Nucleophilic degradation of diazinon in thermoreversible polymer–polymer aqueous biphasic systems†
Received
23rd November 2020
, Accepted 27th January 2021
First published on 27th January 2021
Abstract
Although aqueous biphasic systems have been largely investigated in the separation and/or purification of biocompounds, their potential as reaction media to design integrated reaction–separation processes has been less explored. In this work aqueous biphasic systems (ABSs) composed of polypropylene glycol of molecular weight 400 g mol−1 (PPG 400) and different polyethylene glycols (PEGs) were characterized, and investigated for integrated reaction–separation processes, i.e. in the nucleophilic degradation of diazinon and further separation of reaction products by taking advantage of the lower-critical solution temperature (LCST) behaviour of these ABSs. The nucleophilic degradation of diazinon was carried out in the monophasic regime at 298 K, after which an increase in temperature (up to 313 K) allowed the product separation by two-phase formation (thermoreversible systems). The reaction kinetics and reaction pathways have been determined. The reaction kinetic increases as the PEG molecular weight decreases, with the half-life values obtained being competitive to those previously reported using volatile organic solvents as solvent media and significantly higher than under alkaline hydrolysis. One reaction pathway occurs in ABSs comprising PEGs of higher molecular weights, whereas in the ABS composed of PEG 600 two reaction pathways have been identified, meaning that the reaction pathways can be tailored by changing the PEG nature. ABSs formed by PEGs of lower molecular weights were identified as the most promising option to separate the pesticide degradation products by simply applying changes in temperature.
Introduction
Aqueous biphasic systems (ABSs) have been largely investigated in the separation and purification of biocompounds;1,2 this technique is relatively simple, inexpensive, of easy operation, scalable, and allows the purification and concentration stages to be integrated in a single step.1,3 Despite their relevance in this field, their potential as reaction media to design integrated reaction–separation systems has been seldom investigated.4–6 These systems consist of two aqueous phases based on polymer–polymer, polymer–salt, or salt–salt combinations.1,7–9 Both solutes are water-soluble, separating into two coexisting phases above a given concentration, with one of the aqueous phases being enriched in one of the solutes, whereas in the other phase there is a prevalence of the second polymer or salt.
Conventional ABSs are formed by polymer–salt or polymer–polymer mixtures and have been studied for more than five decades; yet, polymer–polymer systems are the choice of preference for the separation, recovery and purification of solutes sensitive to an ionic environment, such as biomolecules, since these systems have a low/negligible ionic strength.1 In these systems, polyethylene glycol (PEG) is commonly used as one of the phase-forming components due to its high biodegradability, low toxicity, low volatility, low melting temperature, large water miscibility and low cost, being commercially available in a wide range of molecular weights.10 Polypropylene glycol (PPG) shares some of the PEG advantages, and can be used as well as an ABS phase-forming component. Accordingly, PEG and PPG were here selected as ABS phase-forming components to minimize the negative impacts of salts to carry out the nucleophilic reaction herein studied.
Environmental contamination and bioaccumulation of pesticides in soils and groundwater is a widespread ecological problem.11 Pesticides are widely used to enhance food production in agricultural practice and, to a lesser extent, to control unwanted pests and disease vectors in public health. However, they cause several health hazards and have a tremendous environmental impact. Organophosphorus pesticides (OPs) are the most used worldwide and their metabolites are widespread across different populations.12–14 The use of strong oxidants or nucleophiles is among the methods most used for their destruction.15 Diazinon is an OP that has a P
S electrophilic center (Scheme 1). It has been demonstrated that OPs show different reaction pathways when they are broken down by N nucleophiles, such as piperidine. They can exhibit up to three reaction pathways, resulting in a large variety of reaction products.16 The degradation pathway depends strongly on the reaction media. However, when the nucleophile is piperidine two main reaction pathways (see Scheme 1), depending on the solvent nature, have been described.17 Furthermore, a recent report18 showed that when the nucleophilic degradation of paraoxon, another OP, is carried out in aqueous solutions of ionic liquids the selectivity of the reaction is strongly dependent on the water amount. In this type of reactions, the degradation product separation is a difficult task, since some of these products are unstable and susceptible to other nucleophilic attack. On the other hand, considering the potential toxicity of OPs and related metabolites and subproducts, the finding of efficient removal methods of these compounds is a pivotal action.
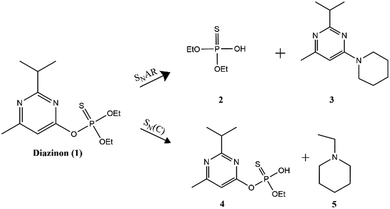 |
| Scheme 1 Reaction pathways for the nucleophilic degradation of diazinon. | |
In this work, ABSs formed by PPG and PEG aqueous mixtures (at their monophasic regimes) were studied as reaction media for the nucleophilic degradation of diazinon, while taking advantage of the effect of temperature to induce two-phase formation (thermoreversible systems) to achieve the separation of the reaction products from the reaction medium. The effect of solvents on the reaction was investigated. The ABSs phase diagrams at several temperatures were determined to infer the mixture composition and temperatures needed to be applied to take advantage of their thermoreversible behavior. The ABS thermoreversible performance to carry out the reaction and proceed with the separation of the reaction products was finally appraised.
Methodology
Chemicals
The ABSs studied in this work were established by using an aqueous solution of polypropylene glycol of molecular weight 400 g mol−1, PPG 400, from Aldrich, and solutions of several PEGs. The PEGs studied were of molecular weight 600 g mol−1, 1000 g mol−1, 2000 g mol−1, 4000 g mol−1, 6000 g mol−1, 8000 g mol−1, 10
000 g mol−1 and 20
000 g mol−1, here abbreviated as PEG 600, PEG 1000, PEG 2000, PEG 4000, PEG 6000, PEG 8000, PEG 10
000 and PEG 20
000, respectively. PEG 600 and PEG 1000 were acquired from Alfa Aesar, PEG 6000 was purchased from Acros, PEG 2000 and PEG 8000 were supplied by Sigma, and the remaining polymers were from Fluka. All polymers were described as pure. Piperidine (99.5%) was distilled before use. Diazinon (100% pure, analytical standard) was purchased from Aldrich.
The water employed was double distilled, passed across a reverse osmosis system and finally treated with a Milli-Q plus 185 water purification apparatus, with a conductivity of 1.60 ± 0.18 μS cm−1.
Phase diagrams
The binodal curve of each phase diagram was determined through the cloud point titration method at several temperatures, namely 288, 298, 308 and 313 K (±1 K), and at atmospheric pressure. The temperature was controlled through a temperature-controlled water bath with a precision of 0.01 K. The experimental method used is detailed elsewhere.19 Aqueous solutions of PPG 400 and PEGs at 90 wt% and from 40 to 70 wt%, respectively, were used in the determination of the PPG–PEG–water ternary phase diagrams. The repetitive dropwise addition of each PEG aqueous solution into the aqueous solution of PPG 400 was carried out until the detection of a cloudy (biphasic) solution, followed by the dropwise addition of ultrapure water until the observation of a limpid solution (monophasic region). All additions were made under continuous stirring. The binodal curves were determined by the weight quantification of all components added (±10−4 g).
Partition of diazinon
From the phase diagrams determined, ternary mixtures composed of 35 wt% PPG 400 + 15 wt% each PEG + 50 wt% H2O (total mass of 2.0 g) were prepared to carry out the partition of diazinon, aiming at identifying the most promising ABSs to develop an integrated reaction–separation process. Ca. 2.0 mg of diazinon was added directly to each ABS and the mixture vigorously stirred until complete solubilization. The phase separation of the ABS was induced by heating and centrifuging the mixture at 313 K at 3000 rpm, during 30 min. Afterwards, a careful separation of the phases was performed, and the diazinon in each phase was quantified by UV-spectroscopy using a BioTeck Synergy HT microplate reader at a wavelength of 247 nm, using a previously established calibration curve. The extraction efficiencies (EE%) of diazinon, defined as the percentage ratio between the amount of diazinon in the PPG-rich aqueous phase and that in the two phases, were determined for each ABS comprising a different PEG. At least three independent ABSs were prepared, and three samples of each phase were quantified. Control or “blank” solutions at the same mixture point used for the extraction studies (with no pesticide added) were used in all the systems.
Diazinon nucleophilic degradation
Nucleophilic reactions were performed in three ABSs, formed by 35 wt% PPG 400 + 15 wt% PEG (600, 2000 and 20
000) + 50 wt% H2O (total mass of 2.0 g). Each mixture was vigorously stirred and left at room temperature until a homogeneous mixture was observed. The pesticide (7.5 mg) and nucleophile (piperidine, 21.3 mg) were added to the mixture, stirred and left at room temperature. Each mixture was made in triplicate. The kinetic reaction was monitored by 31P NMR on a 400 MHz spectrometer following the disappearance of the pesticide signal. Spectra were recorded at different reaction times until the pesticide signal completely disappeared. Then, the phase separation of the thermoreversible ABS was induced by heating up the mixture to 313 K during 30 min. Afterwards, the bottom and top phases were carefully separated and analyzed by 31P-NMR and ESI-MS to identify the reaction products present in each phase.
Results and discussion
Phase diagrams
To evaluate different possibilities and further select the most adequate systems to carry the degradation of diazinon, ternary phase diagrams were determined for various PEGs (PEG 600, 1000, 2000, 4000, 6000, 8000, 10
000 and 20
000) + PPG 400 + water, at 298 K (±1 K) and at atmospheric pressure. The respective ternary phase diagrams are illustrated in Fig. 1, represented in orthogonal coordinates, in which the water content corresponds to the difference to reach 100 wt%. Each point provided in the phase diagram corresponds to a determined experimental solubility point, which is used to describe the binodal or solubility curve of each phase diagram. The solubility curve separates the monophasic region (below the curve) from the biphasic region (above the curve), i.e. at mixture compositions above the bimodal curve there is the formation of two immiscible phases. The experimental weight fraction data of each phase diagram and the adjusted parameters and respective fitting of the experimental data are reported in the ESI.† Some of these systems, namely comprising PEG 600, 2000, 4000 and 6000, were previously reported, being the results here obtained in close agreement with the literature,20 with an average standard deviation of 2.2 wt% – cf. Table S5 in the ESI† with the respective data.
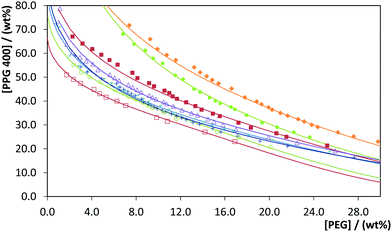 |
| Fig. 1 Phase diagrams for the systems composed of PPG 400 + PEG + H2O with PEGs of different molecular weights: PEG 600 ( ); PEG 1000 ( ); PEG 2000 ( ); PEG 4000 ( ); PEG 6000 ( ), PEG 8000 ( ); PEG 10 000 ( ); PEG 20 000 ( ). The lines correspond to the respective correlations (detailed in the ESI†). | |
Fig. 1 shows the effect of the molecular weight of PEG in the ABS formation. Overall, for PEGs of lower molecular weight, phase separation requires higher quantities of PPG to occur, with the ability to form ABS at a fixed PPG concentration increasing in the following order: PEG 600 < PEG 1000 < PEG 2000 < PEG 4000 < PEG 6000 < PEG 8000 < PEG 10
000 < PEG 20
000. Similar conclusions were reported by Sadeghi and Maali20 in PPG 400 + PEG 600/2000/4000/6000 + water liquid–liquid systems. Analogous behaviours were observed in other ABSs composed of PEG/salt19,21 or PEG/IL pairs.22 This tendency is a consequence of the increase in hydrophobicity displayed by PEGs with higher molecular weights. As discussed by Sadeghi and Maali20 the preferential hydration complexes formed between the more hydrophilic polymer (PEG) and water molecules cause the exclusion of PEG from the near surface region of the more hydrophobic PPG polymer in solution. This exclusion effect increases by increasing the concentration of both polymers and by increasing the PEG molecular weight so that phase formation becomes more entropically favorable.20
Besides the effect of the PEG molecular weight in the ABS formation, in this work, the effect of temperature in the ternary phase diagrams of PEG 600 or PEG 20
000 + PPG 400 + water was evaluated. The phase diagrams of these systems at 288, 298, 308 and 313 K (± 1 K) and atmospheric pressure were determined and are represented in Fig. 2 and 3. The experimental weight fraction data of each phase diagram are reported in the ESI.† With both PEGs, the ability of PPG 400 to form ABS in the presence of a fixed PEG concentration increases in the following temperature order: 288 K < 298 K < 308 K < 313 K. Overall, the required polymer concentration to form a biphasic system decreases by increasing the temperature, following a lower critical solution temperature (LCST) behavior. This behaviour is particularly due to the PPG 400 hydrophobicity that increases with temperature23 and, therefore, the required concentration of PEG to form a second phase decreases. The same behavior was observed by Sadeghi and Maali20 with PPG + PEG + water and PPG + PEGDMEs (polyethylene glycol dimethyl ether) + water systems. At lower temperatures, low-energy polar conformations are present in the polymers, leading to a higher aqueous solubility and a lower ability to form ABS; on the other hand, at higher temperatures a higher energy and less polar conformations are favored, increasing the ability to form ABS.20 Overall, at higher temperatures, hydration shells around the polymer are less developed and the entropy of mixing increases, favoring the ABS formation.
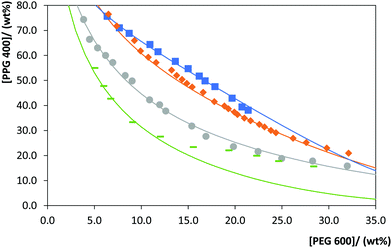 |
| Fig. 2 Phase diagrams for the system composed of PPG 400 + PEG 600 + H2O at different temperatures: 288 K ( ); 298 K ( ), 308 K ( ); 313 K ( ). The lines correspond to the respective correlations (detailed in the ESI†). | |
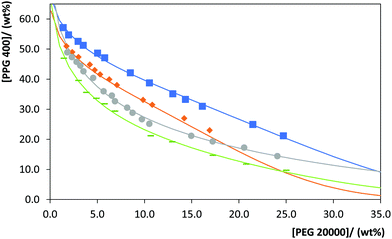 |
| Fig. 3 Phase diagrams for the system composed of PPG 400 + PEG 20 000 + H2O at different temperatures: 288 K ( ); 298 K ( ), 308 K ( ); 313 K ( ). The lines correspond to the respective correlations (detailed in the ESI†). | |
Partition of diazinon
The effect of PEG molecular weight in the partition of diazinon in ABSs formed by 35 wt% PPG 400 + 15 wt% PEG + 50 wt% H2O was firstly evaluated at 313 K (± 1 K) to gather preliminary information on promising systems to carry out the integrated degradation-separation process. The extraction efficiencies (EE%) for diazinon, which correspond to the percentage ratios between the amount of diazinon in the PPG-rich aqueous phases and that in the two phases, in the different ABSs studied are shown in Fig. 4 (detailed results of which are given in the ESI†). In all the systems, diazinon preferentially partitions to the most hydrophobic phase, that is, the PPG-rich phase, with extraction efficiencies ranging between 54.5% and 93.7%. The logarithm of the octanol–water partition coefficient of diazinon, log(Kow) = 3.8124 supports this pesticide's high hydrophobicity and preferential partition to the PPG-rich phase. Although an increase in the EE% of diazinon to the PPG-rich phase is visible when increasing the PEG molecular weight from 600 to 2000 g mol−1, results obtained with ABSs formed by PEGs with a molecular weight equal and higher than 2000 are similar (86.6–93.7%), suggesting that after a certain threshold the increase in the PEG molecular weight has a negligible influence in the diazinon partition to the PPG-rich phase.
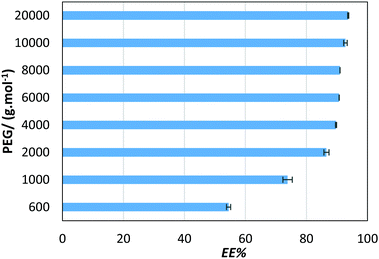 |
| Fig. 4 Extraction efficiencies (EE%) of diazinon to the PPG-rich phases in ABSs formed by 35 wt% PPG 400 + 15 wt% PEG + 50 wt% H2O at 313 K. | |
Considering the extraction efficiencies of diazinon in all the ABSs studied, the systems composed of PPG 400 + PEG (600, 2000 and 20
000) + H2O were selected to perform the reaction of nucleophilic substitution of diazinon. The selection of the three PEGs will allow the molecular weight of the polymer and viscosity of the medium in the nucleophilic degradation of diazinon to be evaluated, while taking into account the different partition trends obtained.
Nucleophilic substitution reactions of diazinon in ABS
The nucleophilic degradation of diazinon and the recovery of the products was carried out in three ABSs, as described above, composed of 35 wt% PPG 400 + 15 wt% PEG (600, 2000 and 20
000) + 50 wt% H2O. Each one of these systems was used as a reaction medium to perform the nucleophilic substitution reaction of diazinon with piperidine. These reactions were carried out at 298 K under pseudo-first order conditions (at least 10 fold excess of piperidine) to compare the results with those previously reported in other reaction media.17,25 The kinetics of diazinon's disappearance in these mixtures were followed by 31P-NMR at room temperature, the results of which are given in Fig. 5–7. From these data, plots of log(%degradation) vs time were used to calculate the pseudo-first order constants (kobs) in all the solvents – the kobs values obtained for each ABS are shown in Table S11 in the ESI.† The used equations and kinetics details are given in the ESI.† The half-lives (t1/2) calculated from the pseudo-first order constants (kobs) showed in Table S11 in the ESI† increase as the PEG molecular weight decreases, i.e., PEG 20
000 (t1/2 = 1851 min) < PEG 2000 (t1/2 = 1273 min) < PEG 600 (t1/2 = 927 min). It is well known that viscosity of polymer solutions increases with the molecular weight increase, which could affect the reaction kinetics. Furthermore, as a consequence of the higher PEG molecular weight, a different packing degree of the solvent system could lead to a different solvation degree of reagents,26 and thus influence the reaction kinetics as well.
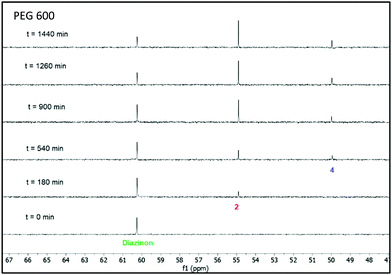 |
| Fig. 5 Stacked 31P-NMR spectra for the nucleophilic degradation of diazinon with piperidine performed in a mixture of 35 wt% PPG 400 + 15 wt% PEG 600 + 50 wt% H2O at 298 K. | |
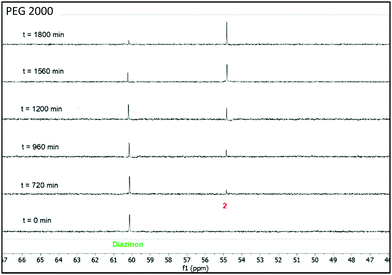 |
| Fig. 6 Stacked 31P-NMR spectra for the nucleophilic degradation of diazinon with piperidine performed in a mixture of 35 wt% PPG 400 + 15 wt% PEG 2000 + 50 wt% H2O at 298 K. | |
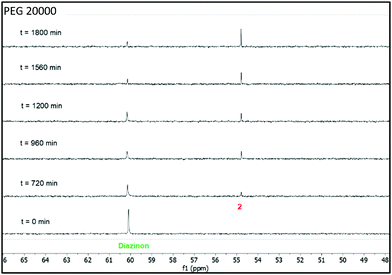 |
| Fig. 7 Stacked 31P-NMR spectra for the nucleophilic degradation of diazinon with piperidine performed in a mixture of 35 wt% PPG 400 + 15 wt% PEG 20 000 + 50 wt% H2O at 298 K. | |
The half-lives (t1/2) values obtained here were compared with those reported previously in other solvents at the same experimental conditions.17,25 This comparison reveals that PEG–PPG aqueous solutions can be used as promising alternative reaction media for the nucleophilic degradation of diazinon. For example, the t1/2 values reported in imidazolium-based ionic liquids range between 71 min and 700 min, while in organic solvents such as MeOH, MeCN and Dioxane these values are 973 min, 1175 min and 2204 min, respectively. Although bio-based solvents have been previously attempted, such as propylene carbonate, the nucleophilic degradation of diazinon was not found to occur.17 The t1/2 values obtained in the current work are similar to those obtained with hazardous volatile organic solvents, yet using aqueous solutions of non-volatile and environmentally friendly polymers.
From the 31P-NMR spectra shown in Fig. 5–7 it can be depicted that as the signal of diazinon decreases (60 ppm) two new phosphoryl signals appear at 55 and 50 ppm in the PEG 600 + PPG 400 + water mixture, while there is only one new phosphorus signal at 55 ppm in the PEG 2000 and 20
000 comprising mixtures. To characterize these NMR signals, product analysis by 31P-NMR and ESI-MS were performed. According to the results obtained here and the literature,17,25 the two phosphoryl signals at 55 and 50 ppm were assigned to diethylthiophosphate (2) and O-ethyl-O-[4-methyl-6-(propan-2-yl)pyrimidin-2-yl]thiophosphate diester (4), respectively, in Scheme 1. Compound 2 is produced in the aromatic pathway (SNAR) by nucleophilic attack at the C-1 carbon of the diazinon's aromatic ring, and product 4 is produced in the aliphatic pathway (SNC) by an attack at the aliphatic carbon of the O-ethyl group of diazinon – cf.Scheme 1. Regarding the non-phosphorylated products, the ESI-MS spectra (Fig. S2 in the ESI†) are in agreement with the structures shown in Scheme 1 for compounds 3 and 5. For the ABS formed by PEG 600 both reaction pathways SNAR and SNC take place, while in the monophasic regimes of ABSs comprising PEG 2000 or PEG 20
000, only the SNAR reaction pathway was observed.
It is important to mention that the diethylthiophosphate (2) visible at 55 ppm is the same product found in the acid or basic hydrolysis of Diazinon.27 Since the mixtures studied here contain 50 wt% water the main reaction product seen in Fig. 5–7 is the one found at 55 ppm, diethylthiophosphate (2). It is interesting to observe that when the PEG 600 mixture was used as the solvent there are two phosphoryl products at 55 ppm and 50 ppm. This suggests that the reaction pathway and its t1/2 are dominated by the transfer phenomena. These results showed that it would be possible to control the reaction pathway by controlling the PEG molecular weight.
Even though the two reaction pathways identified in this work have been previously described for diazinon's degradation in other solvents such as ionic liquids, ACN, DMSO17 and propylene carbonate,25 the relative product distribution reported is different from that obtained in this work. For instance, in ionic liquids, the preference for the aromatic pathway ranges between 28 and 50%; in ACN and DMSO it is about 50% and in propylene carbonate the degradation of diazinon needs more than 100 °C under microwave heating to be completed. In this work, we have found a preference of 80% and 100% for the aromatic pathway in PEG 600-PPG 400 and PEG 2000/20
000-PPG 400 aqueous solutions, respectively. This means that the degradation of diazinon can take place in these ABSs without compromising the reaction rate, while taking advantage of these systems’ thermoreversible nature to easily and effectively separate the most abundant reaction product, the diethylthiophosphate (2), as demonstrated below.
Once the nucleophilic reactions reached equilibria, the systems were heated up to 313 K to induce phase separation and the bottom and top phases were carefully separated and analyzed by 31P-NMR and ESI-MS, the results of which are shown in Fig. 8 for ABSs formed by PEG 600 or PEG 20
000, and in Fig. S3 in the ESI† for PEG 2000.
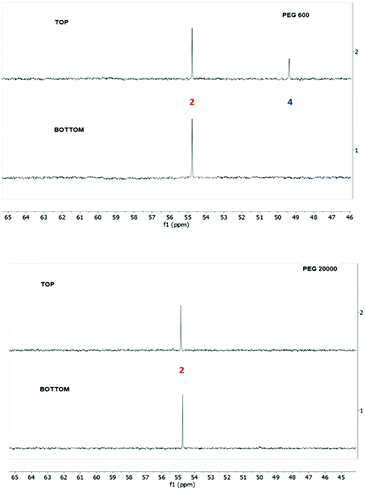 |
| Fig. 8
31P-NMR spectra of both phases of the ABS composed of 35 wt% PPG 400 + 15 wt% PEG (600 and 20 000) + 50 wt% H2O used as - reaction media of nucleophilic degradation of diazinon with piperidine. | |
From the 31P-NMR spectra shown in Fig. 8 and Fig. S3 in the ESI,† it is clear that in ABSs composed of PEG 2000 or PEG 20
000 there is only one phosphorus product at 55 ppm, found in both phases. On the other hand, in the top phase of PEG 600 ABS, two phosphoryl signals at 55 and 50 ppm are seen, whereas in the bottom phase only one peak at 55 ppm appears. These results reveal that PEGs with higher molecular weights are not a promising separation approach for this kind of phosphoryl products. On the other hand, regarding the non-phosphorylated products (3 and 5 in Scheme 1), the ESI-MS spectra (Fig. S2 in the ESI†) show that both of them are found in the top phase of the PEG 600-based ABS, whereas the bottom phase only has diethylthiophosphate (product 2, at 55 ppm), this being a promising option to separate the pesticide degradation products.
To the best of our knowledge there are no previous reports regarding the separation of degradation products of diazinon. Most of the works found in the literature deal with analytical techniques to identify pesticides and their metabolites, mainly based on chromatographic techniques.28–30 In this work we have identified a new and promising solvent to perform the nucleophilic degradation of diazinon based on a PEG 600–PPG 400–water mixture, which acts as both an efficient reaction medium (t1/2 = 927 min) in comparison with the alkaline hydrolysis (t1/2 = 145 h, in similar conditions)31,32 and volatile organic solvents discussed above, but also as a relevant separation platform by simply applying changes in temperature.
Conclusions
In this work, ABSs formed by PPG 400 and PEGs of different molecular weights were investigated as alternative solvents and simultaneous separation platforms for the nucleophilic degradation of the diazinon pesticide. To this end, the ternary phase diagrams of ABSs composed of water, PPG 400 and PEG 600, 1000, 2000, 4000, 6000, 8000, 10
000 or 20
000 were determined at 298 K (± 1 K) and atmospheric pressure. The ternary phase diagrams for ABSs composed of water, PPG 400 and the extreme PEGs, namely PEG 600 or PEG 20
000 were additionally determined at different temperatures (288, 298, 308 and 313 K (± 1 K)) and atmospheric pressure. The experimental data reveal an increase in the capability to form ABSs with the increase of the PEG molecular weight and temperature. After the phase diagram determination, the effect of PEG molecular weight on the partition of diazinon in the ABS formed by 35 wt% PPG 400 + 15 wt% PEG + 50 wt% H2O was evaluated at 313 K (± 1 K). The systems investigated allow extraction efficiencies ranging from 54.5% and 93.7%, with ABSs formed by PEGs of higher molecular weights leading to higher extraction efficiencies of diazinon to the PPG-rich phase. Based on these results, the nucleophilic degradation of diazinon and the recovery of the products were carried out in the systems formed by 35 wt% PPG 400 + 15 wt% PEG (600, 2000 and 20
000) + 50 wt% H2O as reaction media at 298 K, i.e. in the monophasic regime, to perform the nucleophilic substitution reaction of diazinon with piperidine. The results showed that t1/2 values decrease as the PEG molecular weight increases, giving half-life values of 1851 min (PEG 20
000), 1273 min (PEG 2000) and 927 min (PEG 600). The solvents studied allow the degradation of diazinon at a good rate in comparison with organic solvents at similar conditions, in a low-cost and easy to prepare water-rich reaction solvent. The results suggested that the reaction pathway and t1/2 are dominated by the transfer phenomena, and that it would be possible to control the reaction pathway by controlling the PEG molecular weight.
By taking advantage of these systems thermoreversible nature, the temperature was increased up to 313 K to induce ABS phase separation. From the 31P-NMR spectra the presence of phosphorus products in both phases of the ABSs composed of PEG 20
000 or PEG 2000 was observed, while in the system formed from PEG 600, both phosphorus products resulting from the nucleophilic degradation of diazinon migrate toward the top phase and only diethylthiophosphate migrates to the bottom phase. In this sense, with the PPG 400 + PEG 600 + H2O ABS it is possible to separate the most abundant degradation product of diazinon found in the environment, opening an opportunity to develop new techniques in the extraction of this kind of product from different environmental matrices for their subsequent removal.
Conflicts of interest
There are no conflicts to declare.
Acknowledgements
This work was developed within the scope of the project CICECO-Aveiro Institute of Materials, UIDB/50011/2020 and UIDP/50011/2020 financed by national funds through the Portuguese Foundation for Science and Technology/MCTES, D. Millan thanks Fondecyt projects 3150122 and 11170569 and also the NMR facility in the Unidad Central de Investigación at the Pontificia Universidad Católica de Chile for NMR support. A. F. C. S. Rufino acknowledges FCT for the PhD grant SFRH/BD/138997/2018.
References
- M. Iqbal, Y. Tao, S. Xie, Y. Zhu, D. Chen, X. Wang, L. Huang, D. Peng, A. Sattar, M. A. B. Shabbir, H. I. Hussain, S. Ahmed and Z. Yuan, Aqueous two-phase system (ATPS): an overview and advances in its applications, Biol. Proced. Online, 2016, 18, 18 CrossRef.
- P. A. Albertsson, Partition of proteins in liquid polymer-polymer two-phase systems, Nature, 1958, 182, 709–711 CrossRef CAS.
- M. G. Freire, A. F. M. Cláudio, J. M. M. Araújo, J. A. P. Coutinho, I. M. Marrucho, J. N. C. Lopes and L. P. N. Rebelo, Aqueous biphasic systems: a boost brought about by using ionic liquids, Chem. Soc. Rev., 2012, 41, 4966 RSC.
- A. M. Ferreira, H. Passos, A. Okafuji, A. P. M. Tavares, H. Ohno, M. G. Freire and J. A. P. Coutinho, An integrated process for enzymatic catalysis allowing product recovery and enzyme reuse by applying thermoreversible aqueous biphasic systems, Green Chem., 2018, 20, 1218–1223 RSC.
- D. N. Cacace and C. D. Keating, Biocatalyzed mineralization in an aqueous two-phase system: effect of background polymers and enzyme partitioning, J. Mater. Chem. B, 2013, 1, 1794–1803 RSC.
- J. Chen, S. K. Spear, J. G. Huddleston and R. D. Rogers, Polyethylene glycol and solutions of polyethylene glycol as green reaction media, Green Chem., 2005, 7, 64–82 RSC.
-
J. F. B. Pereira and J. A. P. Coutinho, in Handbooks in Separation Science, ed. C. F. B. T.-L.-P. E. Poole, Elsevier, 2020, pp. 157–182 Search PubMed.
- A. F. M. Cláudio, J. F. B. Pereira, P. D. McCrary, M. G. Freire, J. A. P. Coutinho and R. D. Rogers, A critical assessment of the mechanisms governing the formation of aqueous biphasic systems composed of protic ionic liquids and polyethylene glycol, Phys. Chem. Chem. Phys., 2016, 18, 30009–30019 RSC.
- J. F. B. Pereira, K. A. Kurnia, O. A. Cojocaru, G. Gurau, L. P. N. Rebelo, R. D. Rogers, M. G. Freire and J. A. P. Coutinho, Molecular interactions in aqueous biphasic systems composed of polyethylene glycol and crystalline vs. liquid cholinium-based salts, Phys. Chem. Chem. Phys., 2014, 16, 5723–5731 RSC.
- R. Selvaraj, V. Murty, T. Varadavenkatesan, R. Sekar and V. Ramesh, Aqueous Two Phase Systems for the Recovery of Biomolecules – A Review, Sci. Technol., 2011, 1, 7–16 Search PubMed.
- M. L. Ortiz-Hernández, R. Quintero-Ramírez, A. A. Nava-Ocampo and A. M. Bello-Ramírez, Study of the mechanism of Flavobacterium sp. for hydrolyzing organophosphate pesticides, Fundam. Clin. Pharmacol., 2003, 17, 717–723 CrossRef.
- C. Aprea, M. Strambi, M. T. Novelli, L. Lunghini and N. Bozzi, Biologic monitoring of exposure to organophosphorus pesticides in 195 Italian children, Environ. Health Perspect, 2000, 108, 521–525 CrossRef CAS.
- D. B. Barr, R. Bravo, G. Weerasekera, L. M. Caltabiano, R. D. Whitehead Jr, A. O. Olsson, S. P. Caudill, S. E. Schober, J. L. Pirkle, E. J. Sampson, R. J. Jackson and L. L. Needham, Concentrations of dialkyl phosphate metabolites of organophosphorus pesticides in the U.S. population, Environ. Health Perspect., 2004, 112, 186–200 CrossRef CAS.
- C. L. Curl, R. A. Fenske and K. Elgethun, Organophosphorus pesticide exposure of urban and suburban preschool children with organic and conventional diets, Environ. Health Perspect., 2003, 111, 377–382 CrossRef CAS.
- M. Bavcon Kralj, M. Franko and P. Trebše, Photodegradation of organophosphorus insecticides – Investigations of products and their toxicity using gas chromatography–mass spectrometry and AChE-thermal lens spectrometric bioassay, Chemosphere, 2007, 67, 99–107 CrossRef CAS.
- P. Pavez, D. Millán, J. I. Morales, E. A. Castro, C. López A. and J. G. Santos, Mechanisms of degradation of paraoxon in different ionic liquids, J. Org. Chem., 2013, 78, 9670–9676 CrossRef CAS.
- D. Millán, R. A. Tapia and P. Pavez, Efficient Nucleophilic Degradation of an Organophosphorus Pesticide “Diazinon” Mediated by Green Solvents and Microwave Heating, Front. Chem., 2019, 6, 669 CrossRef.
- P. Pavez, R. Figueroa, M. Medina, D. Millán, R. D. Falcone and R. A. Tapia, Choline [Amino Acid] Ionic Liquid/Water Mixtures: A Triple Effect for the Degradation of an Organophosphorus Pesticide, ACS Omega, 2020, 5, 26562–26572 CrossRef CAS.
- M. R. Almeida, H. Passos, M. M. Pereira, Á. S. Lima, J. A. P. Coutinho and M. G. Freire, Ionic liquids as additives to enhance the extraction of antioxidants in aqueous two-phase systems, Sep. Purif. Technol., 2014, 128, 1–10 CrossRef CAS.
- R. Sadeghi and M. Maali, Toward an understanding of aqueous biphasic formation in polymer–polymer aqueous systems, Polymer, 2016, 83, 1–11 CrossRef CAS.
- A. M. Ferreira, V. F. M. Faustino, D. Mondal, J. A. P. Coutinho and M. G. Freire, Improving the extraction and purification of immunoglobulin G by the use of ionic liquids as adjuvants in aqueous biphasic systems, J. Biotechnol., 2016, 236, 166–175 CrossRef CAS.
- M. G. Freire, J. F. B. Pereira, M. Francisco, H. Rodríguez, L. P. N. Rebelo, R. D. Rogers and J. A. P. Coutinho, Insight into the interactions that control the phase behaviour of new aqueous biphasic systems composed of polyethylene glycol polymers and ionic liquids, Eur. Chem. J., 2012, 18, 1831–1839 CrossRef CAS.
- R. Sadeghi and Y. Shahebrahimi, Vapor−Liquid Equilibria of Aqueous Polymer Solutions from Vapor-Pressure Osmometry and Isopiestic Measurements, J. Chem. Eng. Data, 2011, 56, 789–799 CrossRef CAS.
-
C. Hansch, A. Leo and D. Hoekman, Exploring QSAR – Hydrophobic, Electronic, and Steric Constants, American Chemical Society, Washington, DC, 1995 Search PubMed.
- D. Millán, M. Rojas, R. A. Tapia and P. Pavez, Microwave-assisted nucleophilic degradation of organophosphorus pesticides in propylene carbonate, Org. Biomol. Chem., 2020, 18, 7868–7875 RSC.
- S. Tiwari, N. Khupse and A. Kumar, Intramolecular Diels-Alder reaction in ionic liquids: effect of ion-specific solvent friction, J. Org. Chem., 2008, 73, 9075–9083 CrossRef CAS.
-
H. M. Gomaa, I. H. Suffet and S. D. Faust, in Residue Reviews, ed. F. A. Gunther, Springer Berlin, Berlin, Heidelberg, 1969, ch. 5, pp. 170–188 Search PubMed.
- L. Amendola, F. Botrè, A. S. Carollo, D. Longo and L. Zoccolillo, Analysis of organophosphorus pesticides by gas chromatography-mass spectrometry with negative chemical ionization: A study on the ionization conditions, Anal. Chim. Acta, 2002, 461, 97–108 CrossRef CAS.
- V. Andreu and Y. Picó, Determination of pesticides and their degradation products in soil: Critical review and comparison of methods, TrAC, Trends Anal. Chem., 2004, 23, 772–789 CrossRef CAS.
- M. Bavcon Kralj, M. Franko and P. Trebše, Photodegradation of organophosphorus insecticides – Investigations of products and their toxicity using gas chromatography–mass spectrometry and AChE-thermal lens spectrometric bioassay, Chemosphere, 2007, 67, 99–107 CrossRef CAS.
- S. D. Faust and H. M. Gomaa, Chemical hydrolysis of some organic phosphorus and carbamate pesticides in aquatic environments, Environ. Lett., 1972, 3, 171–201 CrossRef CAS.
- S. B. Lartiges and P. P. Garrigues, Degradation Kinetics of Organophesphorus and Organonitrogen Pesticides in Different Waters under Various Environmental Conditions, Environ. Sci. Technol., 1995, 29, 1246–1254 CrossRef CAS.
Footnotes |
† Electronic supplementary information (ESI) available: Experimental weight fraction data for the binodal curves; adjusted parameters and respective standard deviations (σ) obtained by the fitting of the experimental data; comparison of the phase diagrams determined in this work and reported in the literature. See DOI: 10.1039/d0cp06086k |
‡ Equally contributing authors. |
|
This journal is © the Owner Societies 2021 |