Toxicity assessment of nano-sized MAX phases: considerations for safe-by-design approaches†
Received
9th August 2023
, Accepted 17th November 2023
First published on 21st November 2023
Abstract
MAX phases are versatile materials with unique metallic and ceramic properties, utilized in aerospace, nuclear engineering, and high-temperature applications. A comprehensive assessment of the potential hazards and toxicity mechanisms is essential to ensure the safe utilization of these nanomaterials. In light of this, our study investigates the toxicity of two nano-sized MAX phases, Ti2AlC and Ti3AlC2, to provide fundamental data for implementing the safe-by-design (SbD) approach. Cytotoxicity, genotoxicity, and ecotoxicity screening assays were conducted to identify environmental health and safety issues associated with these materials. The comparison with graphene oxide served as a reference nanomaterial in all toxicity tests. At a concentration of 1 mg L−1, MAX phases showed approximately 20% cytotoxicity and a significant increase in DNA strand break marker and IL-6 level in BEAS-2B cells. However, at the same concentration, no significant toxicity was observed in C. elegans and zebrafish embryos. Overall, MAX phases exhibited non-negligible toxicity, with genotoxicity being the most notable endpoint. This study fills the knowledge gap between the prospective use of MAX phases in the biomedical field and their influence on the environment and human health. These findings underscore the importance of evaluating the potential hazards associated with nano-sized MAX phases and provide valuable insights for the implementation of SbD during their research, development, and design phases.
Environmental significance
The potential release of nano-sized MAX phases in various applications raises concerns about their environmental exposure and potential hazards. In response, our study investigated the cytotoxicity, genotoxicity, and ecotoxicity of two representative MAX phases, Ti2AlC and Ti3AlC2, shedding light on their potential risks. Our findings reveal significant cytotoxicity and genotoxicity in human bronchial epithelial cells, underscoring the need for cautious consideration of their use. Furthermore, our research contributes vital data for the implementation of the safe-by-design (SbD) approach, providing a proactive strategy for the development of safer nanomaterials.
|
1. Introduction
A group of ternary carbides and nitrides that exhibit various properties of metallic and ceramic materials, MAX phases have the general formula Mn+1AXn (n = 1, 2, 3…), where M is an early transition metal, A is a group IIIA or IVA element, and X is either nitrogen or carbon.1–3 MAX phase materials have various applications, including aerospace, nuclear engineering, and high-temperature applications,3–5 owing to their appealing material properties, such as good thermal/electrical conductivity, low density, high ductility, toughness, and corrosion resistance.6 The MAX phase can also be used as a precursor for synthesizing MXenes, a family of two-dimensional transition metal carbides, nitrides, and carbonitrides. MXenes are materials with potential applications in various fields such as energy storage and conversion, environment and catalysis, separation membranes, medicine, optics, and electronics.7 As the application range of MXenes increases, the need for research on the hazards of the MAX phase also increases.
In light of the potential versatility of MAX phases for future applications, extensive research has been undertaken to develop various MAX phase materials. However, the assessment of human and environmental hazards associated with these materials remains understudied.8,9 However, MAX phases, being nanomaterials, are also presumed to possess potentially harmful properties. For instance, MAX phase and MXene nanomaterials often have similar physicochemical properties and applications comparable to graphene family nanomaterials (GFNs). GFNs, which were developed earlier than MXene,10 have been extensively studied for their potentially harmful effects in both in vitro and in vivo systems.11 The toxicity of GFNs encompasses physical destruction, oxidative stress, DNA damage, inflammation, apoptosis, autophagy, and necrosis.11–14 Improvements in the toxicological knowledge of MXenes and their precursor MAX phases, which are relatively meager compared to toxicity studies of GFNs, are needed, which will facilitate their broader application.
The “safe-by-design” (SbD) approach addresses environmental, health, and safety (EHS) issues during the research and development (R&D), and design phases of nanotechnologies.15,16 To achieve the development of safer MAX phase materials through the application of SbD, an understanding of potential human and environmental impact is essential.17 In this study, the toxicity of two types of MAX phases, Ti2AlC and Ti3AlC2, were explored to provide a basis for SbD on MAX phases. The status of toxicity studies of MAX phases and MXenes was first confirmed through an analysis of previously published literature. Then, cytotoxicity, genotoxicity, and ecotoxicity screening assays were conducted to identify EHS issues in these materials. Cytotoxicity and genotoxicity assays were performed using human bronchial epithelial cells (BEAS-2B), and toxicity assays using Caenorhabditis elegans and zebrafish were conducted for ecotoxicity screening. All toxicity tests were conducted on Ti2AlC and Ti3AlC2, together with graphene oxide (GO) as a reference nanomaterial.
2. Materials and methods
2.1. Characterization of MAX phases
The commercially available MAX phase particles, Ti2AlC and Ti3AlC2 powders (99.99%), were purchased from Carbon-Ukraine Ltd. (Kyiv, Ukraine). The nano-sized MAX phase particles were obtained by bath-type ultrasonication (Kum Sung, Korea) in 20 mL of distilled water for 16 h. Field emission scanning electron microscopy (FESEM), atomic force microscopy (AFM), energy dispersive spectroscopy (EDS), X-ray photoelectron spectroscopy (XPS), and X-ray diffraction (XRD) were performed to determine the material properties of the MAX phase materials (Table 1). FESEM and EDS (SU8010, HITACHI, Japan) were conducted to examine the surface morphology and identify the elemental composition of the MAX phase particles. The particle thickness and lateral size of the MAX phase particles were characterized using AFM (XE-100, Park Systems, Korea). The bonding on the surface of the MAX phase particles was analyzed using XPS (K-alpha plus Thermo Scientific, USA) with an Al Kα line-focused monochromator (1486.6 eV) with a beam size of 200/400 μm. Survey scans were measured at a pass energy of 200 eV and a step size of 1 eV. Structural characterization of the MAX phase particles was performed by XRD (Rigaku, Smartlab, Japan) over the 2θ range of 5–85° at a scan speed of 3° min−1 and a scan step of 0.05°. The ζ-potentials of the MAX phases in the test media were evaluated using a dynamic light scattering (DLS) spectrometer (ELSZ-1000, Otsuka Electronics Co. Ltd., Japan).
Table 1 Physical property analysis information of MAX phases
Methods |
Manufacturer (product) |
Parameters |
Result |
FESEM |
HITACHI (SU8010) |
Surface morphology |
Fig. 1(a)
|
AFM |
Park Systems (XE-100) |
Particle thickness |
Fig. 1(b and c)
|
EDS |
HITACHI (SU8010) |
Identification of composition of elements |
Fig. 1(d)
|
XPS |
Thermo Fisher Scientific (K-Alpha+) |
Analyzing the surface chemistry |
Fig. 1(e)
|
XRD |
Rigaku (Smartlab) |
Phase identification of a material |
Fig. 1(f)
|
DLS |
Otsuka Electronics (ELSZ-1000) |
Particle size & stability |
Fig. 2
|
2.2. Cell culture and treatment of MAX phases
Human bronchial epithelial cells, BEAS-2B, were purchased from the American Type Culture Collection (Manassas, VA, USA). BEAS-2B cells were maintained in Dulbecco's modified Eagle medium/nutrient mixture F-12 (DMEM/F12, GIBCO BRL Life Technologies, Rockville, MD, USA) supplemented with 10% (v/v) fetal bovine serum and 1% antibiotics, at 37 °C in a humidified atmosphere containing air and 5% CO2. MAX phases (Ti2AlC and Ti3AlC2) were freshly prepared in cell culture medium (DMEM/F12) at the desired concentrations with an appropriate amount from the stock solution (1000 mg L−1 in distilled water). The samples were sonicated for 30 min before biological exposure. In the pristine state, MAX phases are not water-dispersible and thus require treatment with a surfactant, Pluronic-127 (Sigma Aldrich, St. Louis, MO, USA) allow them to disperse in the media. A 0.1% concentration of Pluronic-127 (v/v), which had no adverse effect on the model organisms, was used.
2.3. Cytotoxicity assay
BEAS-2B cells were seeded at a concentration of 1 × 105 cells per mL in 6-well plates and exposed to MAX phases (Ti2AlC and Ti3AlC2) at different concentrations from 0.01 to 1 mg L−1. The treated and untreated cells were incubated for 24 h and harvested for cytotoxicity assays. Cell viability was measured using a RealTime-Glo™ MT cell viability assay (Promega, WI, USA) in a complete DMEM F/12 medium. Three separate experiments were performed for all concentrations in triplicate.
2.4. Comet assay
BEAS-2B cells (5.0 × 104 cells per mL) were seeded in 6-well plates for 24 h before treatment and treated with MAX phases (Ti2AlC and Ti3AlC2) at 1 mg L−1 for the next 24 h. After the treatments, an alkaline (pH > 13) comet assay was performed using the standard method described previously.18 After exposure, the cells were trypsinized and centrifuged at 1100 rpm (200 × g) for 2 min. Approximately 2–6 × 104 cells were resuspended in 0.5% low-melting-point agarose (Bio-Rad Laboratories, CA, USA) at a 1
:
1 ratio. The resuspended cells were placed on slides pre-coated with 1% normal-melting agarose. After solidification, the slides were immersed in cold lysing solution (2.5 M NaCl, 100 mM EDTA, 10 mM Tris-base, 1% N-lauroyl sarcosinate, 1% Triton X-100) for 1.5 h at 4 °C. Slides were transferred to an electrophoresis tank containing freshly made electrophoresis buffer (1 mM EDTA, 300 mM NaOH; pH > 13) and kept for 20 min at room temperature to allow DNA unwinding. Electrophoresis was performed in the same buffer at 25 V and 300 mA at room temperature for 20 min. The slides were neutralized three times with 0.4 M Tris-chloride buffer (pH 7.5), air-dried, and fixed in 70% ethanol. The slides were stained with 40 μL ethidium bromide (10 μg mL−1) and analyzed using a fluorescence microscope (Leica DM IL, Switzerland) at 40× magnification. Approximately 50 cells per slide (three slides per treatment) were examined. DNA damage was expressed as the olive tail moment using image analysis Komet 5.5 software (Kinetic Imaging Ltd., UK). Pb2+ was used as the positive control.
2.5. Inflammation assay
Interleukin (IL)-6 levels in culture supernatants were measured using enzyme-linked immunosorbent assay kits (R&D Systems, Minneapolis, MN, USA). The culture supernatants were collected after each experiment, centrifuged at 11
000 rpm for 20 min, and frozen at −80 °C. IL-6 was quantified in each supernatant in duplicate. The optical density was read on a GloMax® microplate reader (Promega, WI, USA) at a wavelength of 450 nm with a reference wavelength of 540 nm. Values were normalized to those of the control group.
2.6.
C. elegans toxicity assay
C. elegans was provided by the Caenorhabditis Genetics Center (MN, USA), cultured in Petri dishes on a nematode growth medium at 20 °C, and fed OP50 strain Escherichia coli according to the standard protocol.19 Wild-type (N2), and nth-1 (ok724) and ndx-4 (ok1003) loss-of-function mutants were used. The toxicity of the MAX phases on C. elegans was investigated by measuring changes in the reproductive potential after chemical treatment. C. elegans reproduction assays were conducted using a Complex Object Parametric Analyzer and Sorter (COPAS™ SELECT, Union Biometrica, Holliston, MA, USA).20 For the reproduction experiments, 24 replicates were used for each treatment.
2.7. Zebrafish toxicity assay
Adult zebrafish, progeny from wild-type fish, were maintained in a flow-through system of carbon-filtered tap water (pH = 7.9, hardness = 6.7 d, conductivity = 468 S cm−1) at 26 °C with a photoperiod of 14:10 h (light:dark). The fish were fed daily with commercial flakes (SERA Vipan, Germany) as a staple feed supplemented with frozen chironomids and frozen Artemia nauplii (Akvarieteknik, Sweden). The fish were placed in cages in aquaria on the afternoon of the day before egg collection. The MAX phase toxicity in zebrafish was investigated by measuring embryo mortality by chemical treatment. The zebrafish embryo test was conducted according to the Organization for Economic Cooperation and Development test guideline no. 236 with slight modifications.21 Briefly, newly fertilized zebrafish eggs were exposed to the MAX phases for 96 h. The coagulation of fertilized eggs, a marker of mortality, was evaluated at the end of the exposure period. All procedures were approved by the Institutional Animal Care and Use Committee at University of Seoul, and conducted in accordance with relevant guidelines and regulations in Republic of Korea.
2.8. Statistical analyses
The significance of the differences among the treatments was tested using a one-way analysis of variance followed by a post hoc test (Tukey's test, p < 0.05). All statistical analyses were carried out using IBM SPSS (version 20.0; SPSS Inc., IL, USA), and graphs were prepared in SigmaPlot (version 12.0).
3. Results and discussion
3.1. Application and biocompatibility of MAX phases
To investigate the application and biocompatibility of MAX phases and MXenes, relevant literature was collected from PubMed. The search was conducted using keywords such as ‘MAX phase’, ‘MXene’, ‘toxicity’, and ‘biocompatibility’ (January 2022).
The MAX phase has metallic and ceramic properties; light, stiff, and resistant to fire, oxidation, and radiation.22 Research is underway to utilize the MAX phase with these physicochemical properties in various fields. Boatemaa et al. reported that Ti3AlC2 and Ti2AlC resist oxidation in the 900–1400 °C temperature range,23 and Wang et al. confirmed the potential of Ti2AlC to be utilized as a high-temperature solar absorber material.24 The MAX phase has the potential to be applied to nuclear engineering as it is resistant to radiation as well as heat.25 At the evaluation of neutronics and neutron radiation damage response for in-core nuclear applications, Ti3AlC2, and Ti2AlC showed potential,4 Whittle et al. also confirmed the radiation tolerance of Ti3AlC2.26 Since most of the MAX phases have good electrical conductivity,27 many studies have been conducted to use Ti3AlC2, and Ti2AlC as saturable absorbers.28–30 Also, Ti3AlC2 functions as a catalyst in hydrogen storage properties of MgH2 or a catalyst for oxidative dehydrogenation of n-butane.31,32 Overall, given that MAX phases have high application potential in broad fields, the possibility of their exposure to the environment and human body through various media increases, and therefore, a wide range of hazard screening is needed.
In this study, previous studies on the biocompatibility of MAX phases and MXenes were analyzed (Table 2). The number of studies on the biocompatibility of the MAX phase is much smaller (two studies)33,34 than that of MXene (nine studies).35–42 Also, no biocompatibility studies on the Ti3AlC2 and Ti2AlC could be found. Most of the studies for the MXene have focused on its applicability for biomedical purposes, and there have been only two toxicity studies. Various methods were used to measure the physical properties of the materials: nine studies using SEM for morphology, six studies using DLS for particle size/stability, and transmission electron microscopy for structure, four studies using EDS for the elemental composition three studies using XPS for surface chemistry, Fourier transformation for layered reflection, and infrared spectroscopy for bond vibrations. In the studies for the MAX phase, the human cervical cancer (HeLa) cell line and human fibroblast (MSU1.1) cell line33 were used for biomedical application, and rice (Oryza sativa L.) was used for ecotoxicity.34 For the toxicity endpoint, cell viability, reactive oxygen species (ROS), and morphology were measured in the biomedical application study,33 and growth indicators, antioxidant enzyme expression, and morphology were measured in the ecotoxicity study.34
Table 2 Summary of physical property and toxicity analysis information of MAX phases and MXenes
MAX phases/MXenes |
Material characterization |
Toxicity |
Findings |
Ref. |
Methods |
Parameters |
Model |
Endpoint |
TiC |
SEM |
Morphology |
Human cervical cancer (HeLa) cell line, human fibroblast (MSU1.1) cell line |
Viability |
Exposure to high concentrations of TiC and MAX phases <44 μm in size induces cytotoxicity with strong mechanical and chemical membrane disruption. Multilayered MXene affects actin filaments and the associated stress-induced cytoskeleton changes. However, the MXene structure showed excellent cyto-compatibility with cell viabilities |
33
|
Ti2AlC |
HR-TEM |
Structure |
Cytotoxicity |
Ti3AlC2 |
XRD |
Structural property |
ROS |
M-Ti3C2Tx |
Raman |
Vibrational property |
Morphology |
D-Ti3C2Tx |
AFM |
Thickness |
S-Ti3C2Tx |
DLS |
Zeta potential |
Ti3AlC2 |
SEM |
Morphology |
Rice (Oryza sativa L.) |
Germination |
Ti3AlC2 nanosheets entered the leaf cells in the form of Ti ions and activated the immune system. It changes the structure and function of leaves, enhances the activity of antioxidant enzymes, damages cell membranes, reduces biomass, decreases chlorophyll, and inhibits plant growth |
34
|
TEM |
Structure |
Morphology |
HR-TEM |
Structure |
Structure |
EDS |
Components |
Function |
XRD |
Crystal structure |
Water content |
Activity of antioxidant enzymes |
Ti3C2 MXene nanosheets |
TEM |
Thickness |
Human embryonic kidney (HEK)-293 cell |
Cytotoxicity |
Ti3C2 MXene nanosheets for 3D bioprinting application showed high electrical conductivity, and improved mechanical characteristic high viability of most current bioinks |
35
|
Ti3C2 MXene nanocomposite bioink |
XRD |
Presence of elements |
— |
Swelling ratio, morphology, electrical conductivity, compression strength, rheological measurements |
Ti3C2 |
SEM |
Morphology |
Spontaneously transformed aneuploid immortal keratinocyte cell (HaCaT), Human malignant melanoma (A375) cell |
Viability |
2D Ti3C2/PLL hybrids exhibited bactericidal properties and good biocompatibility in a relatively wide concentration range. The tested Ti3C2 MXene phase in both unmodified and surface-modified form did not show cytotoxicity |
36
|
PLL |
EDS |
Elemental composition |
Cellular cycle |
Ti3C2/PLL |
HRTEM |
Morphology |
FFT |
Layered reflection |
IFFT |
Alternating layers |
DLS |
Zeta potential, hydrodynamic diameters |
FTIR |
Characteristic signals derived from bond vibrations |
— |
Band intensity pattern, Isotherm of physical nitrogen sorption, bactericidal properties |
Nb2C nanosheets |
EDS |
Elemental composition |
Porcine cadaver skin, breast cancer cell from BALB/c nude mice (4T1) |
Skin insertion and dissolution test |
Microneedle (MN) system features a neat and complete macroscopic structure, sufficient compressive strength, excellent skin penetration and dissolution abilities, and high photothermal-ablation performance. The MNs exhibited no significant toxicity or adverse effects on the mice |
37
|
Polyvinylpyrrolid one (PVP) |
Electron microscope |
Morphology |
Cytotoxicity photothermal effect |
Microneedle (PVP/Nb2C MN) |
DLS |
Size |
Photothermal-ablation efficacy |
UV-vis-NIR spectrometer |
UV-vis-NIR absorption spectra |
XPS |
Presence of elements |
AFM |
Thickness |
Zwick Materials Testing Machine |
Compressive strength |
SEM |
Morphology |
GTA |
FITR |
Structure |
Mouse fibroblast cell (L929 cell), mice, E. coli, S. aureus, methicillin-resistant S. aureus (MRSA) |
Morphology |
HPEM scaffolds displayed high-antibacterial activity against E. coli, S. aureus, and MRSA and could efficiently reach hemostasis and increase cell proliferation with negligible toxicity in vitro. HPEM significantly enhanced MRSA-infected wound healing via anti-infection, stimulating cell proliferation, an angiogenic process, up-regulating α-SMA and CD31, promoting granulation tissue formation, collagen deposition, vascular endothelial differentiation, and angiogenesis |
38
|
PEI |
SEM |
Morphology |
Viability |
PGE |
TEM |
Structure |
Skin recovery |
HPEM |
EDS |
Elemental composition |
Inflammation |
HCHO |
— |
Viscosity, electronic conductivity, normal stress to the adhesive ability |
Granulation tissue thickness |
HPE |
Collagen content |
MXene@PDA |
Antibacterial activity |
Hemostatic ability |
Ti3C2Tx |
TEM |
Structure |
Zebrafish embryo, human breast cancer cells (MCF7) |
Mortality |
No overall embryonic toxicity or teratogenicity effects for the nanocomposites even at high concentrations. For cancer therapy, efficient apoptosis and photothermal activity were revealed for Au/Fe3O4/MXene nanocomposites |
39
|
Au/MXe ne |
SEM |
Morphology |
Body deformity |
Au/Fe3O 4/MXene |
XRD |
Presence of elements |
Escoliosis |
VSM |
Magnetization of ferromagnetic materials |
Pigmentation |
Yolk edema |
Heart edema |
Movement defects |
Viability |
2D Ti2NTx Mxene |
DLS |
Zeta potential, hydrodynamic diameters |
Human malignant melanoma (A375) cell, spontaneously transformed aneuploid immortal keratinocyte cell (HaCaT), breast cancer cell (MCF-7), human female mammary glands (MCF-10A) |
Cytotoxicity |
2D Ti2NTx only toxic to cancer cells by generating intracellular reactive oxygen species |
40
|
Ti2AlN |
SEM |
Morphology |
ROS |
FFT |
Layered reflection |
Uptake |
HREM |
Structure |
IFFT |
Alternating layers |
EDX |
Elemental composition |
UV-vis-NIR spectrometer |
UV-vis-NIR absorption spectra |
FTIR |
Characteristic signals derived from bond vibrations |
AR-MX |
SEM |
Morphology |
Human malignant melanoma (A375) cell, spontaneously transformed aneuploid immortal keratinocyte cell (HaCaT), breast cancer cell (MCF-7), human female mammary glands (MCF-10A) |
Viability |
Proposing that reactive oxygen species generation is one mechanism of toxicity and surface-Ti2O3 also recognized as a key factor inducing oxidative stress |
41
|
SO-MX |
UV-VIS |
UV-vis-NIR absorption spectra |
ROS |
TO-MX |
FTIR |
Characteristic signals derived from bond vibrations |
XPS |
Presence of elements |
DLS |
Zeta potential |
Ti3C2 QDs |
TEM |
Diameter |
Human umbilical vein endothelial cells (HUVEC) |
Cytotoxicity |
Autophagic dysfunction leads to higher accumulation of pro-caspase 3 |
42
|
Nb2C QDs |
AFM |
Thickness |
Inflammation |
HUVECs |
Intracellular concentration |
Autophagy |
Apoptosis |
Ti3C2 Mxene |
SEM |
Thickness |
Human malignant melanoma (A375) cell, spontaneously transformed aneuploid immortal keratinocyte cell (HaCaT), adenocarcinomic human alveolar basal epithelial cell (A549), Human fetal lung fibroblast cell (MRC-5) |
Viability |
Proposing reactive oxygen species generation as a possible mechanism of the cytotoxicity of 2D sheets of Ti3C2 |
9
|
TEM |
Structure |
ROS |
HREM |
Structure |
FFT |
Layered reflection |
DLS |
Zeta potential, particle size |
XPS |
Presence of elements |
In the MXene studies, seven out of nine studies are biomedical application studies, breast cancer-related cells (MCF-7 and MCF-10A) were used in four studies,37,39–41 skin cancer-related cells (HaCaT and A375) were used in four studies,9,36,40,41 and kidney cells (HEK-293) and fibroblast cells (L929) were also used.35,38 Alternative model species such as zebrafish embryos and bacteria have also been used.38,39 For the toxicity endpoint, cell viability was tested in all studies, ROS measurement was performed in three studies,9,40,41 and inflammatory response tests were performed in two studies.38,42 Uptake, cellular cycle, photothermal effect, skin recovery, granulation tissue thickness, collagen content, autophagy, and apoptosis were also measured.36–38,40,42
While extensive research has been conducted on the characteristics of MAX phases for diverse applications, investigations regarding their biocompatibility and toxicity remain scarce. A significant amount of research exploring biocompatibility is focused exclusively on MXenes. However, the lack of toxicity information on the MAX phase, the precursor of MXene, may hinder the development and utilization of various MXene-based products. Hence, this highlights the crucial importance of conducting comprehensive biocompatibility and toxicity studies on MAX phases, as they are lagging in research priority compared to their intrinsic value. In addition, gaining insight into the toxicity would greatly facilitate their utilization, particularly in the development of safe materials through an SbD approach. Therefore, in this study, toxicity screening assays were performed to ascertain a broader range of toxicity associated with MAX phases.
3.2. Physicochemical properties
MAX phases, Ti2AlC and Ti3AlC2, were characterized by SEM, AFM, EDS, XPS, and XRD before the toxicity test to assess the physicochemical properties of nanomaterials in the test media (Fig. 1). Fig. 1(a) shows the SEM images of the Ti2AlC and Ti3AlC2 particles before and after ultrasonication. Before ultrasonication, the sizes of the bulk Ti2AlC and Ti3AlC2 particles ranged from a few micrometers to tens of micrometers. After ultrasonication, the Ti2AlC and Ti3AlC2 particles were in the order of hundreds of nanometers in size. Fig. 1(b) and (c) show AFM images of the Ti2AlC and Ti3AlC2 particles, respectively, to determine the thickness of the particles. The thickness of the particles ranged from 5 to 10 nm. Fig. 1(d) presents the EDS spectrum of the Ti2AlC and Ti3AlC2 particles. Strong peaks corresponding to Ti, Al, and C were observed. XPS was conducted to identify the bonding on the surface of the MAX phase particles, as shown in Fig. 1(e). Ti2AlC and Ti3AlC2 particles exhibited similar spectral features over the entire binding energy range. The binding energies of Ti 2p, Al 2p, and C 1s were 453, 71, and 281 eV, respectively.43–45Fig. 1(f) shows the measured XRD pattern of the Ti2AlC and Ti3AlC2 samples. The Ti2AlC XRD pattern exhibited nine XRD peaks at 2θ = 12.95° (002), 26.1° (004), 33.9° (100), 39.45° (103), 43.35° (104), 53.1° (106), 60.75° (110), 71.75° (109), and 74.95° (116).46 The Ti3AlC2 sample showed 14 peaks at 2θ = 9.54° (002), 19.14° (004), 34.02° (101), 36.79° (103), 39° (104), 41.74° (105), 44.96° (106), 48.5° (107), 52.56° (108), 56.42° (109), 60.2° (110), 65.48° (1011), 70.4° (2021), and 74.12° (2024).46,47 The highly intense, sharp (002) peak indicates that the MAX phase particles had a high degree of crystallinity. The physicochemical properties of GO were analyzed in a previous study,14 and the methods and results are provided as ESI† (Table S1).
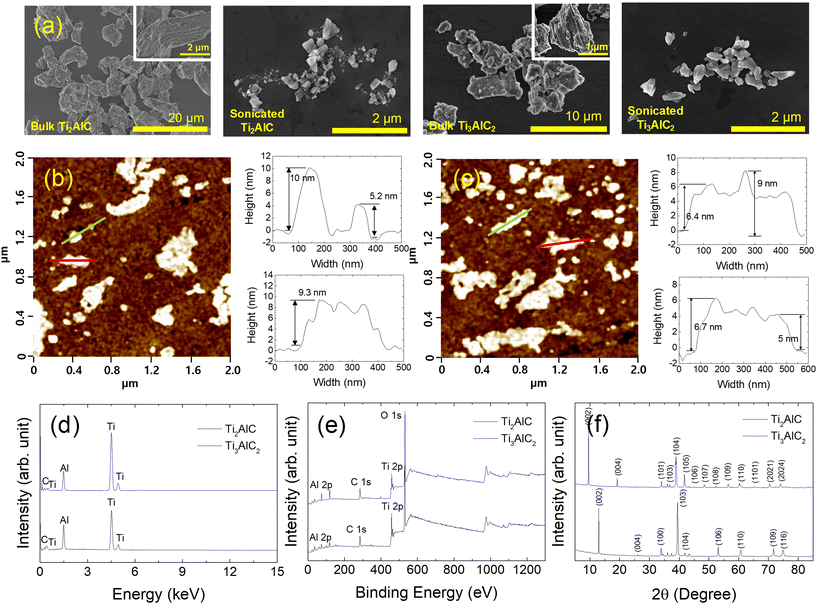 |
| Fig. 1 (a) Measured SEM images of bulk and sonicated Ti2AlC and Ti3AlC2 particles. Measured AFM images of (b) Ti2AlC particles and (c) Ti3AlC2 particles. Measured (d) EDS spectrum and (e) XPS spectra, and (f) XRD patterns of Ti2AlC and Ti3AlC2 particles. | |
The dispersion stability of the MAX phases (Ti2AlC and Ti3AlC2) was determined by DLS in the test media for cytotoxicity and ecotoxicity (Fig. 2). DLS was conducted immediately after spiking (time 0) and 24 h after spiking. The measured values of the absolute zeta potential were small (<−10 mV) for MAX phases in the tested media, except for Ti3AlC2, at 0 h after spiking. The DLS results of each medium collectively suggest that the colloidal dispersion stability of MAX phases and GO showed similar fluctuations over time in all media tested, except for 0 h Ti3AlC2.
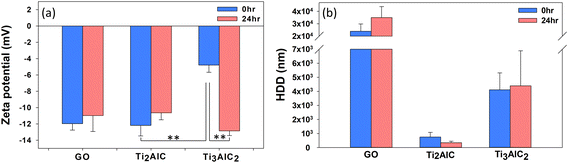 |
| Fig. 2 (a) Zeta potential and (b) hydrodynamic diameter of MAX phases (Ti2AlC and Ti3AlC2) in the test media for cytotoxicity and ecotoxicity. Graphene oxide (GO) was used as a reference material. | |
3.3. Cytotoxicity of MAX phases
The effects of the two MAX phases on human health were first screened by investigating their cytotoxicity using BEAS-2B cells at various time points (2, 4, 6, 8, 12, and 24 h) (Fig. 3). The cytotoxicity demonstrated a significant time-dependent increase within the first 6 h but showed less pronounced time dependency thereafter. Ti3AlC2 induced more severe cytotoxicity than Ti2AlC in the first 6 h, whereas Ti2AlC was more cytotoxic than Ti3AlC2 at 24 h. Overall, MAX phases and GO induced cytotoxicity within the range of 10–20%, and there were no significant differences in cytotoxicity among them (Fig. 3).
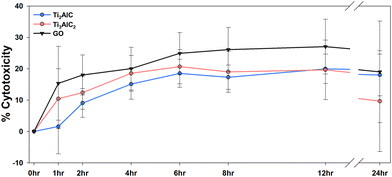 |
| Fig. 3 Cytotoxicity of MAX phases (Ti2AlC and Ti3AlC2) to BEAS-2B cells. Graphene oxide (GO) was used as a reference material. The cells were exposed to MAX phases and GO at 1 mg L−1 for different time points and % cytotoxicity determined by RealTime-Glo™ MT cell viability assay in DMEM F/12 medium. The experiments were performed for three individual replications, each with two technical replicates. The data are presented as the mean ± standard error of the mean. | |
The cytotoxicity of MAX phases and MXenes has been previously investigated. According to Scheibe et al., Ti2AlC and Ti3AlC2 exhibited cytotoxicity in normal human fibroblast (MSU1.1) cells at concentrations equal to or greater than 50 μg mL−1.33 Gu et al. confirmed cytotoxicity in HUVECs cells at concentrations equal to or greater than 50 mg L−1 for Ti3C2 MXene.42 Similarly, Rozmysłowska-Wojciechowska et al. observed cytotoxicity in HaCaT and A375 cells exposed to Ti3C2 MXenes at concentrations of 500 mg L−1.38 In this study, we exposed MAX phases at a concentration of 1 mg L−1, resulting in approximately 20% cytotoxicity. Therefore, this concentration has been established as suitable for conducting subsequent toxicity investigations.
3.4. Genotoxic potential of MAX phases
Oxidative stress is known to be an initial toxic effect caused by exposure to various nanomaterials,48,49 which can lead to subsequent toxic effects such as inflammation, DNA damage, and more. In particular, it is well-established as an upstream event in genotoxicity.50,51 As evident from the literature analysis (Table 2), most toxicity studies on MAX phases and MXenes have measured ROS and oxidative stress. Hence, this study aims to investigate the potential genotoxic capability of MAX phases.
A tiered approach was used, combining comet assay for DNA double-strand breaks and previously established C. elegans functional genetic tool52 for DNA repair capacity. The direct DNA damage in BEAS-2B cells was then investigated using alkaline comet assays (Fig. 4(a)). The olive tail moment, a DNA strand break marker, increased significantly in BEAS-2B cells exposed to both MAX phases (Ti2AlC and Ti3AlC2). This result collectively suggested that MAX phases had genotoxic potential in BEAS-2B cells, and their intensity was comparable to that of GO.
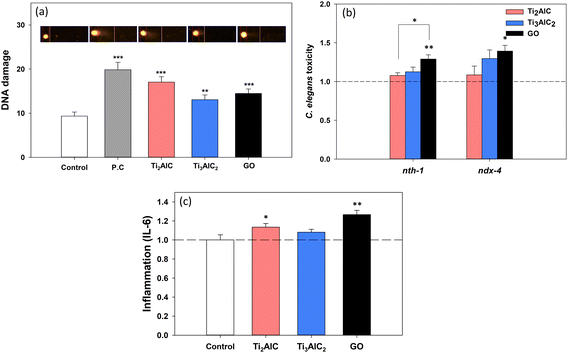 |
| Fig. 4 DNA damage and inflammation of MAX phases. Graphene oxide (GO) was used as a reference material. (a) DNA damage measured in MAX phases (Ti2AlC and Ti3AlC2) and GO exposed BEAS-2B cells using the comet assay. MAX phases and GO were exposed at 1 mg L−1 for 24 h. Pb2+ was used as the positive control. (b) Response of C. elegans DNA repair enzyme gene mutants to MAX phases (Ti2AlC and Ti3AlC2) and GO exposure. MAX phases and GO were exposed at 1 mg L−1 for 72 h. (c) Expression of inflammation marker IL-6 in MAX phases and GO exposed BEAS-2B cells. MAX phases and GO were exposed at 1 mg L−1 for 24 h. All results are shown as the mean ± standard error of the mean. *p < 0.05, **p < 0.01 compared to the control (one-way analysis of variance). | |
The DNA repair pathways in C. elegans are evolutionarily conserved in humans.53–55 The response of the DNA repair enzyme gene (nth-1 and ndx-4) loss-of-function mutants was compared with that of wild-type N2 (Fig. 4(b)). In nth-1 and ndx-4 mutants, increased toxicity was observed after GO exposure, suggesting that the BER pathway was required for defense against GO. Therefore, GO might cause genotoxicity, which is consistent with previous studies.14,56,57 The nth-1 and ndx-4 are both involved in the base-excision repair (BER) of DNA damage, which is often caused by oxidative stress.58,59nth-1 is a C. elegans homolog of human endonuclease III (NTH) and a well-conserved DNA glycosylase that removes oxidized pyrimidine bases,60 and ndx-4 has a MutT-type function that can hydrolyze 8-oxodGTP.61 The toxicity of the BER-defective mutants increased with exposure to the MAX phases, particularly with Ti3AlC2, therefore, the toxicity mechanism of MAX phases might be involved in the BER pathway. Although the response of Ti3AlC2 was slightly higher than that of Ti2AlC, the difference was not statistically significant. Both MAX phases showed lower genotoxic potential than GO in C. elegans.
In order to elucidate the underlying mechanism of genotoxicity, inflammation was assessed as it facilitates DNA repair in response to DNA double-strand breaks.62,63 IL-6, a proinflammatory cytokine marker was investigated in BEAS-2B cells exposed to the MAX phases (Fig. 4(c)). A statistically significant increase in the IL-6 level was observed in the Ti2AlC exposure group; however, no significant difference was found in the Ti3AlC2 group. IL-6, a cytokine associated with oxidative stress,64 has been reported to be linked to genotoxicity in various nanomaterials such as graphene and carbon black.65,66 Therefore, we hypothesized that the genotoxicity induced by MAX phases in BEAS-2B cells could be attributed to augmented ROS production,9,33,40,41 as well as inflammatory responses, leading to secondary genotoxic effects.66 A wide range of molecular and biochemical stress markers should be investigated to gain insight into the consequences of DNA damage at the cellular level.
3.5. Ecotoxicity of MAX phases
Most studies investigating the toxicity of MAX phases have primarily focused on human health, while there is a notable dearth of research on ecotoxicity based on our current knowledge (Table 2). Considering the potential for environmental exposure to MAX phases, it is imperative to conduct studies addressing ecotoxicity. To gain insight into the potential environmental hazards of MAX phases, a battery assay was performed using representative soil and aquatic model organisms, C. elegans and zebrafish (Fig. 5). Exposure to MAX phases did not cause any significant toxicity in C. elegans (Fig. 5(a)) and zebrafish embryo (Fig. 5(b)) at a concentration of 1 mg L−1.
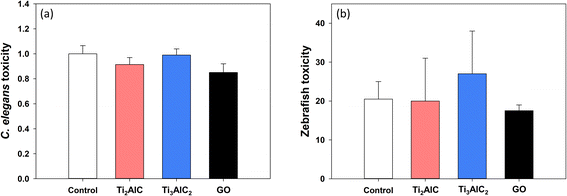 |
| Fig. 5 Number of offspring (a) in MAX phases exposed C. elegans. (b) Mortality of zebrafish embryo exposed to MAX phases. Graphene oxide (GO) was used as a reference material. MAX phases and GO were exposed for 96 h at 1 mg L−1. | |
MAX phases might induce genotoxicity and cytotoxicity in a human cell line, but not at the whole organism level. It is important to note that these results should not be interpreted as MAX phases not causing ecotoxicity, as toxicity generally occurs at lower concentrations at the molecular level than at the individual level.67 One possible explanation for these results could be the differences in the uptake of the MAX phases, resulting in varying concentrations within each biological system. In our study, both cells and model organisms were exposed indirectly through liquid media. However, zebrafish embryos benefit from the protection of the chorion, and C. elegans necessitates ingestion for material to enter their bodies. Although our research did not directly confirm the uptake, given the nano-sized nature of MAX phase particles, it is reasonable to assume significant uptake. Comparing the sizes of MAX phases (less than 1 μm for Ti2AlC and around 4 μm for Ti3AlC2) to those of the reference material, GO with a hydrodynamic diameter of 20–40 μm, suggests that MAX phases are also likely to be internalized by organisms. Previous studies have demonstrated GO uptake by C. elegans68 and its ability to traverse the chorion of zebrafish embryos.69,70
Nevertheless, compared to cell-based assays, it is reasonable to assume that lower concentrations of the MAX phase reach the cell interior, potentially explaining the absence of toxicity at the organism level. Therefore, comprehensive research and understanding of the uptake and kinetics of MAX phases within living organisms are essential. Of course, one could interpret the absence of toxicity as a simple interspecies difference between humans and ecological species. Additionally, this study focused on short-term exposure effects, but given the observed toxicity at the cellular level, further investigations are necessary to examine the effects of long-term exposure at the organism level.
4. Considerations for SbD implementation
The expansion of MAX phase technology has led to a wide range of applications for these materials, owing to their unique metallic and ceramic properties. However, novel applications of MAX phases and its 2-dimensional derivative MXenes may introduce new hazards. This study has focused on assessing the potential hazards and toxicity mechanisms of two MAX phases, Ti2AlC and Ti3AlC2, for environmental health and safety assessments, highlighting their non-negligible hazardous potential compared to GO. Although generalization of the toxicity profile of the MAX phase should be avoided, these results suggest that significant attention should be paid to the mass production and application of the MAX phase. Implementing the SbD concept during the R&D or design phase of MAX phases and MXenes would proactively address these risks and contribute to sustainable development.
The first step of SbD involves utilizing less harmful substances based on their known toxicity.17 In our study, the results revealed that Ti3AlC2 exhibited lower level of cytotoxicity, DNA damage, and inflammatory response (IL-6) at 24 h in comparison to Ti2AlC. It is essential to recognize that nanomaterials such as MAX phases can exhibit variations in toxicity due to factors like composition, size, aspect ratio, and other physicochemical properties. However, there is a notable lack of comprehensive studies regarding the toxicity of MAX phases, making it difficult to identify specific factors that influence toxicity. The two materials examined in our study share very similar physicochemical properties. Nonetheless, Ti3AlC2 exhibited a hydrodynamic diameter approximately four times larger than Ti2AlC and a thinner profile, resulting in a higher aspect ratio. Previous studies have indicated that the relationship between toxicity and aspect ratio may vary depending on experimental conditions or nanomaterial type.71 Under the specific experimental conditions of our study, higher aspect ratios in MAX phases were associated with reduced toxicity. However, as this interpretation is based on a single characteristic, it would be prudent to draw comprehensive conclusions by considering a broader range of characteristics. Therefore, continued efforts should be made to compare the toxicity of MAX phases with various compositions and physicochemical properties using well-established experimental methods to identify safer materials with similar functionalities. Such research will serve as a valuable foundation for supporting the implementation of the SbD approach.
Key physicochemical properties influencing the toxicity of nanomaterials include lateral size, surface structure, functionalization, charge, and impurities.11,72,73 Changes in these properties may alter the toxicity, necessitating additional safety evaluations. To avoid the challenges of individually testing each change, it is crucial to establish the relationship between these properties and toxicity, enabling toxicity prediction without extensive experimental testing. Many studies have explored this relationship, leading to toxicity prediction models for nanomaterials.74–77 Developing an in silico model optimized for MAX phases based on these studies could significantly enhance the development of safer materials. Additional research in this direction could further advance the SbD approach for MAX phases.
Exposure is also a critical factor influencing the toxicity of nanomaterials. In our study, a concentration of 1 mg L−1 was used, which is relatively low compared to previous studies.33,38 As environmental concentration data for MAX phases are currently unavailable, we adopted this concentration based on a study setting the environmental concentration of GO.78 Thus, our experiment simulated low-exposure scenarios in the environment rather than high-exposure scenarios in an occupational accident. Since MAX phases are still in the development stage, the current exposure to MAX phases mainly occurs through inhalation in the workplace, so future research should focus on inhalation exposure and toxicity. For in vitro studies, an air–liquid interface system may be employed.79 In the long term, it is necessary to understand the potential exposure of MAX phases to humans and ecological species and determine the concentrations encountered during the manufacturing process and use in terms of a life cycle assessment.80
5. Conclusions
In this study, two types of MAX phase materials (Ti2AlC and Ti3AlC2) were characterized, and their cytotoxicity, genotoxicity, and ecotoxicity were investigated to gain insight into their potential toxicity, thus enabling the implementation of the SbD approach. Our study highlights the importance of assessing the potential hazards and toxicity mechanisms of MAX phases in the context of human and environmental safety. These findings provide valuable insights and a basis for implementing SbD approaches in the development and application of MAX phases for safer and environmentally conscious nanotechnology advancements.
Author contributions
Jaeseong Jeong: investigation, visualization, writing – original draft, writing – reviewing and editing. Su-Yong Bae: investigation, formal analysis, validation, writing – original draft. Jinho Lee: methodology, visualization, writing – original draft. Suh-Young Kwon: formal analysis, writing – original draft. Ju Han Lee: resources, writing – reviewing and editing. Jinhee Choi: supervision, conceptualization, writing – original draft, writing – reviewing and editing.
Conflicts of interest
There are no conflicts of interest to declare.
Acknowledgements
This work was supported by the Korean Ministry of Environment under the ‘Environmental Health R&D Program’ (2021003310005) and the National Research Foundation of Korea (NRF) grant funded by the Korea government (MSIT) (No. NRF-2021R1A5A1032937).
References
- M. Barsoum and T. El-Raghy, The MAX Phases: Unique New Carbide and Nitride Materials, Am. Sci., 2001, 89, 334 CrossRef.
- Z. M. Sun, Progress in research and development on MAX phases: A family of layered ternary compounds, Int. Mater. Rev., 2011, 56, 143–166 CrossRef CAS.
- M. Haftani, M. Saeedi Heydari, H. R. Baharvandi and N. Ehsani, Studying the oxidation of Ti2AlC MAX phase in atmosphere: A review, Int. J. Refract. Hard Met., 2016, 61, 51–60 CrossRef CAS.
- E. N. Hoffman, D. W. Vinson, R. L. Sindelar, D. J. Tallman, G. Kohse and M. W. Barsoum, MAX phase carbides and nitrides: Properties for future nuclear power plant in-core applications and neutron transmutation analysis, Nucl. Eng. Des., 2012, 244, 17–24 CrossRef CAS.
- J. W. Byeon, J. Liu, M. Hopkins, W. Fischer, N. Garimella, K. B. Park, M. P. Brady, M. Radovic, T. El-Raghy and Y. H. Sohn, Microstructure and residual stress of alumina scale formed on Ti2AlC at high temperature in air, Oxid. Met., 2007, 68, 97–111 CrossRef CAS.
- Z. Zhang, X. Duan, D. Jia, Y. Zhou and S. van der Zwaag, On the formation mechanisms and properties of MAX phases: A review, J. Eur. Ceram. Soc., 2021, 41, 3851–3878 CrossRef CAS.
- Y. Gogotsi and B. Anasori, The Rise of MXenes, ACS Nano, 2019, 13, 8491–8494 CrossRef CAS PubMed.
- H. Lin, Y. Chen and J. Shi, Insights into 2D MXenes for Versatile Biomedical Applications: Current Advances and Challenges Ahead, Adv. Sci., 2018, 5, 1800518 CrossRef.
- A. M. Jastrzębska, A. Szuplewska, T. Wojciechowski, M. Chudy, W. Ziemkowska, L. Chlubny, A. Rozmysłowska and A. Olszyna, In vitro studies on cytotoxicity of delaminated Ti3C2 MXene, J. Hazard. Mater., 2017, 339, 1–8 CrossRef.
- M. Naguib, M. Kurtoglu, V. Presser, J. Lu, J. Niu, M. Heon, L. Hultman, Y. Gogotsi and M. W. Barsoum, Two-dimensional nanocrystals produced by exfoliation of Ti3AlC2, Adv. Mater., 2011, 23, 4248–4253 CrossRef CAS.
- L. Ou, B. Song, H. Liang, J. Liu, X. Feng, B. Deng, T. Sun and L. Shao, Toxicity of graphene-family nanoparticles: A general review of the origins and mechanisms, Part. Fibre Toxicol., 2016, 13, 57 CrossRef.
- A. M. Jastrzębska, P. Kurtycz and A. R. Olszyna, Recent advances in graphene family materials toxicity investigations, J. Nanopart. Res., 2012, 14, 1320 CrossRef.
- N. Chatterjee, Y. Kim, J. Yang, C. P. Roca, S.-W. Joo and J. Choi, A systems toxicology approach reveals the Wnt-MAPK crosstalk pathway mediated reproductive failure in Caenorhabditis elegans exposed to graphene oxide (GO) but not to reduced graphene oxide (rGO), Nanotoxicology, 2017, 11, 76–86 CrossRef CAS PubMed.
- N. Chatterjee, J. S. Yang and J. Choi, Differential genotoxic and epigenotoxic effects of graphene family nanomaterials (GFNs) in human bronchial epithelial cells, Mutat. Res., Genet. Toxicol. Environ. Mutagen., 2016, 798–799, 1–10 CrossRef CAS.
- I. van de Poel and Z. Robaey, Safe-by-Design: from Safety to Responsibility, NANO, 2017, 11, 297–306 Search PubMed.
-
K. Savolainen, U. Backman, D. Brouwer, B. Fadeel and T. Fernandes, Nanosafety in Europe 2015-2025: Towards Safe and Sustainable Nanomaterials and Nanotechnology Innovations Nanosafety in Europe Towards Safe and Sustainable Nanomaterials and Nanotechnology Innovations, Nanosafety in Europe 2015-2025: Towards Safe and Sustainable Nanomaterials and Nanotechnology Innovations Nanosafety in Europe Towards Safe and Sustainable Nanomaterials and Nanotechnology Innovations, 2013 Search PubMed.
- A. Sánchez Jiménez, R. Puelles, M. Perez-Fernandez, L. Barruetabeña, N. R. Jacobsen, B. Suarez-Merino, C. Micheletti, N. Manier, B. Salieri, R. Hischier, R. Tsekovska, Y. Handzhiyski, J. Bouillard, Y. Oudart, K. S. Galea, S. Kelly, N. Shandilya, H. Goede, J. Gomez-Cordon, K. A. Jensen, M. van Tongeren, M. D. Apostolova and I. R. Llopis, Safe(r) by design guidelines for the nanotechnology industry, NanoImpact, 2022, 25, 100385 CrossRef.
- N. Chatterjee, H. Eom and J. Choi, A systems toxicology approach to the surface functionality control of graphene e cell interactions, Biomaterials, 2014, 35, 1109–1127 CrossRef CAS PubMed.
- S. Brenner, The genetics of Caenorhabditis elegans, Genetics, 1974, 77, 71–94 CrossRef CAS PubMed.
- J. Yang, N. Chatterjee, Y. Kim, J. Y. Roh, J. H. Kwon, M. S. Park and J. Choi, Histone methylation-associated transgenerational inheritance of reproductive defects in Caenorhabditis elegans exposed to crude oil under various exposure scenarios, Chemosphere, 2018, 200, 358–365 CrossRef CAS PubMed.
-
OECD, Test No. 236: Fish Embryo Acute Toxicity (FET) Test, 2013 Search PubMed.
- M. W. Barsoum, The MN+1AXN phases: A new class of solids, Prog. Solid State Chem., 2000, 28, 201–281 CrossRef CAS.
- L. Boatemaa, M. Bosch, A. S. Farle, G. P. Bei, S. van der Zwaag and W. G. Sloof, Autonomous high-temperature healing of surface cracks in Al2O3 containing Ti2AlC particles, J. Am. Ceram. Soc., 2018, 101, 5684–5693 CrossRef CAS.
- W. Wang, F. Ye, W. Mu, J. Dutta and B. Laumert, A New High-Temperature Durable Absorber Material Solution through a Spinel-Type High Solar Absorptivity Coating on Ti2AlC MAX Phase Material, ACS Appl. Mater. Interfaces, 2021, 13, 45008–45017 CrossRef CAS PubMed.
- J. Gonzalez-Julian, Processing of MAX phases: From synthesis to applications, J. Am. Ceram. Soc., 2021, 104, 659–690 CrossRef CAS.
- K. R. Whittle, M. G. Blackford, R. D. Aughterson, S. Moricca, G. R. Lumpkin, D. P. Riley and N. J. Zaluzec, Radiation tolerance of Mn+1AXn phases, Ti3AlC2 and Ti3SiC2, Acta Mater., 2010, 58, 4362–4368 CrossRef CAS.
- M. Radovic and M. W. Barsoum, MAX phases: bridging the gap between metals and ceramics, Am. Ceram. Soc. Bull., 2013, 92, 20–27 CAS.
- S. Omar, A. H. A. Rosol, A. A. A. Jafry, N. F. Zulkipli, Z. Jusoh, B. Musa, M. Yasin and S. W. Harun, Ti3AlC2 MAX phase thin film as saturable absorber for generating soliton mode-locked fiber laser, Optik, 2021, 245, 167767 CrossRef CAS.
- J. Lee, S. Kwon and J. H. Lee, Ti2AlC-based saturable absorber for passive Q-switching of a fiber laser, Opt. Mater. Express, 2019, 9, 2057 CrossRef CAS.
- H. Ahmad, H. S. M. Albaqawi, N. Yusoff, W. Y. Chong and M. Yasin, Q-Switched Fiber Laser at 1.5 μm Region Using Ti3AlC2 MAX Phase-Based Saturable Absorber, IEEE J. Quantum Electron., 2020, 56, 1600106 CAS.
- W. H. K. Ng, E. S. Gnanakumar, E. Batyrev, S. K. Sharma, P. K. Pujari, H. F. Greer, W. Zhou, R. Sakidja, G. Rothenberg, M. W. Barsoum and N. R. Shiju, The Ti3 AlC2 MAX Phase as an Efficient Catalyst for Oxidative Dehydrogenation of n-Butane, Angew. Chem., 2018, 130, 1501–1506 CrossRef.
- K. Wang, H. Du, Z. Wang, M. Gao, H. Pan and Y. Liu, Novel MAX-phase Ti3AlC2 catalyst for improving the reversible hydrogen storage properties of MgH2, Int. J. Hydrogen Energy, 2017, 42, 4244–4251 CrossRef CAS.
- B. Scheibe, J. K. Wychowaniec, M. Scheibe, B. Peplińska, M. Jarek, G. Nowaczyk and Ł. Przysiecka, Cytotoxicity Assessment of Ti-Al-C Based MAX Phases and Ti3C2Tx MXenes on Human Fibroblasts and Cervical Cancer Cells, ACS Biomater. Sci. Eng., 2019, 5, 6557–6569 CrossRef CAS.
- H. Jiang, Y. Li, Q. Jin, D. Yang, C. Wu and J. Cui, Physiological and biochemical effects of Ti3AlC2 nanosheets on rice (Oryza sativa L.), Sci. Total Environ., 2021, 770, 145340 CrossRef CAS.
- H. Rastin, B. Zhang, A. Mazinani, K. Hassan, J. Bi, T. T. Tung and D. Losic, 3D bioprinting of cell-laden electroconductive MXene nanocomposite bioinks, Nanoscale, 2020, 12, 16069–16080 RSC.
- E. A. Hussein, M. M. Zagho, B. R. Rizeq, N. N. Younes, G. Pintus, K. A. Mahmoud, G. K. Nasrallah and A. A. Elzatahry, Plasmonic MXene-based nanocomposites exhibiting photothermal therapeutic effects with lower acute toxicity than pure MXene, Int. J. Nanomed., 2019, 14, 4529–4539 CrossRef CAS.
- S. Lin, H. Lin, M. Yang, M. Ge, Y. Chen and Y. Zhu, A two-dimensional MXene potentiates a therapeutic microneedle patch for photonic implantable medicine in the second NIR biowindow, Nanoscale, 2020, 12, 10265–10276 RSC.
- A. Rozmysłowska-Wojciechowska, J. Mitrzak, A. Szuplewska, M. Chudy, J. Woźniak, M. Petrus, T. Wojciechowski, A. S. Vasilchenko and A. M. Jastrzębska, Engineering of 2D Ti3C2 MXene Surface Charge and its Influence on Biological Properties, Materials, 2020, 13, 2347 CrossRef.
- L. Zhou, H. Zheng, Z. Liu, S. Wang, Z. Liu, F. Chen, H. Zhang, J. Kong, F. Zhou and Q. Zhang, Conductive Antibacterial Hemostatic Multifunctional Scaffolds Based on Ti3C2Tx MXene Nanosheets for Promoting Multidrug-Resistant Bacteria-Infected Wound Healing, ACS Nano, 2021, 15, 2468–2480 CrossRef CAS.
- A. Szuplewska, A. Rozmysłowska-Wojciechowska, S. Poźniak, T. Wojciechowski, M. Birowska, M. Popielski, M. Chudy, W. Ziemkowska, L. Chlubny, D. Moszczyńska, A. Olszyna, J. A. Majewski and A. M. Jastrzębska, Multilayered stable 2D nano-sheets of Ti2NTx MXene: synthesis, characterization, and anticancer activity, J. Nanobiotechnol., 2019, 17, 114 CrossRef CAS.
- A. M. Jastrzębska, A. Szuplewska, A. Rozmysłowska-Wojciechowska, M. Chudy, A. Olszyna, M. Birowska, M. Popielski, J. A. Majewski, B. Scheibe, V. Natu and M. W. Barsoum, On tuning the cytotoxicity of Ti3C2 (MXene) flakes to cancerous and benign cells by post-delamination surface modifications, 2D Mater., 2020, 7, 025018 CrossRef.
- M. Gu, Z. Dai, X. Yan, J. Ma, Y. Niu, W. Lan, X. Wang and Q. Xu, Comparison of toxicity of Ti3C2 and Nb2C Mxene quantum dots (QDs) to human umbilical vein endothelial cells, J. Appl. Toxicol., 2021, 41, 745–754 CrossRef CAS PubMed.
- Z. Zhang, S. H. Lim, D. M. Y. Lai, S. Y. Tan, X. Q. Koh, J. Chai, S. J. Wang, H. Jin and J. S. Pan, Probing the oxidation behavior of Ti2AlC MAX phase powders between 200 and 1000 °C, J. Eur. Ceram. Soc., 2017, 37, 43–51 CrossRef CAS.
- F. Kong, K. Feng, Y. Bai, N. Li, X. Qi, Y. Zheng, R. Wang and X. He, Oxidation behavior of high-purity nonstoichiometric Ti2AlC powders in flowing air, J. Mater. Res., 2017, 32, 2747–2754 CrossRef CAS.
- M. Zhu, R. Wang, C. Chen, H. B. Zhang and G. J. Zhang, Comparison of corrosion behavior of Ti3SiC2 and Ti3AlC2 in NaCl solutions with Ti, Ceram. Int., 2017, 43, 5708–5714 CrossRef CAS.
- B. Scheibe, V. Kupka, B. Peplińska, M. Jarek and K. Tadyszak, The Influence of Oxygen Concentration during MAX Phases (Ti3AlC2) Preparation on the αAl2O3 Microparticles Content and Specific Surface Area of Multilayered MXenes (Ti3C2Tx), Materials, 2019, 12, 353 CrossRef CAS.
- S. Wang, J. Ma, S. Zhu, J. Cheng, Z. Qiao, J. Yang and W. Liu, High temperature tribological properties of Ti3AlC2 ceramic against SiC under different atmospheres, Mater. Des., 2015, 67, 188–196 CrossRef CAS.
- A. Manke, L. Wang and Y. Rojanasakul, Mechanisms of nanoparticle-induced oxidative stress and toxicity, BioMed Res. Int., 2013, 2013, 942916 Search PubMed.
- P. P. Fu, Q. Xia, H. M. Hwang, P. C. Ray and H. Yu, Mechanisms of nanotoxicity: Generation of reactive oxygen species, J. Food Drug Anal., 2014, 22, 64–75 CrossRef CAS.
- J. Karpeta-Kaczmarek, M. Dziewięcka, M. Augustyniak, M. Rost-Roszkowska and M. Pawlyta, Oxidative stress and genotoxic effects of diamond nanoparticles, Environ. Res., 2016, 148, 264–272 CrossRef CAS.
- M. Hanot-Roy, E. Tubeuf, A. Guilbert, A. Bado-Nilles, P. Vigneron, B. Trouiller, A. Braun and G. Lacroix, Oxidative stress pathways involved in cytotoxicity and genotoxicity of titanium dioxide (TiO2) nanoparticles on cells constitutive of alveolo-capillary barrier in vitro, Toxicol. In Vitro, 2016, 33, 125–135 CrossRef CAS.
- N. Chatterjee, H. J. Eom and J. Choi, Effects of silver nanoparticles on oxidative DNA damage-repair as a function of p38 MAPK status: A comparative approach using human Jurkat T cells and the nematode Caenorhabditis elegans, Environ. Mol. Mutagen., 2014, 55, 122–133 CrossRef CAS.
- A. Gartner, S. Milstein, S. Ahmed, J. Hodgkin and M. O. Hengartner, A conserved checkpoint pathway mediates DNA damage-induced apoptosis and cell cycle arrest in C. elegans, Mol. Cell, 2000, 5, 435–443 CrossRef CAS PubMed.
- L. Stergiou and M. O. Hengartner, Death and more: DNA damage response pathways in the nematode C. elegans, Cell Death Differ., 2004, 11, 21–28 CrossRef CAS.
- M. Rieckher, A. Bujarrabal, M. A. Doll, N. Soltanmohammadi and B. Schumacher, A simple answer to complex questions: Caenorhabditis elegans as an experimental model for examining the DNA damage response and disease genes, J. Cell. Physiol., 2017, 233, 2781–2790 CrossRef PubMed.
- L. De Marzi, L. Ottaviano, F. Perrozzi, M. Nardone, S. Santucci, J. De Lapuente, M. Borras, E. Treossi, V. Palermo and A. Poma, Flake size-dependent cyto and genotoxic evaluation of graphene oxide on in vitro A549, CaCo2 and vero cell lines, J. Biol. Regul. Homeostatic Agents, 2014, 28, 281–289 CAS.
- M. Hinzmann, S. Jaworski, M. Kutwin, J. Jagiełło, R. Koziński, M. Wierzbicki, M. Grodzik, L. Lipińska, E. Sawosz and A. Chwalibog, Nanoparticles containing allotropes of carbon have genotoxic effects on glioblastoma multiforme cells, Int. J. Nanomed., 2014, 9, 2409–2417 Search PubMed.
- S. Maynard, S. H. Schurman, C. Harboe, N. C. de Souza-Pinto and V. A. Bohr, Base excision repair of oxidative DNA damage and association with cancer and aging, Carcinogenesis, 2009, 30, 2–10 CrossRef CAS PubMed.
- J. Cadet and K. J. A. Davies, Oxidative DNA damage & repair: An introduction, Free Radical Biol. Med., 2017, 107, 2–12 CrossRef CAS.
- H. Morinaga, S. I. Yonekura, N. Nakamura, H. Sugiyama, S. Yonei and Q. M. Zhang-Akiyama, Purification and characterization of Caenorhabditis elegans NTH, a homolog of human endonuclease III: Essential role of N-terminal region, DNA Repair, 2009, 8, 844–851 CrossRef CAS PubMed.
- K. D. Arczewska, C. Baumeier, H. Kassahun, T. SenGupta, M. Bjørås, J. T. Kuśmierek and H. Nilsen, Caenorhabditis elegans NDX-4 is a MutT-type enzyme that contributes to genomic stability, DNA Repair, 2011, 10, 176–187 CrossRef CAS.
- F. Rodier, J. P. Coppé, C. K. Patil, W. A. M. Hoeijmakers, D. P. Muñoz, S. R. Raza, A. Freund, E. Campeau, A. R. Davalos and J. Campisi, Persistent DNA damage signalling triggers senescence-associated inflammatory cytokine secretion, Nat. Cell Biol., 2009, 11, 973–979 CrossRef CAS PubMed.
- Y. Chen, F. Zhang, Y. Tsai, X. Yang, L. Yang, S. Duan, X. Wang, P. Keng and S. O. Lee, IL-6 signaling promotes DNA repair and prevents apoptosis in CD133+ stem-like cells of lung cancer after radiation, Radiat. Oncol., 2015, 10, 1–10 CrossRef PubMed.
- S. Wassmann, M. Stumpf, K. Strehlow, A. Schmid, B. Schieffer, M. Böhm and G. Nickenig, Interleukin-6 Induces Oxidative Stress and Endothehal Dysfunction by Overexpression of the Angiotensin II Type 1 Receptor, Circ. Res., 2004, 94, 534–541 CrossRef CAS.
- E. Di Ianni, P. Møller, T. Cholakova, H. Wolff, N. R. Jacobsen and U. Vogel, Assessment of primary and inflammation-driven genotoxicity of carbon black nanoparticles in vitro and in vivo, Nanotoxicology, 2022, 16, 526–545 CrossRef CAS PubMed.
- M. J. Burgum, M. J. D. Clift, S. J. Evans, N. Hondow, M. Miller, S. B. Lopez, A. Williams, A. Tarat, G. J. Jenkins and S. H. Doak, In Vitro Primary-Indirect Genotoxicity in Bronchial Epithelial Cells Promoted by Industrially Relevant Few-Layer Graphene, Small, 2021, 17, 1–11 CrossRef PubMed.
- N. L. Chepelev, M. E. B. Meek and C. L. Yauk, Application of benchmark dose modeling to protein expression data in the development and analysis of mode of action/adverse outcome pathways for testicular toxicity, J. Appl. Toxicol., 2014, 34, 1115–1121 CrossRef CAS.
- Y. Kim, J. Jeong, J. Yang, S.-W. Joo, J. Hong and J. Choi, Graphene oxide nano-bio interaction induces inhibition of spermatogenesis and disturbance of fatty acid metabolism in the nematode Caenorhabditis elegans, Toxicology, 2018, 410, 83–95 CrossRef CAS.
- Y. Chen, X. Hu, J. Sun and Q. Zhou, Specific nanotoxicity of graphene oxide during zebrafish embryogenesis, Nanotoxicology, 2016, 10, 42–52 CAS.
- Z. Wang and Z. Wang, Nanoparticles induced embryo–fetal toxicity, Toxicol. Ind. Health, 2020, 36, 181–213 CrossRef.
- S. Shukla, F. J. Eber, A. S. Nagarajan, N. A. Difranco, N. Schmidt, A. M. Wen, S. Eiben, R. M. Twyman, C. Wege and N. F. Steinmetz, The impact of aspect ratio on the biodistribution and tumor homing of rigid soft-matter nanorods, Adv. Healthcare Mater., 2015, 4, 874–882 CrossRef CAS PubMed.
- S. Mittal, V. Kumar, N. Dhiman, L. K. S. Chauhan, R. Pasricha and A. K. Pandey, Physico-chemical properties based differential toxicity of graphene oxide/reduced graphene oxide in human lung cells mediated through oxidative stress, Sci. Rep., 2016, 6, 39548 CrossRef CAS PubMed.
- N. Chatterjee, H. J. Eom and J. Choi, A systems toxicology approach to the surface functionality control of graphene-cell interactions, Biomaterials, 2014, 35, 1109–1127 CrossRef CAS PubMed.
- C. Helma, M. Rautenberg and D. Gebele, Nano-Lazar: Read across predictions for nanoparticle toxicities with calculated and measured properties, Front. Pharmacol., 2017, 8, 1–11 Search PubMed.
- D. A. Winkler, Role of Artificial Intelligence and Machine Learning in Nanosafety, Small, 2020, 16, 2001883 CrossRef CAS PubMed.
- A. V. Singh, D. Rosenkranz, M. H. D. Ansari, R. Singh, A. Kanase, S. P. Singh, B. Johnston, J. Tentschert, P. Laux and A. Luch, Artificial Intelligence and Machine Learning Empower Advanced Biomedical Material Design to Toxicity Prediction, Adv. Intell. Syst., 2020, 2, 2000084 CrossRef.
- J. Jeong and J. Choi, Quantitative adverse outcome pathway (qAOP) using bayesian network model on comparative toxicity of multi-walled carbon nanotubes (MWCNTs): safe-by-design approach, Nanotoxicology, 2022, 1–16 Search PubMed.
- X. Zhang, Q. Zhou, W. Zou and X. Hu, Molecular Mechanisms of Developmental Toxicity Induced by Graphene Oxide at Predicted Environmental Concentrations, Environ. Sci. Technol., 2017, 51, 7861–7871 CrossRef CAS PubMed.
- S. Upadhyay and L. Palmberg, Air-liquid interface: Relevant in vitro models for investigating air pollutant-induced pulmonary toxicity, Toxicol. Sci., 2018, 164, 21–30 CrossRef CAS.
- B. Salieri, D. A. Turner, B. Nowack and R. Hischier, Life cycle assessment of manufactured nanomaterials: Where are we?, NanoImpact, 2018, 10, 108–120 CrossRef.
|
This journal is © The Royal Society of Chemistry 2024 |