CAS PubMed.
- L. Y. T. Chou, K. Zagorovsky and W. C. W. Chan, Nat. Nanotechnol., 2014, 9, 148–155 CrossRef CAS PubMed.
- A. Magasinski, P. Dixon, B. Hertzberg, A. Kvit, J. Ayala and G. Yushin, Nat. Mater., 2010, 9, 353–358 CrossRef CAS PubMed.
- S. Guo and S. Sun, J. Am. Chem. Soc., 2012, 134, 2492–2495 CrossRef CAS PubMed.
- X. Bu, D. Zhou, J. Li, X. Zhang, K. Zhang, H. Zhang and B. Yang, Langmuir, 2014, 30, 1416–1423 CrossRef CAS PubMed.
- A. Usami, Chem. Phys. Lett., 1997, 277, 105–108 CrossRef CAS.
- M. H. Huang, S. Mao, H. Feick, H. Yan, Y. Wu, H. Kind, E. Weber, R. Russo and P. Yang, Science, 2001, 292, 1897 CrossRef CAS PubMed.
- S. Nishimura, N. Abrams, B. A. Lewis, L. I. Halaoui, T. E. Mallouk, K. D. Benkstein, J. van de Lagemaat and A. J. Frank, J. Am. Chem. Soc., 2003, 125, 6306–6310 CrossRef CAS PubMed.
- D. Wu, J. Duan, C. Zhang, K. Guo and H. Zhu, J. Phys. Chem. C, 2013, 117, 9121–9128 CAS.
- S. Ramadan, L. Guo, Y. Li, B. Yan and W. Lu, Small, 2012, 8, 3143–3150 CrossRef CAS PubMed.
- L. Tan, Z. Wu, X. Wang and J. Sun, RSC Adv., 2015, 5, 35317–35324 RSC.
- L. Qian, J. Mao, X. Tian, H. Yuan and D. Xiao, Sens. Actuators, B, 2013, 176, 952–959 CrossRef CAS.
- H. Wu, D. Kong, Z. Ruan, P.-C. Hsu, S. Wang, Z. Yu, T. J. Carney, L. Hu, S. Fan and Y. Cui, Nat. Nanotechnol., 2013, 8, 421–425 CrossRef CAS PubMed.
- A. Corma, P. Atienzar, H. Garcia and J.-Y. Chane-Ching, Nat. Mater., 2004, 3, 394–397 CrossRef CAS PubMed.
- J.-J. Wang, D.-J. Xue, Y.-G. Guo, J.-S. Hu and L.-J. Wan, J. Am. Chem. Soc., 2011, 133, 18558–18561 CrossRef CAS PubMed.
- H. Zhang and D. Wang, Angew. Chem., 2008, 120, 4048–4051 CrossRef.
- S. Goel, F. Chen and W. Cai, Small, 2014, 10, 631–645 CrossRef CAS PubMed.
- D. K. Roper, W. Ahn and M. Hoepfner, J. Phys. Chem. C, 2007, 111, 3636–3641 CAS.
- J. Cui, R. Jiang, S. Xu, G. Hu and L. Wang, Small, 2015, 11, 4183–4190 CrossRef CAS PubMed.
- P. Zijlstra, P. M. R. Paulo and M. Orrit, Nat. Nanotechnol., 2012, 7, 379–382 CrossRef CAS PubMed.
- M. S. Yavuz, Y. Cheng, J. Chen, C. M. Cobley, Q. Zhang, M. Rycenga, J. Xie, C. Kim, K. H. Song, A. G. Schwartz, L. V. Wang and Y. Xia, Nat. Mater., 2009, 8, 935–939 CrossRef CAS PubMed.
- J. M. Luther, P. K. Jain, T. Ewers and A. P. Alivisatos, Nat. Mater., 2011, 10, 361–366 CrossRef CAS PubMed.
- S. Link, C. Burda, M. B. Mohamed, B. Nikoobakht and M. A. El-Sayed, J. Phys. Chem. A, 1999, 103, 1165–1170 CrossRef CAS.
- I. Grozdanov and M. Najdoski, J. Solid State Chem., 1995, 114, 469–475 CrossRef CAS.
- I. Kriegel, C. Jiang, J. Rodríguez-Fernández, R. D. Schaller, D. V. Talapin, E. da Como and J. Feldmann, J. Am. Chem. Soc., 2012, 134, 1583–1590 CrossRef CAS PubMed.
- D. Dorfs, T. Härtling, K. Miszta, N. C. Bigall, M. R. Kim, A. Genovese, A. Falqui, M. Povia and L. Manna, J. Am. Chem. Soc., 2011, 133, 11175–11180 CrossRef CAS PubMed.
- H. Chen, L. Shao, T. Ming, Z. Sun, C. Zhao, B. Yang and J. Wang, Small, 2010, 6, 2272–2280 CrossRef CAS PubMed.
- C. Sun, M. Liu, Y. Zou, J. Wei and J. Jiang, RSC Adv., 2016, 6, 26374–26379 RSC.
- X. Wang, C. Wang, L. Cheng, S.-T. Lee and Z. Liu, J. Am. Chem. Soc., 2012, 134, 7414–7422 CrossRef CAS PubMed.
- J. Bai, Y. Liu and X. Jiang, Biomaterials, 2014, 35, 5805–5813 CrossRef CAS PubMed.
- Q. Tian, Q. Wang, K. X. Yao, B. Teng, J. Zhang, S. Yang and Y. Han, Small, 2014, 10, 1063–1068 CrossRef CAS PubMed.
- K. Yang, L. Hu, X. Ma, S. Ye, L. Cheng, X. Shi, C. Li, Y. Li and Z. Liu, Adv. Mater., 2012, 24, 1868–1872 CrossRef CAS PubMed.
- L.-S. Lin, X. Yang, Z. Zhou, Z. Yang, O. Jacobson, Y. Liu, A. Yang, G. Niu, J. Song, H.-H. Yang and X. Chen, Adv. Mater., 2017, 29, 1606681 CrossRef PubMed.
- Q. Tian, J. Hu, Y. Zhu, R. Zou, Z. Chen, S. Yang, R. Li, Q. Su, Y. Han and X. Liu, J. Am. Chem. Soc., 2013, 135, 8571–8577 CrossRef CAS PubMed.
- R. Jiang, B. Li, C. Fang and J. Wang, Adv. Mater., 2014, 26, 5274–5309 CrossRef CAS PubMed.
- X. Liu, C. Lee, W.-C. Law, D. Zhu, M. Liu, M. Jeon, J. Kim, P. N. Prasad, C. Kim and M. T. Swihart, Nano Lett., 2013, 13, 4333–4339 CrossRef CAS PubMed.
- X. Ding, C. H. Liow, M. Zhang, R. Huang, C. Li, H. Shen, M. Liu, Y. Zou, N. Gao, Z. Zhang, Y. Li, Q. Wang, S. Li and J. Jiang, J. Am. Chem. Soc., 2014, 136, 15684–15693 CrossRef CAS PubMed.
|
This journal is © The Royal Society of Chemistry 2017 |