Nylon fabric coated with a silver nanowire network covered by graphene oxide sheets serves as an electrostatic air filter for highly efficient particulate matter removal†
Received
29th September 2023
, Accepted 14th November 2023
First published on 15th November 2023
Abstract
Among other environmental issues, air pollution has become an increasing concern. Notably, particulate matter (PM) with a diameter of less than 2.5 micrometers (PM2.5) poses a serious risk to both the environment and public health, necessitating the development of effective, user-friendly, and adaptable prevention and treatment tools. In this study, we introduce a new reusable air filter that can be easily cleaned and captures PM2.5 with significant efficiency. A highly stable network of silver nanowires covered by sheets of graphene oxide were sequentially deposited on nylon fabric by dip coating, followed by 15 min of annealing at 120 °C. The fabricated filter not only removes PM2.5 with high efficiency (greater than 98.7%) but also demonstrates stable reusability. Moreover, the silver nanowires have long-term stability because the graphene oxide layer prevents oxidization in the air. Overall, this research demonstrates the potential of high-performance PM capture devices using simple materials and production methods.
Environmental significance
One of the biggest issues we have today is air pollution. There is a lot of research being done to address this issue, especially for particulate matter with an aerodynamic diameter less than 2.5 micrometers. However, current technology still has drawbacks such as high driving voltage, non-reusability, and high pressure drop. Here, we present a network of silver nanowires coated with graphene oxide sheets and deposited on nylon fabric that functions as an air filter with a strong electrostatic force for trapping PM2.5. The high conductivity of silver nanowire networks makes them suitable for use as high-performance electric-type air filters. Silver nanowire networks are protected from oxidation and atmospheric conditions using the graphene oxide sheet. Here is a novel reusable air filter that can capture PM2.5 and is simple to clean. It also has a low pressure drop and low applied voltage on the filter and the ionizer, which are necessary for optimal performance. Additionally, this approach has various advantages in terms of engineering, such as easy filter fabrication, low voltage usage, low energy consumption, easy reuse, and long-term utility, which may open up viable alternative routes for tackling crises related to air pollution.
|
1. Introduction
The increasing concentration of particulate matter (PM) with a diameter of less than 2.5 micrometers (PM2.5) is one of the growing global concerns, exacerbated by rapid urbanization and economic development over the past decade. Exposure to fine particulates is a chronic problem that poses a significant health risk.1 PM is a mixture of various particles suspended in the air, with different sizes and chemical compositions.2 The size and amount of PM not only affect climate change but also impact public health and numerous organisms, resulting in increased morbidity and mortality.3 PM2.5 must be filtered or removed from the air before it enters the body to avoid these issues.
Researchers have approached the problem in multiple ways, which can be divided into three main methods: scrubbing, filtering, and electrical. Scrubbing methods are limited because they require significant amounts of water, only treat sewage, and are prone to corrosion. Therefore, most research has been focused on electrical and filtration methods. In general, filtration systems capture particles in the air through a combination of three principles: interception, impaction, and diffusion. The operating systems are usually simple, and removal efficiency can be improved by employing multiple layers or increasing the filter thickness, but this causes a high-pressure drop before and after the filter.4,5 In addition, the filter cannot be used several times owing to the damage caused during the washing process. To overcome the drawbacks of conventional filters, several studies have attempted to develop thin, transparent, and highly effective air filters.6–8 However, reusability remains challenging. Finally, electrical types use certain operating principles that consist of discharge and electrostatic attraction. In electrostatic filtration, these mechanisms are associated with mechanical efficiency and electrostatic, therefore, shows the best performance among the three types.9 Additionally, electrical systems exhibit high removal efficiency for any size particle and a relatively low pressure drop. However, this type of system typically requires large equipment and a high voltage on the order of dozens of kV, and thus it has space constraints and poses an explosion hazard for combustible gas. Moreover, ozone (O3), which is toxic to the body, can be generated during discharge because of the high voltage.10–14
Numerous studies have explored various approaches to resolve these issues. Functional electroactive filters can reduce energy consumption but increase PM adhesion in the filter system. Also, these filters can be helpful in reducing air pollution by releasing less ozone into the environment.15–17 A prevalent method for removing small particles and reducing their size is to employ metal nanowires and coated metal nanowire composite filaments as filter materials. Silver nanowires (AgNWs) and AgNW composite meshes are flexible and porous, and they have useful electrical and electrostatic force characteristics, in addition to a strong affinity for air pollutants, which enable high removal efficiencies at low pressure drops.18–20 However, AgNWs quickly react with air moisture, O2, and H2S, resulting in unwanted oxidation, sulfidation, and corrosion, decreasing their long-term stability and electrical conductivity.21,22 To address the demands of practical applications, protective layers must have certain characteristics, such as low-cost manufacturing and high compatibility with AgNW sheet preparation.
Recently, extensive research has been focused on graphene and its derivatives, such as graphene oxide (GO) and reduced GO, as encapsulation layers for AgNWs, leading to the demonstration of large-area flexible hybrid devices.23–25 Owing to their chemical stability and impenetrability to gases, enhanced long-term stability has been achieved in hybrid devices.26–29 Among different graphene-based materials, thin GO sheets exhibit high resistance, making them more suitable for electrical-based filtering applications.30,31 Additionally, the strong adhesion of GO is beneficial for hybridization with other materials in multifunctional devices.30 In this work, we present an AgNW mesh coated with thin GO films, all deposited on nylon sheets by facile dip coating. Notably, the thin GO layer was used to prevent the AgNWs network from oxidizing in air. Furthermore, the GO dispersion was hydrophilic because of its oxygen functional groups and was not electrically conductive.31 Even though it is an insulator, a filter sheet was fabricated with low surface roughness and a high adhesive force, featuring a thin GO layer that could directly pass currents via tunneling, thereby providing the necessary electrical characteristics while improving the oxidation resistance of the AgNWs.32 While controlling the electric potential applied to the filter, we measured the PM2.5 concentrations obtained during filtration in real time. Finally, we studied not only the filter efficiency but also the reusability after cleaning. Specifically, we conducted a cyclic test with repeated cleaning and filtration while considering the changes in efficiency and resistance.
2. Experimental
2.1 Materials and reagents
Ethylene glycol (EG) and anhydrous ferric chloride (FeCl3, 99%) were purchased from Loba Chemicals (Loba Chemie Pvt Ltd). Polyvinylpyrrolidone (PVP, K-90, Mw ≈ 1
300
000 Da) was purchased from Bkkchemi Co., Ltd. Sodium chloride (NaCl, 99%), silver nitrate (AgNO3, 99.8%), potassium chloride (KCl, 99%), acetone (99.5%), and ethanol (99.7%) were purchased from RCI Labscan Ltd. Resorcinol (C6H6O2, 99%) was purchased from HiMedia Laboratories Pvt Ltd. Hydrogen peroxide (H2O2, 35%) and potassium permanganate (KMnO4, 99%) were purchased from Qrec Chemical, Ltd. Sulfuric acid (H2SO4, 95–97%), hydrochloric acid (HCl, 37%), and phosphoric acid (H3PO4, 85%) were obtained from Merck. Graphite powder (<25 μm) was obtained from Sigma-Aldrich. Commercial nylon fabric (500 mesh or 25 μm) was used in this work. All chemicals, solvents, and reagents were of analytical grade and used without further purification. Deionized (DI) water was used throughout the experiments.
2.2 Preparation of AgNWs
The following procedure was used to create long AgNWs. First, 50 mL of EG was used to dissolve 0.3 g of PVP and 0.20 g of AgNO3 (1.18 mmol). Then, a solution of FeCl3 (0–25.0 μM) was added and stirred at room temperature. The resultant mixture was transferred to a 100 mL Teflon hydrothermal bomb reactor and reacted at 130 °C for 8 h. After the reaction was completed, the product was washed with acetone. This washing procedure was repeated twice to remove the excess solvent and chemical agents (PVP and other reactants). The AgNWs were subsequently re-dispersed in ethanol for filtering. Next, the short-length AgNWs and nanoparticles were removed using a nylon filter with a pore size of 25 μm. The long AgNWs remaining on the nylon fabric mesh were collected and re-dispersed in 8 mg mL−1 ethanol.33
2.3 Preparation of GO
Graphite was directly transformed into GO.34 First, 1 g of graphite was stirred in a 9
:
1 acidic solution with 120 mL of H2SO4 and 13.4 mL of H3PO4 at room temperature. To prevent an explosion, 6 g of the oxidant (KMnO4) was slowly added.35 Then, the reaction mixture was heated and the temperature was maintained while being magnetically stirred for a total of 12 h at 50 °C. After each reaction was completed, 400 mL of frozen water was added to quench the solution and help maintain a more constant temperature, and approximately 3 mL of H2O2 was used to assist in decomposing the insoluble manganese salts.36 A few unreacted black particles floating above the acidic solution were removed using a disposable pipette. Through spontaneous sedimentation, the GO was removed from the acidic solution and subsequently washed with HCl, ethanol, and deionized water. Next, GO dispersions were spun at high speed for 3–4 cycles until the pH reached approximately 6. After each centrifugation and redispersion cycle, the GO swelled further. Then, each GO aqueous solution underwent further exfoliation during sonication at approximately 74 W L−1 for 10 min, followed by magnetic stirring at 300 rpm for 5 min. The unexfoliated sheets were removed by centrifuging for 30 min at 2000 rpm three times.37
2.4 Preparation of GO-coated AgNW network on nylon fabric (AGON)
To create the GO-coated AgNWs network on the nylon fabric (AGON) filter, a GO solution was applied after the nylon fabric had been submerged in AgNWs. The PVP surfactant and EG were first removed by washing 20 mL of AgNWs solution twice with ethanol. Then, AgNWs were dispersed in ethanol in a large Petri dish. Commercial nylon was cleaned in ethanol and then acetone before the fabrication process. The nylon fabric was allowed to air dry for 10 min at room temperature before being dipped for 1 min in a mixture of 9% resorcinol and 91% ethyl acetate and dried in an incubator at 60 °C. The AgNW solution was applied to the nylon fabric for 30 s, and it was then allowed to air dry at room temperature. A film of AgNWs gradually developed on the raw nylon fabric as the number of dip coating cycles increased. Following many immersions in an 8 mg mL−1 AgNW solution, the sheet resistance was determined to be within an acceptable range (<5 Ω sq−1). The AgNWs nylon fabric film was then immersed in a GO aqueous solution (1 mg mL−1, 0.5 mL of methanol), and multiple coating cycles were performed while ensuring that the sheet resistance remained below 4 Ω sq−1. Finally, the obtained AGON filter was annealed at 120 °C for 15 min.20
2.5 PM2.5 generation and PM2.5 filtering
According to previous a study,20 burning incense smoke generates PM and provides an atmosphere with a high particle density. During the whole test, the incense continuously fed PM into the chamber system, without being diluted. PM was generated with sizes ranging from 10–300 μm, but most of the particles may have been smaller than 2 μm.6,19 The input particle density exceeded 1000 μg m−3, which is sufficiently dangerous for human health. To determine the particle size produced by the incense, a Benetech GM8803 indoor air analyzer was employed. The JP-D1221 ionizer, a small commercial device for home air purifiers, was used to charge the PM for the experimental filtration system. The PM density was monitored using a laser PM counter sensor module (EcologicSense, France). The particle sensor can simultaneously measure all three particle densities: 1.0 μm (PM1.0), 2.5 μm (PM2.5), and 10 μm (PM10). An electric fan and anemometer (HT-9829, China) were used to assess the efficiency of PM removal under the given flow conditions. To monitor the pressure drop during filtration, a differential pressure gauge (DT-8920/CEM, China P.C.) was employed.
2.6 Cleaning the used AgNW/GO nylon mesh air filter
Cleaning was accomplished using EG, ethanol, and deionized water. The filter was cleaned by immersing it for 30 s in 20 mL of EG, followed by 30 s in ethanol and 30 s in water. The filter was subsequently dried for 5 min at 65 °C in a hot air oven.19,20
2.7 Characterization
Powder X-ray diffraction (XRD, PANalytical X'Pert Pro MPD) was conducted using monochromatized CuKα radiation, an accelerating voltage of 40 kV, and an applied current of 30 mA to investigate the structure of the AgNWs and GO. UV-vis spectroscopy (Agilent, Cary 60) was performed to measure the absorption spectrum of the AgNWs and GO solution in the wavelength range of 300–800 nm. The chemical composition and surface structure of the GO, AgNWs, as-fabricated AGON filter, AGON filter after PM filtration, and AGON filter after cleaning were characterized by field emission scanning electron microscopy (FESEM) combined with energy dispersive X-ray spectroscopy (EDS) (TESCAN, MIRA4). Transmission electron microscopy (TEM) images were obtained using an FEI Tecnai G-20 microscope to observe the shape, distribution, and selected area electron diffraction (SAED) of the GO. Fourier-transform infrared (FT-IR) spectroscopy (Shimadzu, FT-IR 8900) in the wavenumber range of 4000–400 cm−1 and X-ray photoelectron spectroscopy (XPS, AXIS, Ultra DLD) were employed to identify the chemical properties. For the FT-IR approach, a fine powder of the sample was dried in an oven at 110 °C for 12 h, then crushed and mixed with KBr in a ratio of 1
:
1000 by weight. An attenuated total reflection Fourier transform infrared (ATR-FT-IR) spectrometer (Perkin Elmer Model Spectrum GX) was used to determine the chemical structures of the AGON filter before and after filtration. Spectra were obtained with a resolution of 4 cm−1 in the range of 4000–500 cm−1. Raman microscopy was carried out using a system equipped with a 532 nm wavelength laser. The resistances were measured using a multimeter (Fluke, 73III), and the sheet resistances (R) were computed using the equation Rs = R(W/L), where L and W refer to the distance between the two inner edges of the aluminum sheet electrode and the two sides of the filter sheet, respectively.26 The pressure drop and flow rate were measured using a differential pressure gauge (Testo 510, Germany) and a flowmeter (Testo 450-V1, Germany), respectively.
3. Results and discussion
3.1 Characteristics of AgNWs
AgNWs were prepared through a modified one-pot polyol process.33 A mixture containing PVP, AgNO3, FeCl3, and EG was reacted in a hydrothermal bomb reactor at 130 °C for 8 h. The final purification step involved the filtration of the acquired materials. First, acetone was used to extract the unreacted reactants from the cooled reaction mixture. The aggregated AgNWs were redistributed in ethanol for filtration following the elimination of the acetone supernatant. According to previous reports, Fe3+ may also influence the formation of AgNWs.38–40 Here, the key effect of FeCl3 concentration on the diameter and length of AgNWs was investigated using FeCl3 concentrations of 0.5, 0.75, 1.0, and 1.25 μM. Higher energy UV-vis spectra are related to light absorption and scattering in the transverse (short) direction of the nanowires. Meanwhile, absorption and scattering along the long axis (longitudinal) of the nanowires correspond to signals at lower energy. The differences in the absorption peaks can be used to determine the AgNW formations and properties.41 UV-vis spectra of AgNWs for various concentrations of FeCl3 are shown in Fig. S1.† There is a shoulder peak at ∼445 nm using the FeCl3 concentration of 0.5 μM, ascribed to the surface plasmon resonance of Ag nanoparticles, and a shoulder peak appears at ∼445 nm using the FeCl3 concentration of 1.25 μM, which indicates that the final products predominantly contain AgNWs and a small amount of nanoparticles. These results, especially when Cl− is present in high concentrations, may be the result of oxidative etching in the solvent.41 When the concentration of FeCl3 changes from 0.75 to 1.0 μM, only the peaks at ∼354 and ∼393 nm can be observed, indicating that only AgNWs exist in the final product. The most reasonable final product was obtained when the concentration of FeCl3 was 1.0 μM.
To eliminate Ag nanoparticles and short-length AgNWs further, the produced AgNWs were filtered using a nylon filter cloth with a 25 μm pore size opening. From their UV-vis spectra, we used equations to estimate and evaluate the diameter, concentration, and yield of the AgNWs.41 This simple UV-vis method is fast and provides useful information for AgNWs, as summarized in Table S1.†Fig. 1 presents the characterization results for the optimum as-synthesized AgNWs (FeCl3 concentration of 1.0 μM), which were used in further experiments. Fig. 1a shows the XRD pattern of the AgNW sample, exhibiting four peaks at 2θ ≈ 38.2°, 44.6°, 64.3°, and 77.4°, which correspond to the crystalline planes of (111), (200), (220) and (311), respectively (JCPDS file no. 04-0783). All the diffraction peaks can be indexed to the face-centered cubic Ag crystal (a¼b¼c¼, space group Fm
m (225)). The lattice constant calculated from this XRD pattern is 4.080 Å, which is close to the theoretical value of 4.086 Å. The UV-vis absorption spectrum is shown in Fig. 1b, in which the absorption peak at 353 nm is attributed to the plasmon response of long AgNWs, whereas the peak at 399 nm is attributed to the transverse plasmon mode of AgNWs.42 Furthermore, Fig. 1c and e show the SEM images of the AgNWs with lengths of ∼105 μm and diameters of ∼59 nm. The histograms (Fig. 1d and f) show the length and diameter distributions of AgNWs.
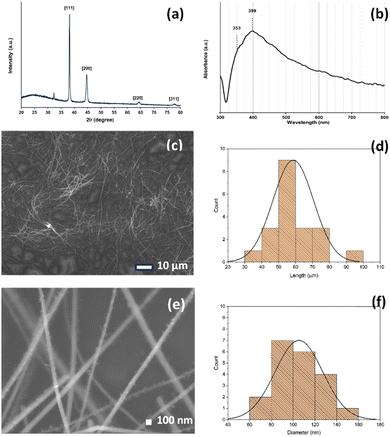 |
| Fig. 1 XRD pattern of the AgNWs showing the FCC crystal (a) UV-vis absorption spectrum of long AgNWs (b) and FESEM image of AgNWs on 10 μm and 100 nm scale (c and e). Histograms showing the distribution of AgNWs lengths and diameters (d and f). | |
3.2 Characteristics of GO
Fig. 2a shows the Raman spectra of GO, in which two distinct peaks around 1500 cm−1 (D and G bands) are essentially identical to the characteristic peaks of GO, corresponding to the D peak at 1353 cm−1 and the G peak at 1599 cm−1. The D peak originates from sp3 carbon atoms with a disordered carbon structure, and the G peak originates from the vibration of sp2 hybrid carbon atoms in the aromatic structure.43 Typically, the ID/IG ratio is used to express the defect degree of nanomaterials.
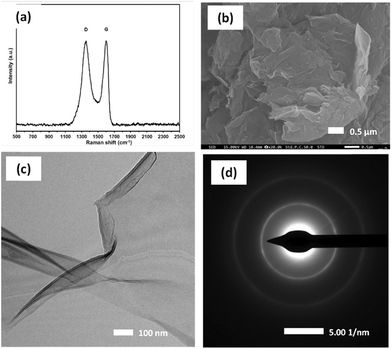 |
| Fig. 2 (a) Raman spectra, (b) SEM image, (c) TEM image, and (d) SAED pattern of GO. | |
The rise in D-band intensity and an increase in the ID/IG ratio observed in the GO samples also confirm the complete formation of GO and the presence of functional groups, which is consistent with the UV-vis (Fig. S3a†), XRD (Fig. S3b†), and FT-IR analyses (Fig. S3c†). Fig. S3a† shows the UV-vis spectroscopy results for the GO sample. The peaks at 224 nm and shoulder at 304 nm are attributed to the π–π* and n–π* transitions, respectively, suggesting that the GO has a lower number of retained aromatic rings.34 Fig. S3b† shows the XRD results for the GO, and the interlayer spacing is related to the degree of oxidation A strong diffraction peak is observed at 2θ ≈ 10°, corresponding to the GO crystal plane (001), and the layer-to-layer distance between GO sheets (0.96 Å), which indicates that the graphite has been oxidized and covered with oxygen-containing functional groups. The FT-IR spectrum (Fig. S3c†) contains peaks at 1068, 1225, and 1624 cm−1, corresponding to C–O, C–O, and C
C bonds, respectively. In addition, the peaks at 1724 and 3410 cm−1 are attributed to the stretching vibration of the COOH and O–H functionalgroups.44Fig. 2b shows the microstructure of GO obtained by SEM using a magnification of 20
000×. The splitting of graphite stacks into layers was observed, revealing a disassembled and crumpled structure. TEM was employed to observe the high-resolution morphology of the GO sample. Fig. 2c shows the flake-like shapes of graphite oxide particles with multilayer GO sheets corrugated together. The selected area electron diffraction pattern of the GO sample (Fig. 2d) exhibits diffuse diffraction rings, and the diffraction dots are unresolved, indicating that the GO samples were amorphous.35
3.3 Characteristics of GO-coated AgNW network on nylon fabric (AGON)
Fig. 3 shows the overall fabrication of the AGON filter using a percolated AgNW network coated with GO. To support the AgNW networks, a nylon fabric was used as a substrate, with a 3/3 twill cross-section. The nylon fabric pore size was approximately 25 μm, which allowed for air permeability while providing a secure base for the AgNW network. The presence of hydrophobic (–CO–NH–) groups within the nylon fabric contributes to its inherent characteristics. To enhance the hydrophilicity of the material, it is possible to introduce polar groups onto its surface.
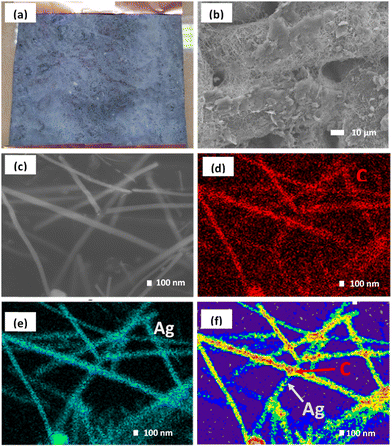 |
| Fig. 3 (a) Digital camera image of AGON filter (b) magnification (800×) SEM micrograph of AGON (c) high magnification (30 000×) SEM micrograph of the AGON (d) EDS element mapping of carbon (e) silver, and (f) carbon and silver on surface of AGON. | |
The nylon fabric was washed with alcohol and acetone before undergoing surface modification. After 30 min of ultrasonic treatment, the fabric was dried at 60 °C and then immersed in a 9% resorcinol and 91% ethyl acetate solution for 60 s before being dried in the air. This is a typical pre-treatment technique for planar nylon surfaces. When nylon fabric is immersed in resorcinol, polar hydroxyl groups are created on its surface, and these groups can subsequently establish hydrogen bonds with the carbonyl groups of PVP, leading to nanowire adhesion. Dip coating and annealing were conducted to transfer a layer of AgNW and a layer of GO onto a nylon fabric and create an AGON filter.
Initially, a solution of ethanol containing AgNWs at a concentration of 8 mg mL−1 was employed as an ink to stain the nylon fabric with a surface area of 25 cm2. By adjusting the number of dipping cycles, it is possible to control the density of the coating of AgNWs and GO on the nylon mesh surface. After dipping eighteen times in an AgNW solution (8 mg mL−1) and three times in a GO solution (1 mg mL−1), followed by heat annealing at 120 °C for 15 min, the sheet resistance was satisfactory (Fig. S4a and b†). SEM images of the synthesized AGON filter using magnifications of 800× and 30
000× are shown in Fig. 3a–c, indicating that GO sheets were deposited on the surfaces of the AgNWs. EDS was conducted to map the elemental composition, as shown in Fig. 3d and e. Element mapping shows that C is homogeneously distributed, and Ag is distributed as nanowires. Comparisons of C from GO and Ag from AgNWs confirm that GO is coated on the AgNW network, as shown in Fig. 3f. The EDS spectrum (Fig. S5†) shows that the concentrations of C and Ag in the AGON filter are 27.2 and 72.8 wt%, respectively. The entire AGON filter preparation process was completed in air at moderate temperatures. Additionally, the process is amenable for large-area manufacturing at a low cost and does not require sophisticated equipment.
3.4 PM2.5 removal characteristics
Prior to the PM2.5 filtration test, the airflow of the filter was assessed. This is a crucial criterion for evaluating the efficiency of an air filter because a higher airflow resistance results in higher energy consumption and a shorter service lifetime. To evaluate the pressure differential across the AGON filter, we performed pressure drop (ΔP) tests on the filter at various airflow rates, ranging from 5 to 21 cm s−1. Similar to traditional filters, inertia impaction filters exhibit a low ΔP of 5–10 Pa and quality factor values of up to 0.380 Pa−1.45 Hence, the operation of the AGON for the PM2.5 filtration system can be verified under a fixed airflow velocity of 12 cm s−1, which results in a ΔP of approximately 9 Pa, as seen in Fig. 4. Fig. 5 shows a schematic diagram of the experimental setup used for testing AGON filter PM2.5 removal. The filter was placed between two separate transparent acrylic chambers. A PM counter sensor was positioned on the left side of the chamber to measure the air density of PM2.5 in real time, and a negative ionizer was positioned on the right side to charge the PM. The PM concentrations were also measured at the input and output sides. Before the experiment started, the right side of the chamber was filled with PM. The concentration of PM2.5 was regulated by injecting air into the intake side near the negative ionizer and controlling the particle density value, which was approximately 1000 μg m−3 for each test. Compared with polymer fiber filters that have polar surface functional groups, the proposed AGON filter is more efficient at removing PM2.5. By adjusting the positive voltage delivered to the AGON filter and keeping it charged, PM removal was actively managed. Furthermore, direct current applied to the negative ion generator using voltages of 3, 5, 7, 9, and 12 V caused the generation of negative ions. Fig. S6† shows the density of PM2.5 removal under these applied voltages. It was found that the higher the voltage of the ionizer, the higher the density of PM2.5 removal, and the maximum efficiency was obtained at 9 V. When the ionizer voltage was increased further to 12 V, the removal efficiency decreased. Thus, the maximum PM2.5 removal performance was obtained when the applied positive voltage was 9 V, as shown in Fig. S7.† Considering the significant influence of the direct current in the negative ionizer, a constant 9 V was used to generate a negative direct current input. The experimental results obtained using various applied voltages (3, 5, 7, 9, and 12 V) on the AGON filter are shown in Fig. 6. The direct current in the negative ionizer set at 9 V and the positive applied voltages on the AGON filter set at 9 V provide the highest PM2.5 removal efficiency.
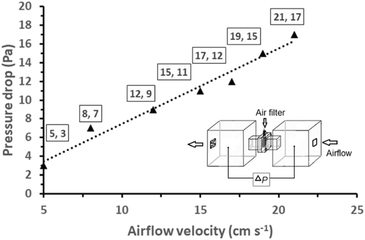 |
| Fig. 4 Pressure drop versus airflow velocity of the AGON filter; the insert shows a schematic diagram of the setup for the measurement of the pressure drop of the AGON filter. | |
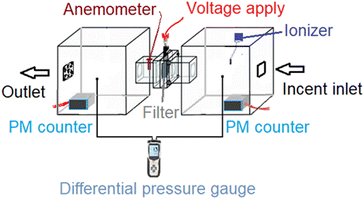 |
| Fig. 5 Schematic experimental setup for PM removal with AGON filter. | |
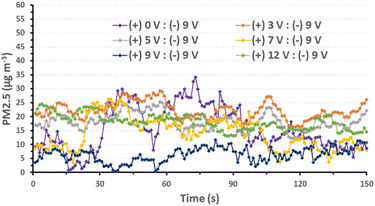 |
| Fig. 6 Removal efficiency of PM2.5 for various AGON filter voltage conditions and ionizer voltage conditions fixed at 9 V. | |
Additionally, we investigated the effects of the ionizer and charging voltage on the AGON air filter plate. The particle density at the output side was measured in real time, and both of these factors were tested using simple basic tests with the following conditions: (a) both turned off, (b) only the AGON turned on (9 V), (c) only the ionizer turned on (9 V), and (d) both turned on. Case (d) exhibited better PM2.5 removal performance than the other cases, as shown in Fig. 7. This implies that the AGON filter plate and ionizer should function together to remove PM2.5. In all cases, the initial particle density removal value was less than 35 μg m−3. This means that the particles passing through the AGON filter were immediately filtered out of the AgNW network, as well as the GO coating. Under an applied positive voltage of 9 V on the AGON filter and a negative voltage fixed at 9 V, the particle density out of the AGON filter was observed in the range of 0.4–12.7 μg m−3.
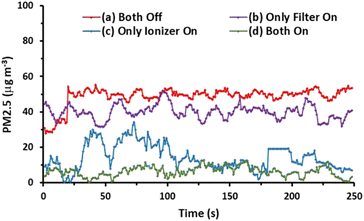 |
| Fig. 7 PM2.5 reduction effectiveness under transient conditions for (a) both are turned off, (b) only AGON is turned on (9 V), (c) only ionizer is turned on (9 V), and (d) both AGON and ionizer are turned on. | |
The key factor contributing to the PM removal may be the electrostatic attraction force between the negative charge of the particle and the positive charge of the AGON filter,19 and the GO coating plays a role in adsorption to help capture PM.44 The removal efficiency (η) was calculated using the following equation:
| 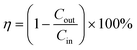 | (1) |
where
Cin and
Cout are the mass concentration (μg m
−3) of the particles before and after removal, respectively. The
η of the AGON filter was calculated to be greater than 98.7% (Fig. S8
†).
Compared with PM filters constructed from polar polymeric nanofibers, the AGON filter showed particularly high efficiency.46
SEM images were used to evaluate the surface morphology before and after PM2.5 filtration and examine the PM2.5 particle capture process. Fig. 8a shows a representative SEM micrograph, revealing the 3/3 twill pattern and weft section of the nylon mesh sheet. The morphology of the as-synthesized AGON, including the percolating AgNW network, GO sheets, and nylon substrate, is observed in Fig. 8b. The randomly percolating network and contact regions between AgNWs provide a conduction path. GO covering the AgNW network acts as a soldered junction, and the layered sheet helps to improve the mechanical strength and long-term stability of the AGON sheet in air, especially by functioning as a gas barrier that prevents AgNWs from oxidizing,45Fig. 8c–e displays the PM captured on the AGON filter surface. We observed that several distinct PM clusters formed first on the positively charged AgNW surfaces, and the negative charge of the PM caused electrostatic Coulomb attraction. The subsequent PM then progressively accumulated over the preexisting clusters with the addition of incoming incense smoke, taking the form of a nearly spherical shape or a flatter, thin layer. The PM may also migrate across the nanowire network and combine to form larger clusters. Furthermore, the arriving PM may cling to the outgoing ones and aggregate. As the capturing process progressed, the larger agglomerated particles eventually filled the AgNW network. Particularly, more particles accumulated at the specific interfaces between materials and then stabilized. As the particle capture duration continued, the AgNWs became thicker, and their diameters significantly increased. Following PM filtration, the AGON filter was soaked in EG for a brief period to prepare the filter for reuse.19,20 The dust particles were eliminated after the EG cleaning process. Fig. 8f shows the surface morphology of the AGON filter after the cleaning process. The surface and structure of the AGON filter remained stable; there was no AgNW or GO sheet breakage or collapse. The surface of the filter returned to its original smooth state.
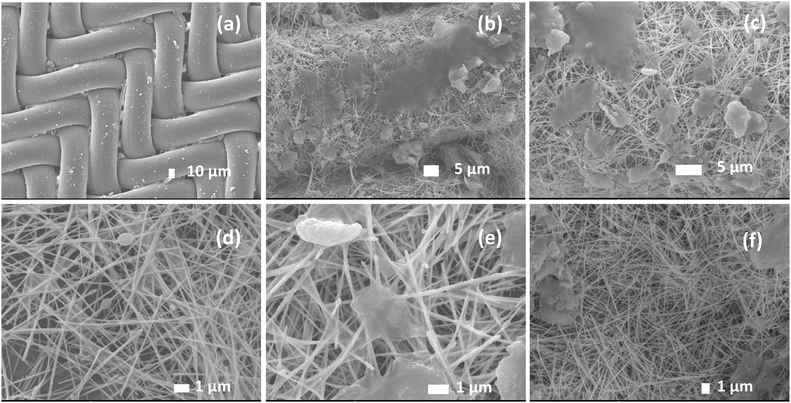 |
| Fig. 8 SEM images displaying the capturing PM particles by AGON filters at different magnifications (a) bare nylon fabric magnification (300×) (b) AGON filter before filtration (c)–(e) AGON filter after filtration magnification (15 000×, 25 000×, 70 000×, respectively), and (f) AGON filter after cleaning process magnification (40 000×). | |
XPS analyses revealed the chemical status and elemental compositions. The XPS survey spectrum (Fig. 9a) indicates that the sample contains mainly Ag, C, O, and N. Fig. 9b shows the Ag 3d spectra obtained from the AGON before PM2.5 removal (AGON-BF), the AGON after PM2.5 removal (AGON-AF), and the AGON filter after the cleaning process (AGON-AC). The peaks observed at 368.6 and 374.6 eV (Fig. 9b) can be ascribed to Ag 3d5/2 and Ag 3d3/2 of the metallic silver, respectively. Silver in metallic states (Ag 3d5/2) has no conversion to other forms such as oxidation state (AgO; Ag 3d5/2), and sulfidation state (Ag2S; Ag 3d5/2). This demonstrates the stability of the silver due to the coating of GO, even after being reused many times. Two distinct peaks of silver, Ag 3d5/2 (368.6 eV) and Ag 3d3/2 (374.6 eV), with a spin energy separation of 6.0 eV, revealed the common characteristics of metallic silver. After PM2.5 filtration (AGON-AF), we observed that the Ag 3d peak exhibited a low signal intensity and change in binding energy, but the peak intensity of the C1s increased. This was likely caused by a significant amount of PM deposited on the surface of the AGON filter network. The main bands at ∼285.0 eV (C1s) are caused by strong C–C bond bindings. The spectra at ∼285.9 (C1s) and 531.5 eV (O1s) are attributed to C–O and C
O bonds. The spectra at ∼288.0 (C1s), 533.7 (O1s), and 532.5 eV (O1s) are all due to C
O bonds. The spectrum at ∼399.8 eV (N1s) is due to the C–N bond, which is the main component of nylon.47 The binding energies of 399.8 (N1s) and 531.3 eV (O1s) correspond to N and O atoms in the amide group –(CO)NH. After PM2.5 filtration, the AGON filter (AGON-AF) has peaks corresponding to Si–C, C
C, Si–O–Si, and C–N bonds at 283.2 (C1s), 284.1 (C1s), 530.7 (O1s), and 399.0 eV (N1s), respectively (Fig. 9c–e). This result confirms that the PM adsorbed on the AGON filter. The AgNWs likely absorb PMs by electrostatic absorption, providing high filtration performance.
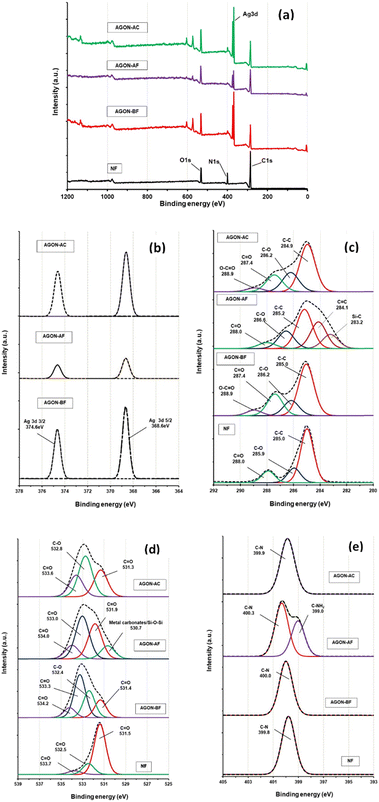 |
| Fig. 9 XPS spectra of bare nylon fabric (NF), AGON filter before and after PM2.5 removal and AGON filter after cleaning process for (a) survey mode, (b) high resolution XPS of Ag 3d, (c) C1s, (d) O1s, and (e) N1s. | |
The AGON filter was then characterized by ATR-FT-IR analysis, as shown in Fig. 10. The ATR-FT-IR spectrum observed after filtering (AGON-AF) contains absorption peaks around 3419 and 1726 cm−1, which are typical of the N–H and C
O functional groups in air pollutant particles. In addition, the absorption peaks around 1199 and 838 cm−1 correspond to the C–N and C–Si functional groups of dust particles absorbed on the AGON filter.19 These analytical findings confirm the AGON filter's ability to capture PM2.5 species. After filtration (AGON-AF), XPS peaks were found at approximately 283.2 (C1s), 284.1 (C1s), 530.7 (O1s), and 399.0 eV (N1s), associated with the C
C, Si–C, Si–O–Si, and C–NH2 bonds within particles that adhered to the AGON filter, but none of these signals were detected after cleaning the filter with EG (AGON-AC). Thus, all of the particles had been completely removed by the cleaning process.
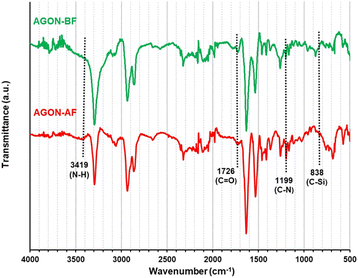 |
| Fig. 10 ATR-FT-IR spectra of AGON filter before (AGON-BF) and after PM2.5 filtration (AGON-AF). | |
To ensure the stability of the AGON filter, it is imperative to perform cyclic tests of repeated cleaning and filtration (Fig. S9†). During each filtering test, the real-time particle detection was performed for 24 h. After a single trial, the chamber filtration system was cleaned with acetone, ethanol, and deionized water to avoid any interference from residual PM. For reused filter experiments, we maintained a particle density exceeding 1000 μg m−3 at the input side of the chamber for all tests. Additionally, after each washing procedure, the AGON's sheet resistance was measured. The normalized sheet resistance (R/Ro) (Fig. S10†) did not change significantly after each cleaning process. The original structure of the AGON filter was also maintained without significant deformation, supporting the reusability of the AGON filter. Moreover, the relative resistance of the AGON filter in this study (R/Ro = 1.04–1.52) was similar to or lower than that observed in previous research (R/Ro = 1.02–1.73),20 with less variation.48 Herein, we fabricate a simple strategy for the AGON filter for efficient PM2.5 capture. It only costs $181 and 1 h to fabricate 1 m2 with no modifications, with a sheet resistance as low as 4 Ω sq−1, a low cost for the cleaning process, and it is easy to scale up production in commercial settings (Table S2†). Compared with previous PM2.5 conductive air filters, which used a hierarchical silver nanowire percolation network,19 electrooptical silver nanowire/TiO2 NP-polyester fabric,12 and reduced graphene-oxide filter,49 all these filter types showed a similar percentage of removal efficiency, close to 99%. The AGON filter used the lowest pressure drop (9 Pa), while both AGON filter and hierarchical silver nanowire percolation network used low power consumption and were reusable after ethylene glycol washing. Overall, the AGON filter exhibited effective PM2.5 removal and high reliability for repeated usage.
4. Conclusions
In this study, we showed that a network of AgNWs covered by sheets of GO and deposited on nylon fabric can be used as an electrostatic air filter for capturing PM2.5. AgNW networks can serve as high-performance electric-type air filters owing to their high conductivity. The GO sheet can be used to protect AgNW networks from atmospheric conditions and oxidization. According to real-time measurements, the fabricated AGON air filter exhibits a higher filtration efficiency than previously reported devices. Notably, an applied positive voltage of 9 V on the AGON filter and a negative voltage of 9 V on the ionizer were required for optimal performance. The key factor contributing to PM removal may be the electrostatic attraction between the positive charge of the AGON filter and the negative charge of the PM. Ultimately, the AGON filter uses relatively low power and has low airflow resistance. After a simple cleaning procedure, the AGON filter remains effective and can be reused several times.
Author contributions
Methodology, data curation, and formal analysis: Siwaporn Janprommin and Jutatip Sommana. Conceptualization, investigation, supervision, and resources: Samroeng Narakaew and Songkot Utara. Project administration, funding acquisition, original draft, review, and editing: Aphiruk Chaisena.
Conflicts of interest
All authors declare no conflict of interest.
Acknowledgements
The authors gratefully acknowledge support of this project by the National Research Council of Thailand (No. NRCT. MHESI. (A) (PS)/439/2563) and the Center of Excellence for Innovation in Chemistry (PERCH-CIC), Ministry of Higher Education, Science, Research, and Innovation (MHESI).
References
- S. Chowdhury, A. Pozzer, A. Haines, K. Klingmüller, T. Münzel, P. Paasonen, A. Sharma, C. Venkataraman and J. Lelieveld, Global health burden of ambient PM2.5 and the contribution of anthropogenic black carbon and organic aerosols, Environ. Int., 2022, 159, 107020 CrossRef CAS.
-
P. K. Rai, in Biomagnetic Monitoring of Particulate Matter, Elsevier, 2016, pp. 1–13 Search PubMed.
- I. Manisalidis, E. Stavropoulou, A. Stavropoulos and E. Bezirtzoglou, Environmental and health impacts of air pollution: A review, Front. Public Health, 2020, 8(14), 1–13 Search PubMed.
- H. Liu, C. Cao, J. Huang, Z. Chen, G. Chen and Y. Lai, Progress on particulate matter filtration technology: basic concepts, advanced materials, and performances, Nanoscale, 2020, 12, 437–453 RSC.
- H. Gao, Y. Yang, O. Akampumuza, J. Hou, H. Zhang and X. Qin, A low filtration resistance three-dimensional composite membrane fabricated via free surface electrospinning for effective PM2.5 capture, Environ. Sci.: Nano, 2017, 4, 864–875 RSC.
- C. Liu, P.-C. Hsu, H.-W. Lee, M. Ye, G. Zheng, N. Liu, W. Li and Y. Cui, Transparent air filter for high-efficiency PM2.5 capture, Nat. Commun., 2015, 6, 6205 CrossRef CAS PubMed.
- V. K. Singh, S. K. Ravi, W. Sun and S. C. Tan, Transparent nanofibrous mesh self-assembled from molecular LEGOs for high efficiency air filtration with new functionalities, Small, 2017, 13, 1601924 CrossRef.
- L. Chen, Y. Guo and X. Peng, Hydrophobic and porous cellulose nanofibrous screen for efficient particulate matter (PM2.5) blocking, J. Phys. D: Appl. Phys., 2017, 50, 405304 CrossRef.
- Y. Gao, E. Tian, Y. Zhang and J. Mo, Utilizing electrostatic effect in fibrous filters for efficient airborne particles removal: Principles, fabrication, and material properties, Appl. Mater. Today, 2022, 26, 101369 CrossRef.
- J. Chen and J. H. Davidson, Ozone production in the positive DC corona discharge: model and comparison to experiments, Plasma Chem. Plasma Process., 2002, 22, 495–522 CrossRef CAS.
- T.-Y. Wen, H.-C. Wang, I. Krichtafovitch and A. V. Mamishev, Novel electrodes of an electrostatic precipitator for air filtration, J. Electrost., 2015, 73, 117–124 CrossRef.
- R.-Y. Zhang and G.-W. Hsieh, Electrostatic polyester air filter composed of conductive nanowires and photocatalytic nanoparticles for particulate matter removal and formaldehyde decomposition, Environ. Sci.: Nano, 2020, 7, 3746–3758 RSC.
- D. Y. Choi, E. J. An, S.-H. Jung, D. K. Song, Y. S. Oh, H. W. Lee and H. M. Lee, Al-coated conductive fiber filters for High-efficiency electrostatic filtration: effects of electrical and fiber structural properties, Sci. Rep., 2018, 8, 5747 CrossRef PubMed.
- M.-W. Kim, S. An, H. Seok, S. S. Yoon and A. L. Yarin, Electrostatic transparent air filter membranes composed of metallized microfibers for particulate removal, ACS Appl. Mater. Interfaces, 2019, 11, 26323–26332 CrossRef CAS.
- K. Zhao, J. Huang, J. Mao, Z. Bao, Z. Chen and Y. Lai, Charged graphene aerogel filter enabled superior particulate matter removal efficiency in harsh environment, Chem. Eng. J., 2020, 395, 125086 CrossRef CAS.
- E. Tian, Q. Yu, Y. Gao, H. Wang, C. Wang, Y. Zhang, B. Li, M. Zhu, J. Mo, G. Xu and J. Li, Ultralow resistance two-stage electrostatically assisted air filtration by polydopamine coated PET coarse filter, Small, 2021, 17, 2102051 CrossRef CAS.
- Y. Gao, E. Tian and J. Mo, Electrostatic polydopamine-interface-mediated (e-PIM) filters with tuned
surface topography and electrical properties for efficient particle capture and ozone removal, J. Hazard. Mater., 2023, 441, 129821 CrossRef CAS PubMed.
- W.-R. Huang, Z. He, J.-L. Wang, J.-W. Liu and S.-H. Yu, Mass production of nanowire-nylon flexible transparent smart windows for PM2.5 capture, iScience, 2019, 12, 333–341 CrossRef CAS PubMed.
- S. Jeong, H. Cho, S. Han, P. Won, H. Lee, S. Hong, J. Yeo, J. Kwon and S. H. Ko, High efficiency, transparent, reusable, and active PM2.5 filters by hierarchical Ag nanowire percolation network, Nano Lett., 2017, 17, 4339–4346 CrossRef CAS PubMed.
- S. Narakaew, S. Thungprasert, S. Janprommin and A. Chaisena, Silver-nanowire/bamboo-charcoal composite percolation network on nylon sheet for improved PM2.5 capture efficiency, Appl. Surf. Sci., 2022, 596, 153666 CrossRef CAS.
- G.-S. Liu, Y. Xu, Y. Kong, L. Wang, J. Wang, X. Xie, Y. Luo and B.-R. Yang, Comprehensive stability improvement of silver nanowire networks via self-assembled mercapto inhibitors, ACS Appl. Mater. Interfaces, 2018, 10, 37699–37708 CrossRef CAS PubMed.
- J. Jiu, J. Wang, T. Sugahara, S. Nagao, M. Nogi, H. Koga, K. Suganuma, M. Hara, E. Nakazawa and H. Uchida, The effect of light and humidity on the stability of silver nanowire transparent electrodes, RSC Adv., 2015, 5, 27657–27664 RSC.
- B.-Y. Wang, E.-S. Lee, Y.-J. Oh and H. W. Kang, A silver nanowire mesh overcoated protection layer with graphene oxide as a transparent electrode for flexible organic solar cells, RSC Adv., 2017, 7, 52914–52922 RSC.
- Y. Zhu, T. Wan, P. Guan, Y. Wang, T. Wu, Z. Han, G. Tang and D. Chu, Improving thermal and electrical stability of silver nanowire network electrodes through integrating graphene oxide intermediate layers, J. Colloid Interface Sci., 2020, 566, 375–382 CrossRef CAS PubMed.
- J. Liang, L. Li, K. Tong, Z. Ren, W. Hu, X. Niu, Y. Chen and Q. Pei, Silver nanowire percolation network soldered with graphene oxide at room temperature and its application for fully stretchable polymer light-emitting diodes, ACS Nano, 2014, 8, 1590–1600 CrossRef CAS PubMed.
- Y. Ahn, Y. Jeong and Y. Lee, Improved thermal oxidation stability of solution-processable silver nanowire transparent electrode by reduced graphene oxide, ACS Appl. Mater. Interfaces, 2012, 4, 6410–6414 CrossRef CAS.
- W.-H. Chung, S.-H. Park, S.-J. Joo and H.-S. Kim, UV-assisted flash light welding process to fabricate silver nanowire/graphene on a PET substrate for transparent electrodes, Nano Res., 2018, 11, 2190–2203 CrossRef CAS.
- A. G. Ricciardulli, S. Yang, G.-J. A. H. Wetzelaer, X. Feng and P. W. M. Blom, Hybrid silver nanowire and graphene-based solution-processed transparent electrode for organic optoelectronics, Adv. Funct. Mater., 2018, 28, 1706010 CrossRef.
- J. Y. Oh and D. Lee, Core–shell structured graphene sphere-silver nanowire hybrid filler embedded polydimethylsiloxane nanocomposites for stretchable conductor, Nanotechnology, 2019, 30, 445706 CrossRef CAS PubMed.
- F. Duan, W. Li, G. Wang, C. Weng, H. Jin, H. Zhang and Z. Zhang, Can insulating graphene oxide contribute the enhanced conductivity and durability of silver nanowire coating?, Nano Res., 2019, 12, 1571–1577 CrossRef CAS.
- D. A. Dikin, S. Stankovich, E. J. Zimney, R. D. Piner, G. H. B. Dommett, G. Evmenenko, S. T. Nguyen and R. S. Ruoff, Preparation and characterization of graphene oxide paper, Nature, 2007, 448, 457–460 CrossRef CAS PubMed.
- I. K. Moon, J. I. Kim, H. Lee, K. Hur, W. C. Kim and H. Lee, 2D graphene oxide nanosheets as an adhesive over-coating layer for flexible transparent conductive electrodes, Sci. Rep., 2013, 3, 1112 CrossRef.
- Y. Zhang, J. Guo, D. Xu, Y. Sun and F. Yan, One-pot synthesis and purification of ultralong silver nanowires for flexible transparent conductive electrodes, ACS Appl. Mater. Interfaces, 2017, 9, 25465–25473 CrossRef CAS.
- D. C. Marcano, D. V. Kosynkin, J. M. Berlin, A. Sinitskii, Z. Sun, A. Slesarev, L. B. Alemany, W. Lu and J. M. Tour, Improved synthesis of graphene oxide, ACS Nano, 2010, 4, 4806–4814 CrossRef CAS.
- D. C. Marcano, D. V. Kosynkin, J. M. Berlin, A. Sinitskii, Z. Sun, A. S. Slesarev, L. B. Alemany, W. Lu and J. M. Tour, Correction to improved synthesis of graphene oxide, ACS Nano, 2018, 12, 2078 CrossRef CAS PubMed.
- S. Eigler, M. Enzelberger-Heim, S. Grimm, P. Hofmann, W. Kroener, A. Geworski, C. Dotzer, M. Röckert, J. Xiao, C. Papp, O. Lytken, H.-P. Steinrück, P. Müller and A. Hirsch, Wet chemical synthesis of graphene, Adv. Mater., 2013, 25, 3583–3587 CrossRef CAS.
- Z. Benzait, P. Chen and L. Trabzon, Enhanced synthesis method of graphene oxide, Nanoscale Adv., 2021, 3, 223–230 RSC.
- M. B. Gebeyehu, T. F. Chala, S.-Y. Chang, C.-M. Wu and J.-Y. Lee, Synthesis and highly effective purification of silver nanowires to enhance transmittance at low sheet resistance with simple polyol and scalable selective precipitation method, RSC Adv., 2017, 7, 16139–16148 RSC.
- C. Wang, Z. Zhang, G. Yang, Q. Chen, Y. Yin and M. Jin, Creation of controllable high-density defects in silver nanowires for enhanced catalytic property, Nano Lett., 2016, 16, 5669–5674 CrossRef CAS PubMed.
- J. Ma and M. Zhan, Rapid production of silver nanowires based on high concentration of AgNO3 precursor and use of FeCl3 as reaction promoter, RSC Adv., 2014, 4, 21060–21071 RSC.
- M.-R. Azani and A. Hassanpour, Synthesis of silver nanowires with controllable diameter and simple tool to evaluate their diameter, concentration and yield, ChemistrySelect, 2019, 4, 2716–2720 CrossRef CAS.
- Y. Sun, Y. Yin, B. T. Mayers, T. Herricks and Y. Xia, Uniform silver nanowires synthesis by reducing AgNO3 with ethylene glycol in the presence of seeds and poly(vinyl pyrrolidone), Chem. Mater., 2002, 14, 4736–4745 CrossRef CAS.
- J.-B. Wu, M.-L. Lin, X. Cong, H.-N. Liu and P.-H. Tan, Raman spectroscopy of graphene-based materials and its applications in related devices, Chem. Soc. Rev., 2018, 47, 1822–1873 RSC.
- W. Zou, B. Gu, S. Sun, S. Wang, X. Li, H. Zhao and P. Yang, Preparation of a graphene oxide membrane for air purification, Mater. Res. Express, 2019, 6, 105624 CrossRef CAS.
- X. Zhang, W. Zhang, M. Yi, Y. Wang, P. Wang, J. Xu, F. Niu and F. Lin, High-performance inertial impaction filters for particulate matter removal, Sci. Rep., 2018, 8, 4757 CrossRef CAS PubMed.
- J. Xu, C. Liu, P.-C. Hsu, K. Liu, R. Zhang, Y. Liu and Y. Cui, Roll-to-roll transfer of electrospun nanofiber film for high-efficiency transparent air filter, Nano Lett., 2016, 16, 1270–1275 CrossRef CAS.
- Q. S. Bhatia, M. C. Burrell and J. J. Chera, XPS surface studies of injection-molded poly(phenylene ether)/nylon 6,6 and poly(phenylene ether)/HIPS blends, J. Appl. Polym. Sci., 1992, 46, 1915–1925 CrossRef CAS.
- Y. Zhang, S. Bai, T. Chen, H. Yang and X. Guo, Facile preparation of flexible and highly stable graphene oxide-silver nanowire hybrid transparent conductive electrode, Mater. Res. Express, 2020, 7, 016413 CrossRef CAS.
- W. Jung, J. S. Lee, S. Han, S. H. Ko, T. Kim and Y. H. Kim, An efficient reduced graphene-oxide filter for PM2.5 removal, J. Mater. Chem. A, 2018, 6, 16975–16982 RSC.
|
This journal is © The Royal Society of Chemistry 2024 |
Click here to see how this site uses Cookies. View our privacy policy here.