Comparative analysis of chemically and green synthesized titanium dioxide nanoparticles for the regulation of photosynthesis in Lactuca sativa L.†
Received
29th August 2023
, Accepted 9th November 2023
First published on 16th November 2023
Abstract
Green synthesized titanium dioxide nanoparticles (g-TiO2 NPs) have aroused widespread interest in agriculture. Nevertheless, whether they are safer than chemically synthesized TiO2 NPs (c-TiO2 NPs) remains to be demonstrated. Herein, the photosynthetic response of Lactuca sativa L. was evaluated by foliar spraying of 10, 100, 250, and 500 mg L−1 c-TiO2 NPs or g-TiO2 NPs. Results indicated that NPs interfered with nutrient accumulation and the cellular redox state, and all treatments displayed inhibition of growth except the 10 mg L−1 g-TiO2 NP group. Photosynthetic parameters and FTIR analysis revealed that NP stress on photosynthesis was also manifested by lower photosystem activity (500 mg L−1) and disrupted Calvin cycle metabolism, but carbohydrates and proteins were more sensitive to c-TiO2 NPs and g-TiO2 NPs, respectively. Notably, both NPs promoted photosynthetic electron transfer (≤250 mg L−1), thus alleviating detrimental effects due to suppressed ATPase and Rubisco activity, thylakoid lysis, and chloroplast autophagy. Differently, chloroplasts were more responsive to ultraviolet (non-directly utilizable) and visible light under c-TiO2 NP treatment, which facilitated the Hill reaction while posing a photo-oxidative risk to plants. In contrast, g-TiO2 NPs were less phytotoxic, as evidenced by higher NADPH, ATPase activity, chlorophyll, and photosynthetic efficiency, and less chloroplast damage, where 10 mg L−1 g-TiO2 NPs effectively activated the plant defense system and improved light capture and conversion. Collectively, g-TiO2 NPs showed lower phytotoxicity by modulating energy conversion processes in chloroplasts, which provided a cutting-edge research perspective for the application of nanopesticides.
Environmental significance
Recently, green synthetic nanotechnology with cost and environmental benefits has attracted great attention. Chemically synthesized TiO2 nanoparticles (c-TiO2 NPs) can stress plants when improving plant growth and the soil environment, but it is unclear whether green synthesized TiO2 (g-TiO2) NPs can mitigate these nanotoxicities. Herein, the effects of c-TiO2 NPs and g-TiO2 NPs on lettuce photosynthesis were explored by foliar spraying. The results indicated that both NPs facilitated photosynthetic electron transfer, while interference with energy synthesis and defense systems attenuated this advantage. In contrast, g-TiO2 NPs demonstrated lower toxicity to photosynthesis by modulating light harvesting, phototransformation, and chloroplast activity. These findings revealed the potential of g-TiO2 NPs in agricultural development and provided new insights into the risk assessment of environment-friendly nanoparticles.
|
1. Introduction
Nanotechnology is considered one of the most prospective technologies of the 21st century,1 and its core building blocks, nanoparticles (NPs), are extensively applied in industrial and medical fields due to their special physicochemical and optoelectronic properties.2 In recent years, NPs have also brought great promise for the development of agriculture, in particular the exploitation of nanopesticides and nanofertilizers not only reduced the overuse of traditional pesticides (20–30%)3 and the pressure on the environment, but also improved the efficiency of agricultural inputs and the nutritional value of crops (2.8–18%).4 It has been reported that Ag-based, Ti-based, and Cu-based nanomaterials are the commonest analyzed metal-based nanopesticides, where titanium dioxide (TiO2) NPs with excellent photocatalytic properties, high stability, and low cost are widely used as antimicrobial agents.4,5 Nonetheless, TiO2 NPs are identified by the Organisation for Economic Co-operation and Development (OECD) as priority contaminants for ecotoxicity testing.6 Furthermore, considering the limitations of conventional pesticides, there is an urgent need to carefully review pesticides in the form of NPs to ensure their efficacy and safety.
Currently, TiO2 NPs can be synthesized by hydrothermal, microwave irradiation, and sol–gel methods,7 but these methods require hazardous chemicals and extreme reaction conditions, which are time-consuming, energy-wasting, and environmentally unfriendly.8 Therefore, plant- and microbial-mediated synthesis of NPs is of increasing interest to researchers. Synthesis using plant extracts is generally green, safe, and cost-effective, and by-products are less toxic and easy to handle, due to the antioxidants and secondary metabolites contained in the extracts that act as bio-capping and reducing agents to stabilize the formation of NP crystals.9 These advantages provide a strong security guarantee for the agricultural application of NPs. It has been shown that green synthesized NPs improved plant growth by enhancing antimicrobial properties,10 protecting defense systems,11 promoting nutrient uptake,12 and mitigating heavy metal stress13 compared to chemically synthesized NPs. This showed that the green synthesis method is a substitute process to improve the biocompatibility of NPs and alleviate phytotoxicity, but previous studies have not systematically elucidated why the products of this process have lower phytotoxicity from the source of energy synthesis. Moreover, most data on the interaction of TiO2 NPs with plants were based on c-TiO2 NPs, while key evidence for the practical application of g-TiO2 NPs in agriculture is lacking.
It is generally believed that NPs can accumulate in plant tissues through roots or leaves, but no consistent results have been obtained regarding the effects of NPs on plants. TiO2 NPs not only improve crop growth14 and enhance stress tolerance,5 but also cause abnormalities in metabolism,15 gene expression,16 and mineral nutrient uptake.17 Typically, mitochondria and chloroplasts are the main organelles attacked by nanopesticides, where chloroplasts are closely related to plant growth and very sensitive to environmental pollutants as the energy synthesis factory of plants. Middepogu et al.18 found that 20 mg L−1 TiO2 NPs reduced light absorption of Chlorella pyrenoidosa through the shading effect and entered the chloroplast stroma causing damage to thylakoids. Hong et al.19 demonstrated that photosynthesis-related genes in Brassica chinensis L. were sensitive to 350 mg kg−1 cerium oxide (CeO2) NPs, ultimately manifesting in reduced carbon sequestration efficiency. Lu et al.20 sprayed 20 mg mL−1 iron oxide (Fe2O3) NPs inducing significant production of hydroxyl radicals (·OH) that decomposed chlorophyll in Triticum aestivum. Overall, chemically synthesized NPs may disrupt photosynthesis, so can NPs synthesized with bioactive components regulate the operation of energy factories to reduce harm to non-target crops?
In this study, the widely consumed and cultivated Lactuca sativa L. was identified as a model crop, and TiO2 NPs synthesized from plant extracts and traditional chemicals were selected to (1) explore the effects of TiO2 NPs on lettuce growth (physiological and biochemical), (2) investigate changes in photosynthetic parameters and metabolites in the light reaction and Calvin cycle, (3) elucidate the influence mechanism of TiO2 NPs on photosynthesis in terms of energy required for photoreaction, chloroplast structure, cell management mechanism, and NP properties, and (4) compare the regulatory differences of TiO2 NPs synthesized by distinct methods on plant growth and photosynthesis. This study provides valuable information for the efficient and safe application of TiO2 NPs in agriculture, which will contribute to the sustainable development of nanopesticides.
2. Materials and methods
2.1 Preparation and characterization of NPs
Anatase c-TiO2 NPs (5–10 nm, 99.8%) were purchased from Ronghua Sci. & Tech. Co. (Nanjing, China). The g-TiO2 NPs were prepared with reference to Goutam et al.21 The detailed procedure is described in Text S1.† Energy dispersive spectroscopy (EDS, Octane Plus, USA) and X-ray diffraction (XRD, Ultima IV, Japan) indicated the successful synthesis of anatase g-TiO2 NPs (Fig. S1 and S2†). Average sizes of c-TiO2 NPs (9.3 nm) and g-TiO2 NPs (20.1 nm) were detected by scanning electron microscopy (SEM, ZEISS Gemini 300, Germany) (Fig. S3†).
2.2 Exposure and plant growth
Due to hydroponics being clean, fertilizer-saving, and high-yield, Lactuca sativa L. has been successfully grown commercially in hydroponic systems, the more easily controlled hydroponics was chosen as the culture method to simulate actual exposure scenarios. Lettuce seeds were provided by Hezhiyuan Seed Corporation (Shandong, China). The seeds were disinfected with 1% NaClO and grew for 3 weeks in a tray containing ⅛-strength Hoagland nutrient solution, and then the seedlings were transferred to triangle bottles containing ¼-strength Hoagland nutrient solution for 1 week. 10, 100, 250, and 500 mg L−1 NPs were uniformly sprayed on lettuce leaves with small sprayers from 9 a.m. to 10 a.m. for 14 consecutive days from the 5th week (2.5 ml per day per plant), and the TiO2 NP suspension was ultrasonicated for 30 min before exposure. Control checks (CK) were performed with water instead of NPs. At the same time, the bottle was covered to prevent the hydroponic substrate from being contaminated. Each group was supplemented with an equal amount of nutrient solution every 2 days. There were three replicates per treatment and all incubations were performed in a light incubator with a diurnal cycle of 16/8 h (23/18 °C).
2.3 Physiological and photosynthetic index testing
2.3.1 Growth parameters and nutrient content.
Fresh weights of lettuce tissues treated for 2 weeks were determined after ultrasonic cleaning with EDTA-Na2, and dry weights were obtained after drying. The dried leaves were digested with concentrated H2SO4 and H2O2 (2
:
1) at 200 °C until the solution was clear and then it was filtered to obtain the digestion solution. Elemental contents were detected by atomic absorption spectroscopy (AAS, PerkinElmer Optima 8000, USA).
2.3.2 Measurement of oxidative stress indicators.
Fresh leaves were placed in a pre-chilled mortar and homogenized with 0.05 M 7.8 pH phosphate buffer solution (PBS) at a ratio of 1
:
10 (w
:
v) in an ice-cold environment, and the mixture was centrifuged to obtain a crude enzyme extract. The activities of catalase (CAT) and ascorbate peroxidase (APX) were determined as explained by Kohatsu et al.11 The superoxide dismutase (SOD) activity was measured following the assay published by Ji et al.22 The reduced glutathione (GSH) content was analyzed according to Huang et al.23
2.3.3 Photosynthetic parameter analysis.
The net CO2 assimilation rate (A), stomatal conductance (Gs), intercellular CO2 concentration (Ci), and transpiration rate (E) were determined with a portable photosynthesis measurement system (TARGAS-1, Hansatech, UK). A pulse-modulated fluorometer (FMS 2, Hansatech, UK) was used to calculate the maximum quantum efficiency (Fv/Fm), photochemical efficiency of photosystem II (ΦPSII), photochemical quenching (qP), non-photochemical quenching (NPQ) and photosynthetic electron transport rate (ETR). The chlorophyll content was measured using the ethanol method.
2.3.4 Assessment of changes in biomolecules.
Fourier Transform Infrared (FTIR, Spotlight 200i, PerkinElmer) spectroscopy was adopted to obtain changes in leaf biomolecules. Band assignments based on previous studies are given in Table S1.† Two-dimensional correlation spectroscopy (2D-COS) was utilized to improve the resolution of the spectra, and the amide I region was further analyzed using the second derivative spectra. The average results from three replications were analyzed and plotted. See Text S2† for a detailed description.
2.4 Determination of energy and metabolites in photosynthesis
The contents of nicotinamide adenine dinucleotide phosphate (NADPH) and protein in leaves were extracted and determined using reagent kits (Nanjing Jiancheng Biotechnology Institute, China). The activities of ribulose-1,5-bisphosphate carboxylase/oxygenase (Rubisco) and adenosine triphosphate synthase (ATPase) in leaves were measured using an enzyme-linked immunosorbent assay (ELISA) kit (Beijing Libo Biotechnology Co., Ltd., China). The soluble sugar content in leaves was assayed with anthrone colorimetry.
2.5 Hill reaction and optical property monitoring
Chloroplast suspensions were extracted as defined by Pan et al.24 and the chlorophyll concentration was 360 mg L−1. Chloroplast suspensions were mixed with 10, 100, 250, and 500 mg L−1 TiO2 NPs (1
:
1), respectively, and incubated in the dark for 2 h to obtain TiO2 and chloroplast complexes (TiO2/Chl). The Hill reaction was performed to evaluate the effects of TiO2 NPs on photosynthetic activity. The reduction of 2,6-dichlorophenol indophenol (DCPIP) and potassium ferricyanide was determined with reference to Li et al.25 with minor modifications. The difference in photoelectron generation rates of c-TiO2 NPs and g-TiO2 NPs was identified by methylene blue (MB) reduction. UV-vis spectra of TiO2/Chl were determined on a spectrophotometer (Spotlight 200i, Perkin Elmer). Refer to Text S3† for the detailed process.
2.6 Observation of subcellular structure
After 14 days of exposure, leaves from the control, 10 mg L−1 g-TiO2 NP, 500 mg L−1 g-TiO2 NP, and c-TiO2 NP treatments were selected for glutaraldehyde fixation. After rinsing with 0.1 M PBS, the leaves were immersed in 1% osmium acid solution, dehydrated in gradient ethanol, and transferred to acetone solution. Subsequently, the tissues were put in a mixture of embedding agent and acetone and then left overnight in the pure embedding agent. Finally, sections made with an ultramicrotome (Leica EM UC7, Germany) were stained with lead citrate and uranyl acetate, and chloroplast structures were examined using a transmission electron microscope (TEM, Hitachi H-7650, Japan).
2.7 Visualization of autophagosome
To visualize the structure of intracellular autophagy, monodansylcadaverine (MDC) staining was performed following the method by Wang et al.26 with minor modifications (Text S4†). Briefly, protease inhibitor-treated leaves were immediately vacuum stained with 100 μM MDC (Sigma-Aldrich) in the dark for 30 min at a constant temperature, then rinsed with pH 7.4 PBS and observed under confocal laser scanning microscopy (CLSM, LSM 800, Zeiss, Germany) for fluorescence from MDC and chloroplasts.
2.8 Statistical analysis
All data in this work were displayed as mean ± standard deviation (SD). One-way ANOVA was performed using IBM SPSS Statistics 26 and combined with Duncan's multiple comparison test, and the significance level of all results was defined as p < 0.05.
3. Results and discussion
3.1 Disturbance of antioxidant defense system and growth
The effects of TiO2 NPs on lettuce growth were assessed by measuring changes in biomass and inorganic nutrients. As seen in Fig. 1, both NPs negatively affected growth at concentrations above 100 mg L−1, and c-TiO2 NPs exhibited greater phytotoxicity. It is worth noting that 10 mg L−1 g-TiO2 NPs increased the fresh weight of roots and leaves by 1.35 and 1.17 times over the control (Fig. 2A). Furthermore, TiO2 NPs interfered with macro- and micro-nutrient uptake. Specifically, most plants contained fewer elements after exposure, but 10 mg L−1 c-TiO2 NPs (Mn, Zn) and g-TiO2 NPs (Ca, Mg, and Mn) promoted elemental absorption (Fig. 2C). These elements are closely related to plant development, and an imbalanced intake of any of them can lead to physiological disorders. Xie et al.27 found that CeO2 NPs may affect mineral accumulation in Phaseolus vulgaris by interfering with ion transport channels, thus directly limiting plant growth. Consequently, the reduction of nutrients caused by NPs is one of the causes of lettuce biomass decrease.
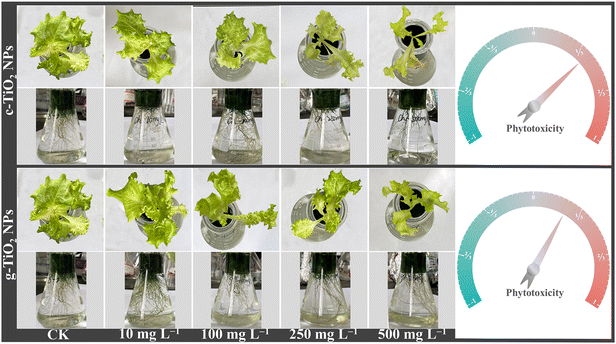 |
| Fig. 1 Morphology of lettuce leaves and roots in different treatment groups and indications for phytotoxic intensity of c-TiO2 NPs and g-TiO2 NPs. Numbers denote the proportion of up-regulation (blue) or down-regulation (red) in leaf fresh weight at 500 mg L−1 compared to the control, with darker colors meaning greater intensity. | |
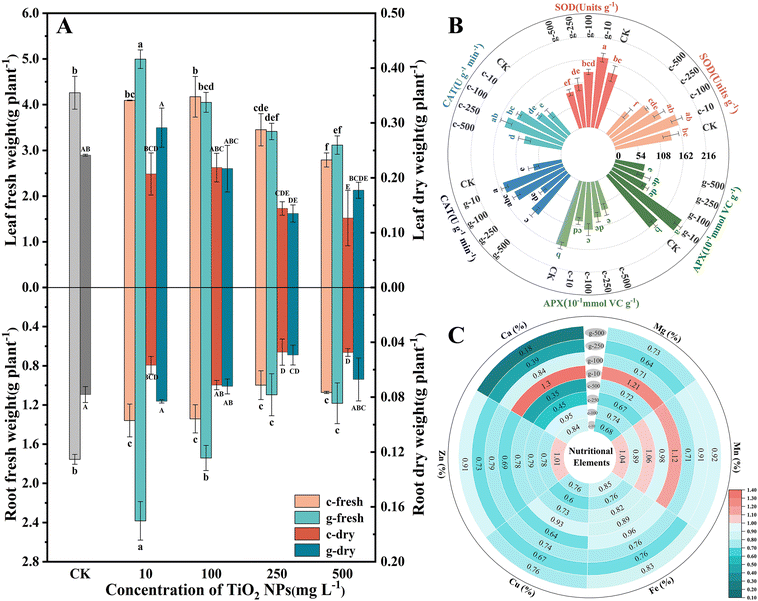 |
| Fig. 2 Fresh weight and dry weight of leaves and roots (A), antioxidant enzyme activities in leaves (B), and changes of inorganic elements in leaves (C), values in cells represent fold change in content compared to the control, red indicates increase and blue reflects decrease. Different letters represent significant differences (p < 0.05). c-: c-TiO2 NPs and g-: g-TiO2 NPs. | |
As a by-product of aerobic metabolism, low levels of reactive oxygen species (ROS) are involved in regulating plant physiological activities, but external stresses can break the balance between ROS production and clearance, at which point plants produce enzymatic and non-enzymatic antioxidants to maintain cellular stability. As shown in Fig. 2B, TiO2 NPs disrupted the redox metabolic status of lettuce. Compared with the control, 10 mg L−1 g-TiO2 NPs significantly increased SOD, CAT, and APX activity. The SOD activity showed a U-shaped curve trend and was strongly inhibited at 500 mg L−1, but was unrelated to the synthesis method. SOD is often considered the first line of defense against oxidative stress by disproportionating superoxide radicals (·O2−) to H2O2 and O2,28 after which APX is involved in the removal of H2O2, and the remaining H2O2 can be reduced to H2O by CAT.29 Thus, the diminished APX activity in all treatment groups except 10 mg L−1 g-TiO2 NPs indicated that APX could not completely decompose H2O2, which further stimulated CAT (Fig. 2B). The increased antioxidant enzyme activity was a compensatory mechanism for ROS accumulation in lettuce, but excess ROS inhibited activity and may even oxidatively damage proteins, lipids, and DNA.27 Moreover, except for enzymatic reactions, which are the preferred pathway for ROS detoxification, non-enzymatic antioxidants play an invaluable role in resisting oxidative stress. In Fig. S4,† the GSH content decreased dramatically only at 500 mg L−1 c-TiO2 NPs, which was caused by the oxidation of GSH to oxidized glutathione (GSSG) by uncleared ROS. The above findings revealed that c-TiO2 NPs and g-TiO2 NPs activated the antioxidant system, while 10 mg L−1 g-TiO2 NPs had stronger oxidative protection.
3.2 Response of photosynthetic parameters
Changes in growth are directly related to photosynthesis. As observed in Fig. 3A, A was significantly enhanced after spraying of 100 mg L−1 c-TiO2 NPs (∼12%) and 10 mg L−1 g-TiO2 NPs (∼48%), so appropriate doses of TiO2 NPs promoted photosynthesis and lettuce could synthesize more organic matter. c-TiO2 NPs (100, 500 mg L−1) and g-TiO2 NPs (10, 500 mg L−1) increased Gs and E. Theoretically, Gs was negatively correlated with Ci, but 500 mg L−1 NP groups had higher Ci, indicating that CO2 fixation was limited. Chlorophyll is an essential pigment for photosynthesis, which converts light energy into chemical energy. In Fig. S5,† the content of chlorophyll presented dose-dependent decreases with increasing exposure time and g-TiO2 NPs were less toxic. In contrast, 10 mg L−1 g-TiO2 NPs increased photosynthetic pigments. This difference can be attributed to the effect of TiO2 NPs on the uptake of minerals (Mg, Mn, etc.) (Fig. 2C), as Mg is important for chlorophyll synthesis, and Fe, Mn, and Zn are auxiliary elements during photosynthetic pigment formation.30,31 On the other hand, ·OH, which cannot be scavenged by the plant antioxidant system, is a very strong non-selective oxidant that can decompose chlorophyll. These results showed that TiO2 NPs inhibited light absorption and conversion by impeding chlorophyll biosynthesis or promoting chlorophyll degradation, while 10 mg L−1 g-TiO2 NPs resulted in more Mg accumulation and minimized ROS impairment, thereby mitigating phytotoxicity.
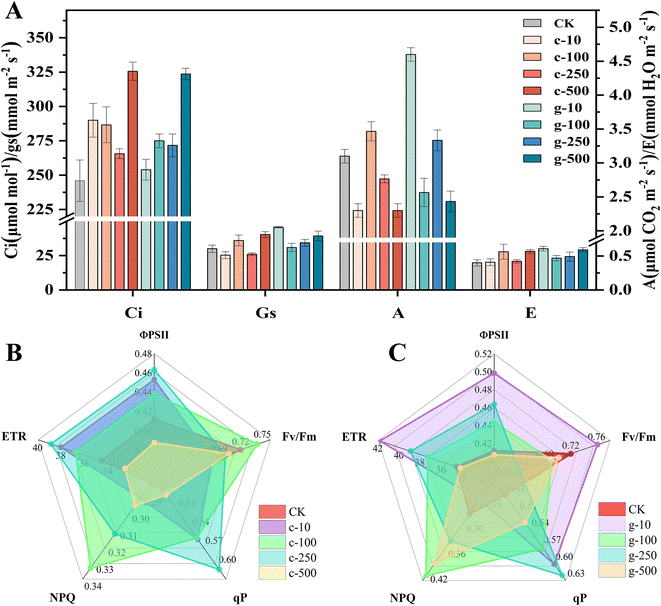 |
| Fig. 3 Gas exchange parameters of lettuce (A) and chlorophyll fluorescence parameters (B and C). c-: c-TiO2 NPs, g-: g-TiO2 NPs. | |
Photosynthetic fluorescence parameters were measured to gain more information about the influence of TiO2 NPs on the photosystem. As shown in Fig. 3B and C, g-TiO2 NPs had higher ΦPSII, ETR, and qP than c-TiO2 NPs and exhibited the greatest positive effect on ΦPSII or ETR at 10 and 250 mg L−1, respectively, but both were lower than the control at the highest concentration. It was found that all qP parameters were above the untreated group, indicating that both NPs enhanced the photochemical reaction of lettuce. The impeded ETR may be owing to the lack of Fe and Cu in the leaves (Fig. 2C) because the electron transport from QA to QB requires Fe catalysis,32 and the electron transfer from the cytochrome b6f complex to photosystem I (PSI) is associated with Cu.33 More information on the correlation between TiO2 NPs and electron transfer will be further explored below. In addition, insufficient levels of Mn, an important metal cofactor for electron transfer in PSII, were also associated with decreased ΦPSII and ETR. Fv/Fm reflects the photosynthetic efficiency of PSII under dark conditions, i.e., the number of absorbed photons that can drive electron transport.34 In all experimental groups, the Fv/Fm values of lettuce exposed only to 100 mg L−1 c-TiO2 NPs and 10 mg L−1 g-TiO2 NPs were higher than the control (Fig. 3B and C). The inhibition of Fv/Fm confirmed that the PSII reaction center was disrupted. NPQ prevents photodamage by dissipating excess excitation energy and is an embodiment of plant photoprotective capacity.35 It can be observed that NPQ under 10 mg L−1 g-TiO2 NP treatment was only 0.88 of the control, suggesting that lettuce could use light energy more efficiently and reduce heat dissipation. Other groups of g-TiO2 applications had higher NPQ than c-TiO2, which might be due to enhanced light capture by g-TiO2 NPs. In general, g-TiO2 NPs showed more potential in improving photoreaction efficiency, but the problem of photodamage caused by excess light energy should be noted.
3.3 Alterations in Calvin cycle metabolites
The perturbed light reaction would affect CO2 fixation. In this work, FTIR spectroscopy was used to identify changes in lipids (2500–3500 cm−1), proteins (1200–1800 cm−1), and carbohydrates (800–1200 cm−1) of leaves. Signals in the 3585–3100 cm−1 band identified in the control were weakened in the treated samples (Fig. 4A). Notably, new peaks appeared especially under g-TiO2 NP treatment at 1551 cm−1 and 1460 cm−1 in the amide II region. These findings implied that g-TiO2 NPs have the potential to increase the amide species of protein. The higher dose of NPs also attenuated the peak intensity corresponding to amide III (1240 cm−1). Besides, NPs adversely impacted carbohydrates, as exemplified by the weakened peak strength at 1052 cm−1 and 1161 cm−1 representing cellulose, and the peak at 824 cm−1 associated with sugar skeleton vibration almost disappeared. Similar to our findings, Bakshi et al.36 found that TiO2 NPs elicited the reprogramming of macromolecular metabolism in Solanum lycopersicum.
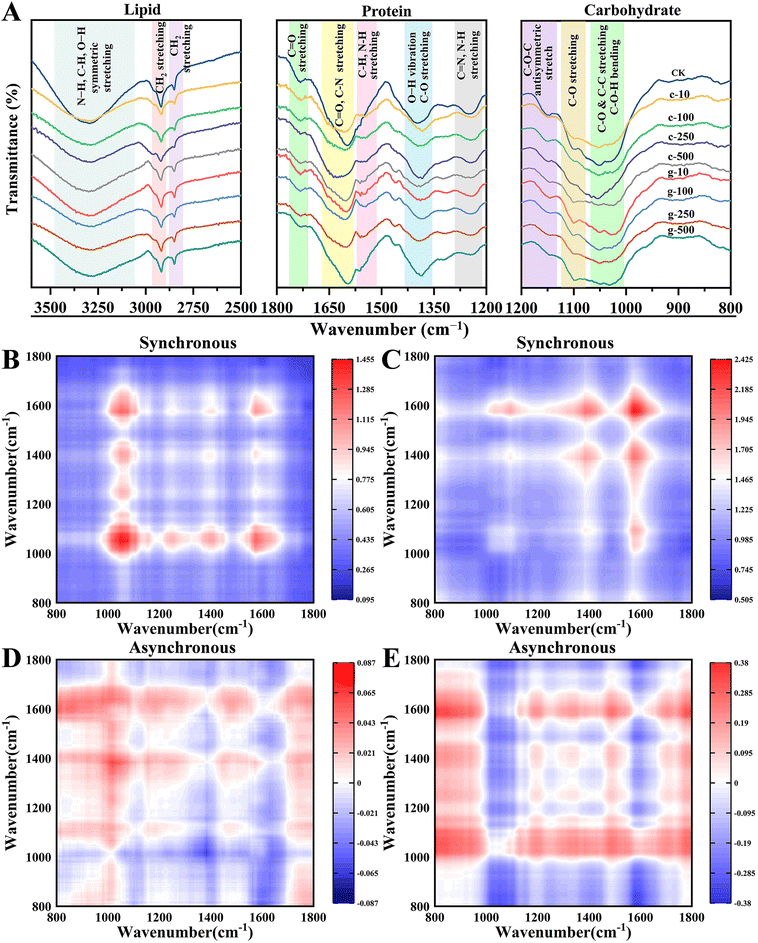 |
| Fig. 4 FTIR spectrum of lettuce leaves (A) and synchronous (B and C) and asynchronous (D and E) 2D-FTIR-COS maps generated from the 1800–800 cm−1 region under c-TiO2 NP (B and D) and g-TiO2 NP (C and D) treatments. c-: c-TiO2 NPs, g-: g-TiO2 NPs. | |
It is well-known that the 1600–1700 cm−1 region is characteristic of amide I,37 which was observed to be deformed and attenuated. It reflects the protein secondary structure, including β-sheet (1610–1640 cm−1), random coil (1640–1650 cm−1), α-helix (1650–1658 cm−1), and β-turn (1660–1700 cm−1).38 This work enhanced the identification of raw characteristic peaks by FTIR curve fitting based on the second derivative spectra to analyze protein structures (Fig. S6†). It can be seen from Table S2† that the protein structure in leaves changed from α-helix to β-sheet at 100 and 250 mg L−1, and the c-TiO2 NP treatment showed a stronger response. Normally, the transition from α-helix to β-sheet is considered a form to resist oxidative stress in adversity, such as antibiotic,39 perfluorooctanoic acid,40 perfluorooctane sulfonic acid stress,41etc. The α-helix is more compact to enhance protein tolerance of external disturbances, while the loose β-sheet provides more redox active sites, which can lead to oxidative damage of protein. From this, the increase in β-sheet conformation induced by TiO2 NPs may cause more serious physiological or metabolic abnormalities.
To further investigate the discrepancies between the two NPs on biomolecules, concentration-dependent 2D-FTIR-COS analysis was adopted (Fig. 4B–E). The diagonal auto-peak in the 2D-FTIR-COS synchronous map means sensitivity to perturbations, while the cross-peak represents changes in the corresponding peaks that have the same mechanism and origin.42 In this study, most auto-peaks appeared in the 800–1800 cm−1 region (Fig. S7†). Differently, the most obvious auto-peaks appeared in dissimilar regions in the c-TiO2 NPs (800–1200 cm−1) and g-TiO2 NPs (1200–1800 cm−1) spectra, signifying that carbohydrates and proteins were more susceptible to c-TiO2 NP and g-TiO2 NP interference, respectively. The soluble protein and starch content in leaves exhibited a similar trend (Fig. S8†). This contradicted the results of previous protein structural analysis, which may be caused by stronger changes of amide II and amide III than those of amide I. All cross-peaks with the positive signal implied that changes in biochemical components corresponding to IR signals in leaves were synchronized.
In addition, the order of change in functional groups in response to external disturbances can be further determined by comparing cross-peak signals in synchronous and asynchronous maps.42 The information for each cross-peak symbol is collated in Table S3† according to Noda's rule.43 The functional group responded to c-TiO2 NPs in the order 1052 cm−1 > 3307 cm−1 > 1243 cm−1 > 1385 cm−1 > 1596 cm−1. Therefore, c-TiO2 NPs first elicited disruption of carbohydrates such as cellulose, after which amides and suberin were destroyed. Likewise, leaves exposed to g-TiO2 NPs exhibited a reaction sequence of 654 cm−1 > 1385 cm−1 > 1596 cm−1 > 3307 cm−1 > 1052 cm−1. Unlike c-TiO2 NPs, g-TiO2 NPs elicited a more rapid change in proteins. This coincided with the sensitivity of macromolecules to NPs in synchronous maps. In conclusion, foliar application of TiO2 NPs altered Calvin cycle metabolites in lettuce and different macromolecules presented divergent sensitivities to the two NPs. The strong interference of c-TiO2 NPs with carbohydrates can directly cause low yields. Ahmed et al.44 pointed out that disturbance of protein by contaminants could affect the lipid and carbohydrate content and category, so a high response of protein to g-TiO2 NPs would pose a further threat to growth.
3.4 Mechanism of photosynthetic response induced by NPs
3.4.1 Modulation of light absorption and conversion.
To explore the mechanism of TiO2 NPs affecting the lettuce photoreaction and the Calvin cycle, important enzyme activities and the product content during photosynthesis were measured. It is widely known that electrons in photosystems can be transferred to NADP+ to reduce it to NADPH. The NADPH yield can reflect the rate of photosynthetic electron transport.45 As shown in Table 1, NADPH was more influenced by g-TiO2 NPs, which were all more than twice as much as the control, suggesting that g-TiO2 NPs increased the number of electrons in the PSI. Meanwhile c-TiO2 NPs increased NADPH by 42% only at 250 mg L−1. Thereby, the discrepancy between the two NPs on photosynthetic electron transport should not be ignored. ATPases play a critical role in the phosphorylation process of photosynthesis. The NPs inhibited ATPase activity except for 10 mg L−1 g-TiO2 NPs (up 14%), which directly down-regulated ATP production in the photochemical phase. Moreover, insufficient NADPH and ATP will prevent the process of carboxylation, reduction, and regeneration in the Calvin cycle,33 because 3-phosphoglycerate (PGA) and glyceraldehyde-3-phosphate (GAP) as photosynthetic intermediates cannot be converted without catalysis of ATP and NADPH. Carboxylation is the first step for CO2 to enter the plant, and this process is regulated by Rubisco, which catalyzes CO2 into energy-rich metabolites.46 The Rubisco activity decreased after the application of NPs, where it was significantly higher under g-TiO2 NP treatment than c-TiO2 NP treatment at 10 and 100 mg L−1, but the opposite was true at 500 mg L−1, which may be related to the greater sensitivity of protein to g-TiO2 NPs (Fig. 4C). Moreover, the ROS burst also reduced the Rubisco activity.47 The CO2 fixation efficiency of Rubisco is only 1–10 s−1,48 making it a rate-limiting enzyme for photosynthesis, so the decrease in its activity inhibited carbohydrate synthesis and thus affected the respiratory process.47 Middepogu et al.18 confirmed that restriction of Chlorella pyrenoidosa development by TiO2 NPs stemmed from inhibition of ATPase activity. Therefore, even though TiO2 NPs reduced more NADP+ by facilitating photosynthetic electron transfer, their inhibition of ATPase and Rubisco resulted in the underutilization of NADPH, thereby limiting the conversion of PGA and GAP to downstream metabolites such as amino acids (Fig. S8A†), sugars (Fig. S8B†), and fatty acids.
Table 1 Influences of TiO2 NPs on photosynthesis energy and the ability of TiO2 NPs or TiO2/Chl to generate electrons
Treatment |
Dose (mg L−1) |
NADPH content (nmol g−1 FW) |
ATPase activity (U mg−1 prot) |
Rubisco activity (U g−1 FW) |
Reduction (%) |
DCPIP |
Ferricyanide |
MB |
Note: different letters represent significant differences (p < 0.05). |
Control |
0 |
6.70 ± 0.10e |
3.46 ± 0.10b |
0.29 ± 0.004a |
19.21 ± 0.15e |
39.47 ± 1.49e |
13.75 |
c-TiO2 NPs |
10 |
5.69 ± 0.20e |
3.13 ± 0.09c |
0.27 ± 0.004c |
23.28 ± 0.78de |
43.81 ± 1.51de |
26.41 |
100 |
6.12 ± 0.10e |
2.08 ± 0.08ef |
0.24 ± 0.005ef |
47.85 ± 0.73c |
58.41 ± 0.93c |
35.66 |
250 |
9.50 ± 0.20d |
1.89 ± 0.12f |
0.25 ± 0.003e |
54.46 ± 1.26b |
71.65 ± 1.91b |
39.74 |
500 |
5.47 ± 0.61e |
2.89 ± 0.11cd |
0.24 ± 0.003f |
65.69 ± 1.56a |
75.47 ± 1.36ab |
70.04 |
g-TiO2 NPs |
10 |
20.45 ± 0.41a |
3.94 ± 0.15a |
0.28 ± 0.002b |
28.76 ± 0.27d |
47.92 ± 1.75d |
30.07 |
100 |
14.54 ± 0.20c |
2.64 ± 0.18d |
0.26 ± 0.004d |
42.22 ± 1.28c |
56.77 ± 1.92c |
34.39 |
250 |
17.14 ± 1.02b |
2.27 ± 0.10ef |
0.24 ± 0.002f |
58.10 ± 0.46b |
75.68 ± 1.78ab |
50.12 |
500 |
14.26 ± 0.81c |
2.93 ± 0.11c |
0.23 ± 0.003g |
67.43 ± 3.85a |
78.73 ± 1.77a |
75.27 |
It has been discussed in section 3.2 that the decrease in ETR was due to the deficiency of crucial elements in the photosynthetic electron transfer process; meanwhile, could the increased ETR under NP treatment (≤250 mg L−1) be related to the photoelectric properties of TiO2 NPs? To address this issue, MB degradation by TiO2 under light was measured, and the interaction between NPs and plants at the organelle level was explored using chloroplasts isolated from lettuce. As seen in Table 1, MB degradation increased with dose after irradiation with xenon lamps for 3 h, which was accompanied by photogenerated electrons from NPs, while g-TiO2 NPs showed higher effects in most groups. Subsequently, the Hill reaction was used to verify that this light-absorbing capacity of NPs could be utilized by chloroplasts to increase photosynthetic activity. DCPIP was chosen as the electron acceptor to intercept electrons in the photosystem.49 The reduction of DCPIP was faster in treated groups than in chloroplasts alone, but this was independent of the synthesis method. Then, the ferricyanide reduction experiment by TiO2/Chl was adopted to further determine whether enhanced Hill activity was related to NPs, whose reaction trend was similar to DCPIP, and there were no significant differences between the two NPs. The above results indicated that TiO2 NP application supplemented the electrons in photosystems, thus improving the photosynthetic efficiency. In Fig. S9,† chloroplasts showed two absorption bands at 400–500 nm and 650–700 nm and TiO2/Chl complexes had the same absorption pattern as bare chloroplasts, but with greater absorption intensity, in which c-TiO2/Chl exhibited more pronounced absorption. Combined with Table S4,† g-TiO2 NPs had stronger adsorption capacity because they were more dispersed in the aqueous solution, which explained why g-TiO2 NPs with weak UV absorption degraded MB faster instead.
Therefore, the greater advantage of g-TiO2 NPs over c-TiO2 NPs in enhancing NADPH and ETR is mainly due to improved electron transport processes, such as elemental uptake, chloroplast activity, and cellular homeostasis, rather than the effect of the optoelectronic properties of NPs. Notably, the enhanced absorption of the UV-A region stressed plants after exposure to NPs (Fig. S9†),50 while excess electrons in the light reaction triggered a ROS burst that inhibited photosynthesis,25 which explained the decrease in antioxidant enzyme activity at high concentrations as shown in Fig. 2B. Therefore, even though both TiO2 NPs increased electrons in the photosynthetic chain, their inhibition of critical enzyme activities and induction of ROS counteracted some positive effects.
3.4.2 Disruption of the cellular ultrastructure.
In addition to the limitation of photosynthetic reactions, metabolic disorders stemming from the disrupted chloroplast structure may be another critical reason for poor photosynthetic efficiency. Chloroplasts showed diverse structural features as observed from the TEM images (Fig. 5). Chloroplasts in the control group were clear in the cytoplasm and were ellipse-aligned along the cell wall, indicating vacuole integrity and a normal chloroplast shape. Also, there were more chloroplasts and starch grains in the control, and the thylakoid layer was clearly distributed and tightly clustered (Fig. 5A–C). The difference was that thylakoids tended to loosen and dissociate after 10 mg L−1 g-TiO2 NP treatment (Fig. 5D–F). Under 500 mg L−1 treatment, the number of chloroplasts and starch grains decreased, and osmiophilic granules increased, where c-TiO2 NPs reduced thylakoid stacking (Fig. 5G–J).
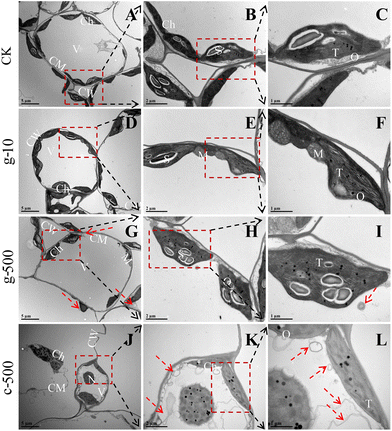 |
| Fig. 5 Ultrastructure of lettuce chloroplasts under control (A–C), g-10 (D–F), g-500 (G–I), and c-500 (J–L) treatments. CW: cell wall, Ch: chloroplast, S: starch granule, CM: cell membrane, T: thylakoid, O: osmiophilic granule, N: nucleus, V: vacuole, c-: c-TiO2 NPs, g-: g-TiO2 NPs. Arrows indicate autophagosomal-like structures. | |
Normally, thylakoids are stacked into grana in the chloroplast,18 so the dissolution of their lamellar structures was an important reason for blocked light absorption. Besides, the increased osmiophilic granules suggested that TiO2 NPs elicited oxidative damage in plants, as they are usually a symbol of oxidative stress,51 which was strongly supported by the significant inhibition of enzymatic and non-enzymatic antioxidants at 500 mg L−1. The decrease in starch granules was the result of their degradation or reduced efficiency of carbon assimilation. It was noted that starch degradants are osmotic and can lead to inflation of organelles,17 which explained the deformation and over-swelling of chloroplasts observed in Fig. 5D, G and J. Starch drives most carbon fluxes in plants and occupies an essential position in carbon allocation, and its deficiency can directly restrict crop growth (Fig. S8B†). Previous studies have confirmed that NPs can be internalized by leaves through the cuticle (0.6–4.8 nm)52 and stomata (about 20–40 μm long and 5–10 μm wide).53 Therefore, smaller c-TiO2 NPs were more likely to enter cells through the stomata than g-TiO2 NPs in this work, thus posing a greater threat to the integrity of chloroplasts and thylakoid membranes. In summary, TiO2 NPs had negative effects on thylakoids even at low concentrations, and higher doses induced chloroplast degradation, but this phenomenon was more pronounced under c-TiO2 NP exposure.
3.4.3 Occurrence of autophagic response.
It has been confirmed that autophagy is a mechanism for modulating cellular constituents. When faced with abiotic stresses including heat, high salinity, and drought, plants remove unwanted intracellular components by triggering an autophagic signaling cascade in vivo. Alternatively, autophagy can also regulate critical metabolic processes by decomposing certain organelles or cells during normal growth.54 During autophagy, the sequestering membrane called the phagophore or isolation membrane will engulf the cytoplasm and eventually form an autophagosome with a double-membrane structure.55 According to the autophagosomal-like structures in Fig. 5G and K, it was speculated that TiO2 NPs might trigger the intracellular autophagic response. To further verify the conjecture, MDC, which specifically stains autophagosomes, was selected to label acidic spaces. There were no obvious punctate fluorescence structures in leaves treated without NPs and 10 mg L−1 g-TiO2 NPs (Fig. 6A). However, the number of MDC-labeled autophagosomes increased appreciably at high concentrations, and numerous fluorescent spots were observed around chloroplasts, especially after c-TiO2 NP coercion (Fig. 6C and D). This was consistent with the quantitative analysis of MDC fluorescence, where the MDC fluorescence intensity under 500 mg L−1 g-TiO2 NP and c-TiO2 NP treatments was 3.2-fold and 8.6-fold stronger than the control, respectively (Fig. 6B). Higher than normal levels of autophagy showed that more organelles were destroyed by TiO2 NPs, which was a manifestation of nanotoxicity.
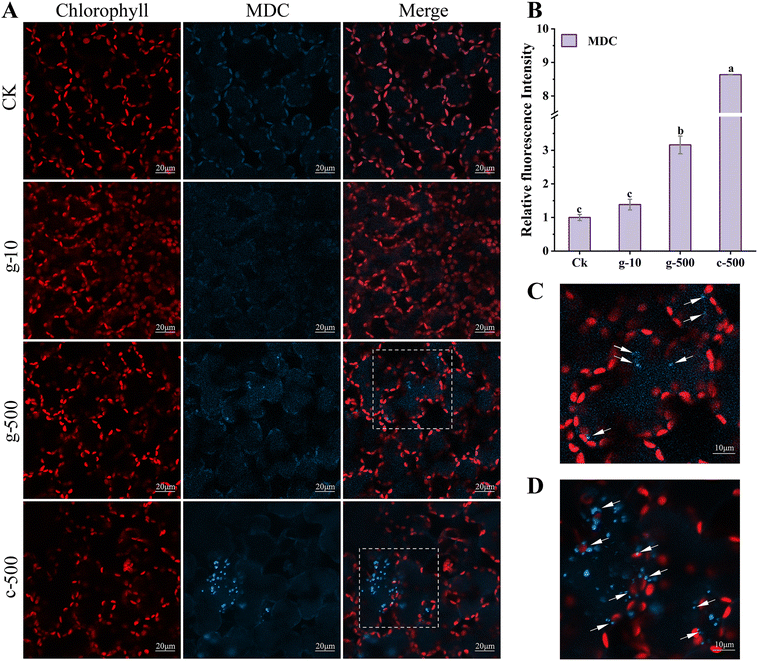 |
| Fig. 6 CLSM images of leaf tissue stained by MDC (A), the relative fluorescence intensity of MDC (B), and enlarged views of blue dashed boxes in g-500 (C) and c-500 (D) groups in A, respectively. MDC-labeled structures are blue and chloroplasts are red. Quantification of autophagic activity relative to the control by calculating the mean fluorescence intensity in 6 fields of view. Different letters represent significant differences (p < 0.05). c-: c-TiO2 NPs, g-: g-TiO2 NPs. | |
The prerequisites for autophagy are usually inextricably linked to a burst of ROS, which oxidizes cellular macromolecules and then leads to organelle damage and cytological dysfunction. Consequently, as a plant housekeeping mechanism, autophagy will clean up these components destroyed by excess ROS induced by TiO2 NPs. Colocalization of chloroplast and MDC fluorescence suggested that TiO2 NPs activated the autophagic degradation of chloroplasts. This was proved in Fig. S10,† where 500 mg L−1 TiO2 NPs reduced the number of chloroplasts and their fluorescence intensity, but inhibition was greater under c-TiO2 NP treatment. Summarizing the above results, the photosynthetic response model of lettuce to TiO2 NPs is illustrated in Scheme 1. Additionally, Izumi et al.55 reported that the breakdown of Rubisco was closely related to autophagy in rice. Since approximately half of the soluble proteins in leaves are derived from Rubisco, the decrease in Rubisco activity (Table 1) and soluble protein content (Fig. S8A†) was also associated with chloroplast autophagy. On the other hand, plants can also maintain survival under stress by limiting programmed cell death56 and recycling beneficial components57 through autophagy. Collectively, higher levels of TiO2 NPs triggered the autophagic protection mechanism in lettuce but reduced the activity and number of chloroplasts, which was detrimental to the utilization of light energy.
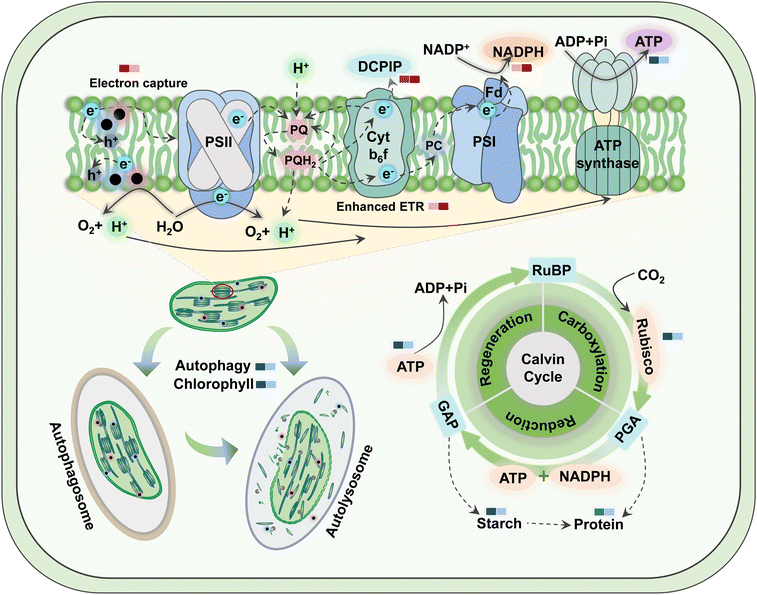 |
| Scheme 1 Schematic representation of TiO2 NPs modulating photosynthesis in lettuce. The left rectangle of the color bar represents c-TiO2 NPs, and the right means g-TiO2 NPs. Red indicates up-regulation, blue indicates down-regulation, and the shade of color reflects the intensity of regulation. Fd, ferredoxin; Cytb6f, cytochrome b6f complex; PQ, plastoquinone; PQH2, plastoquinol; PC, plastocyanin; RuBP, ribulose 1,5-bisphosphate; GAP, glyceraldehyde-3-phosphate; PGA, 3-phosphoglycerate. | |
4. Conclusion
This study evaluated the effects of lettuce photosynthesis by foliar spraying of TiO2 NPs synthesized with different methods and investigated the modulatory principles of TiO2 NPs on photosynthesis in terms of light energy conversion, chloroplast structure, cellular management mechanisms, and NP properties. Results revealed that c-TiO2 NPs and g-TiO2 NPs exert dose-dependent impacts on plant growth. They perturbed the accumulation of nutrients, including Mg, Fe, Cu, Zn, and Mn, thereby impeding chlorophyll synthesis and photosynthetic electron transport. NPs promoted chloroplast absorption of light at 300–800 nm wavelength, but this advantage caused a burst of oxidative radicals at high concentrations, which induced thylakoid lysis, chlorophyll degradation, and defense system destruction, and eventually evoked a decline in photosynthetic efficiency and resulted in disruption of carbon metabolism. Therein, these phenomena were more obvious when exposed to c-TiO2 NPs with superior photoelectric properties. Furthermore, direct biomass loss in leaves (2–35%) and roots (23–44%) resulted from a stronger response of carbohydrates to c-TiO2 NPs, while high susceptibility of proteins to g-TiO2 NPs posed a threat to carbohydrates and lipids during long-term exposure. Notably, 10 mg L−1 g-TiO2 NPs alleviated stress by regulating pigment synthesis, photophosphorylation, antioxidant enzymes, and chloroplast activities. Overall, g-TiO2 NPs show greater potential for agricultural development than c-TiO2 NPs and play a critical role in modulating plant photosynthesis when used appropriately, providing additional evidence for the efficient combination of nanotechnology and plant protection products. It should be noted that foliar exposure to NPs altered nutrient uptake, so further verification of root damage is necessary, and health risks of titanium levels in plants also need to be considered before practical application.
Author contributions
Yuzhu Weng: conceptualization, methodology, investigation, formal analysis, writing – original draft, writing – review & editing; Xue Bai: conceptualization, project administration, funding acquisition, supervision, writing-review & editing; Mengen Kang: investigation, writing – review & editing; Yue Huang: methodology, investigation; Yetong Ji: writing – review & editing; Haoke Wang: methodology; Zulin Hua: funding acquisition.
Conflicts of interest
There are no conflicts of interest to be declared.
Acknowledgements
The authors gratefully acknowledge the support provided by the National Natural Science Foundation of China (U2243601) and the Priority Academic Program Development of Jiangsu Higher Education Institutions of China.
References
- S. Cai, P. Zhang, Z. Guo, F. Jin, J. Wang, Z. Song, T. Nadezhda, I. Lynch and X. Dang, Multi-walled carbon nanotubes improve nitrogen use efficiency and nutritional quality in Brassica campestris, Environ. Sci.: Nano, 2022, 9, 1315–1329 RSC.
- V. M. Neves, G. M. Heidrich, E. S. Rodrigues, M. S. P. Enders, E. I. Muller, F. T. Nicoloso, H. W. P. Carvalho and V. L. Dressler, La2O3 Nanoparticles: Study of Uptake and Distribution in Pfaffia glomerata (Spreng.) Pedersen by LA-ICP-MS and μ-XRF, Environ. Sci. Technol., 2019, 53, 10827–10834 CrossRef CAS.
- M. Kah, R. S. Kookana, A. Gogos and T. D. Bucheli, A critical evaluation of nanopesticides and nanofertilizers against their conventional analogues, Nat. Nanotechnol., 2018, 13, 677–684 CrossRef CAS.
- D. Wang, N. B. Saleh, A. Byro, R. Zepp, E. Sahle-Demessie, T. P. Luxton, K. T. Ho, R. M. Burgess, M. Flury, J. C. White and C. Su, Nano-enabled pesticides for sustainable agriculture and global food security, Nat. Nanotechnol., 2022, 17, 347–360 CrossRef CAS PubMed.
- X. Pan, D. Nie, X. Guo, S. Xu, D. Zhang, F. Cao and X. Guan, Effective control of the tomato wilt pathogen using TiO2 nanoparticles as a green nanopesticide, Environ. Sci.: Nano, 2023, 10, 1441–1452 RSC.
- Q. Abbas, B. Yousaf, M. A. Mujtaba Munir, A. I. Cheema, I. Hussain and J. Rinklebe, Biochar-mediated transformation of titanium dioxide nanoparticles concerning TiO2NPs-biochar interactions, plant traits and tissue accumulation to cell translocation, Environ. Pollut., 2021, 270, 116077 CrossRef CAS PubMed.
- L. Xu, Z. Zhu and D. W. Sun, Bioinspired Nanomodification Strategies: Moving from Chemical-Based Agrosystems to Sustainable Agriculture, ACS Nano, 2021, 15, 12655–12686 CrossRef CAS PubMed.
- O. A. Qamar, F. Jamil, M. Hussain, S. Bae, A. Inayat, N. S. Shah, A. Waris, P. Akhter, E. E. Kwon and Y.-K. Park, Advances in synthesis of TiO2 nanoparticles and their application to biodiesel production: A review, Chem. Eng. J., 2023, 460, 141734 CrossRef CAS.
- Y. Li, Y. Fu and M. Zhu, Green synthesis of 3D tripyramid TiO2 architectures with assistance of aloe extracts for highly efficient photocatalytic degradation of antibiotic ciprofloxacin, Appl. Catal., B, 2020, 260, 118149 CrossRef CAS.
- H. Zhang, S. Chen, X. Jia, Y. Huang, R. Ji and L. Zhao, Comparation of the phytotoxicity between chemically and green synthesized silver nanoparticles, Sci. Total Environ., 2021, 752, 142264 CrossRef CAS.
- M. Y. Kohatsu, C. N. Lange, M. T. Pelegrino, J. C. Pieretti, G. Tortella, O. Rubilar, B. L. Batista, A. B. Seabra and T. A. d. Jesus, Foliar spraying of biogenic CuO nanoparticles protects the defence system and photosynthetic pigments of lettuce (Lactuca sativa), J. Cleaner Prod., 2021, 324, 129264 CrossRef CAS.
- J. Singh, S. Kumar, A. Alok, S. K. Upadhyay, M. Rawat, D. C. W. Tsang, N. Bolan and K.-H. Kim, The potential of green synthesized zinc oxide nanoparticles as nutrient source for plant growth, J. Cleaner Prod., 2019, 214, 1061–1070 CrossRef CAS.
- M. A. Irshad, M. Z. U. Rehman, M. Anwar-Ul-Haq, M. Rizwan, R. Nawaz, M. B. Shakoor, L. Wijaya, M. N. Alyemeni, P. Ahmad and S. Ali, Effect of green and chemically synthesized titanium dioxide nanoparticles on cadmium accumulation in wheat grains and potential dietary health risk: A field investigation, J. Hazard. Mater., 2021, 415, 125585 CrossRef CAS PubMed.
- E. Skiba, M. Pietrzak, S. Michlewska, J. Gruszka, J. Malejko, B. Godlewska-Żyłkiewicz and W. M. Wolf, Photosynthesis governed by nanoparticulate titanium dioxide. The Pisum sativum L. case study, Environ. Pollut., 2023, 340, 122735 CrossRef.
- B. Wu, L. Zhu and X. C. Le, Metabolomics analysis of TiO2 nanoparticles induced toxicological effects on rice (Oryza sativa L.), Environ. Pollut., 2017, 230, 302–310 CrossRef CAS PubMed.
- S. Schiavo, M. Oliviero, A. Philippe and S. Manzo, Nanoparticles based sunscreens provoke adverse effects on marine microalgae Dunaliella tertiolecta, Environ. Sci.: Nano, 2018, 5, 3011–3022 RSC.
- J. Hu, X. Wu, F. Wu, W. Chen, X. Zhang, J. C. White, J. Li, Y. Wan, J. Liu and X. Wang, TiO2 nanoparticle exposure on lettuce (Lactuca sativa L.): dose-dependent deterioration of nutritional quality, Environ. Sci.: Nano, 2020, 7, 501–513 RSC.
- A. Middepogu, J. Hou, X. Gao and D. Lin, Effect and mechanism of TiO2 nanoparticles on the photosynthesis of Chlorella pyrenoidosa, Ecotoxicol. Environ. Saf., 2018, 161, 497–506 CrossRef CAS PubMed.
- J. Hong, S. Jia, C. Wang, Y. Li, F. He and J. L. Gardea-Torresdey, Transcriptome reveals the exposure effects of CeO2 nanoparticles on pakchoi (Brassica chinensis L.) photosynthesis, J. Hazard. Mater., 2023, 444, 130427 CrossRef CAS PubMed.
- K. Lu, D. Shen, X. Liu, S. Dong, X. Jing, W. Wu, Y. Tong, S. Gao and L. Mao, Uptake of iron oxide nanoparticles inhibits the photosynthesis of the wheat after foliar exposure, Chemosphere, 2020, 259, 127445 CrossRef CAS.
- S. P. Goutam, G. Saxena, V. Singh, A. K. Yadav, R. N. Bharagava and K. B. Thapa, Green synthesis of TiO2 nanoparticles using leaf extract of Jatropha curcas L. for photocatalytic degradation of tannery wastewater, Chem. Eng. J., 2018, 336, 386–396 CrossRef CAS.
- H. Ji, J. Wang, A. Xue, F. Chen, H. Guo, Z. Xiao and Z. Wang, Chitosan–silica nanocomposites induced resistance in faba bean plants against aphids (Acyrthosiphon pisum), Environ. Sci.: Nano, 2023, 10, 1966–1977 RSC.
- H. Huang, M. Li, M. Rizwan, Z. Dai, Y. Yuan, M. M. Hossain, M. Cao, S. Xiong and S. Tu, Synergistic effect of silicon and selenium on the alleviation of cadmium toxicity in rice plants, J. Hazard. Mater., 2021, 401, 123393 CrossRef CAS.
- X. Pan, F. Fu, Z. Xie, W. Li, X. Yang, Y. Kang, S. Qu, Y. Zheng, Q. Li, H. Zhang, S. Song and B. Lei, Light-nutrition coupling effect of degradable fluorescent carbon dots on lettuce, Environ. Sci.: Nano, 2023, 10, 539–551 RSC.
- Y. Li, X. Pan, X. Xu, Y. Wu, J. Zhuang, X. Zhang, H. Zhang, B. Lei, C. Hu and Y. Liu, Carbon dots as light converter for plant photosynthesis: Augmenting light coverage and quantum yield effect, J. Hazard. Mater., 2021, 410, 124534 CrossRef CAS PubMed.
- Y. Wang, B. Yu, J. Zhao, J. Guo, Y. Li, S. Han, L. Huang, Y. Du, Y. Hong, D. Tang and Y. Liu, Autophagy contributes to leaf starch degradation, Plant Cell, 2013, 25, 1383–1399 CrossRef CAS.
- C. Xie, Y. Ma, J. Yang, B. Zhang, W. Luo, S. Feng, J. Zhang, G. Wang, X. He and Z. Zhang, Effects of foliar applications of ceria nanoparticles and CeCl3 on common bean (Phaseolus vulgaris), Environ. Pollut., 2019, 250, 530–536 CrossRef CAS.
- H. Xing, X. Yu, J. Sun, G. Lu, M. Zhu, J. Liang, L. Jin and L. Zhu, Interaction between Phthalate Ester and Rice Plants: Novel Transformation Pathways and Metabolic-Network Perturbations, Environ. Sci. Technol., 2023, 57, 8870–8882 CrossRef CAS PubMed.
- A. Chahardoli, H. Sharifan, N. Karimi and S. N. Kakavand, Uptake, translocation, phytotoxicity, and hormetic effects of titanium dioxide nanoparticles (TiO2NPs) in Nigella arvensis L, Sci. Total Environ., 2022, 806, 151222 CrossRef CAS.
- J. Li, X. Cao, X. Jia, L. Liu, H. Cao, W. Qin and M. Li, Iron Deficiency Leads to Chlorosis Through Impacting Chlorophyll Synthesis and Nitrogen Metabolism in Areca catechu L., Front. Plant Sci., 2021, 12, 710093 CrossRef PubMed.
- S. Jalil, S. M. S. Alghanem, A. A. Al-Huqail, M. M. Nazir, F. Zulfiqar, T. Ahmed, S. Ali, A. H. A. Abeed, K. H. M. Siddique and X. Jin, Zinc oxide nanoparticles mitigated the arsenic induced oxidative stress through modulation of physio-biochemical aspects and nutritional ions homeostasis in rice (Oryza sativa L.), Chemosphere, 2023, 338, 139566 CrossRef CAS.
- Y. Zhu, Z. Li, J. Shen, K. Wu, P. Zhao, Z. Wu, Z. Liu, J. Yang, H. Liu, C. Rensing and R. Feng, Toxicity of different forms of antimony to rice plants: Photosynthetic electron transfer, gas exchange, photosynthetic efficiency, and carbon assimilation combined with metabolome analysis, J. Hazard. Mater., 2022, 437, 129433 CrossRef CAS PubMed.
- C. Mony, P. Kaur, J. E. Rookes, D. L. Callahan, S. V. Eswaran, W. Yang and P. K. Manna, Nanomaterials for enhancing photosynthesis: interaction with plant photosystems and scope of nanobionics in agriculture, Environ. Sci.: Nano, 2022, 9, 3659–3683 RSC.
- A. Wang, Q. Jin, X. Xu, A. Miao, J. C. White, J. L. Gardea-Torresdey, R. Ji and L. Zhao, High-Throughput Screening for Engineered Nanoparticles That Enhance Photosynthesis Using Mesophyll Protoplasts, J. Agric. Food Chem., 2020, 68, 3382–3389 CrossRef CAS PubMed.
- K. Xu, Z. Li, P. Juneau, F. Xiao, Y. Lian, W. Zhang, L. Shu, H. Jiang, K. Zhang, C. Wang, S. Wang, Q. Yan and Z. He, Toxic and protective mechanisms of cyanobacterium Synechocystis sp. in response to titanium dioxide nanoparticles, Environ. Pollut., 2021, 274, 116508 CrossRef CAS PubMed.
- M. Bakshi, C. Line, D. E. Bedolla, R. J. Stein, R. Kaegi, G. Sarret, A. E. Pradas Del Real, H. Castillo-Michel, P. C. Abhilash and C. Larue, Assessing the impacts of sewage sludge amendment containing nano-TiO2 on tomato plants: A life cycle study, J. Hazard. Mater., 2019, 369, 191–198 CrossRef CAS.
- Y. Ye, K. Cota-Ruiz, J. A. Hernández-Viezcas, C. Valdés, I. A. Medina-Velo, R. S. Turley, J. R. Peralta-Videa and J. L. Gardea-Torresdey, Manganese Nanoparticles Control Salinity-Modulated Molecular Responses in Capsicum annuum L. through Priming: A Sustainable Approach for Agriculture, ACS Sustainable Chem. Eng., 2020, 8, 1427–1436 CrossRef.
- G. Hu, M. Ma, Z. Batool, L. Sheng, Z. Cai, Y. Liu and Y. Jin, Gel properties of heat-induced transparent hydrogels from ovalbumin by acylation modifications, Food Chem., 2022, 369, 130912 CrossRef CAS.
- Q. Xiong, Y. S. Liu, L. X. Hu, Z. Q. Shi and G. G. Ying, Levofloxacin and sulfamethoxazole induced alterations of biomolecules in Pseudokirchneriella subcapitata, Chemosphere, 2020, 253, 126722 CrossRef CAS PubMed.
- T. T. Wang, S. Wang, S. Shao, X. D. Wang, D. Y. Wang, Y. S. Liu, C. J. Ge, G. G. Ying and Z. B. Chen, Perfluorooctanoic acid (PFOA)-induced alterations of biomolecules in the wetland plant Alisma orientale, Sci. Total Environ., 2022, 820, 153302 CrossRef CAS PubMed.
- Y. L. Wu, Q. Xiong, B. Wang, Y. S. Liu, P. L. Zhou, L. X. Hu, F. Liu and G. G. Ying, Screening of structural and functional alterations in duckweed (Lemna minor) induced by per- and polyfluoroalkyl substances (PFASs) with FTIR spectroscopy, Environ. Pollut., 2023, 317, 120671 CrossRef CAS.
- Y. Zhu, Y. Jin, X. Liu, T. Miao, Q. Guan, R. Yang and J. Qu, Insight into interactions of heavy metals with livestock manure compost-derived dissolved organic matter using EEM-PARAFAC and 2D-FTIR-COS analyses, J. Hazard. Mater., 2021, 420, 126532 CrossRef CAS.
- Y. Tao, H. Shi, Y. Jiao, S. Han, M. S. Akindolie, Y. Yang, Z. Chen and Y. Zhang, Effects of humic acid on the biodegradation of di-n-butyl phthalate in mollisol, J. Cleaner Prod., 2020, 249, 119404 CrossRef CAS.
- B. Ahmed, A. Rizvi, A. Syed, A. M. Elgorban, M. S. Khan, H. A. Al-Shwaiman, J. Musarrat and J. Lee, Differential responses of maize (Zea mays) at the physiological, biomolecular, and nutrient levels when cultivated in the presence of nano or bulk ZnO or CuO or Zn2+ or Cu2+ ions, J. Hazard. Mater., 2021, 419, 126493 CrossRef CAS.
- Z. Cao, C. Stowers, L. Rossi, W. Zhang, L. Lombardini and X. Ma, Physiological effects of cerium oxide nanoparticles on the photosynthesis and water use efficiency of soybean (Glycine max (L.) Merr.), Environ. Sci.: Nano, 2017, 4, 1086–1094 RSC.
- Y. Li, X. Xu, B. Lei, J. Zhuang, X. Zhang, C. Hu, J. Cui and Y. Liu, Magnesium-nitrogen co-doped carbon dots enhance plant growth through multifunctional regulation in photosynthesis, Chem. Eng. J., 2021, 422, 130114 CrossRef CAS.
- Y. Dong, M. Gao, W. Qiu and Z. Song, Effects of microplastic on arsenic accumulation in Chlamydomonas reinhardtii in a freshwater environment, J. Hazard. Mater., 2021, 405, 124232 CrossRef CAS.
- C. Song, X. Han, Q. Yin, D. Chen, H. Li and S. Li, Performance intensification of CO2 absorption and microalgae conversion (CAMC) hybrid system via low temperature plasma (LTP) treatment, Sci. Total Environ., 2021, 801, 149791 CrossRef CAS PubMed.
- P. Li, A. Wang, W. Du, L. Mao, Z. Wei, S. Wang, H. Yuan, R. Ji and L. Zhao, Insight into the interaction between Fe-based nanomaterials and maize (Zea mays) plants at metabolic level, Sci. Total Environ., 2020, 738, 139795 CrossRef CAS PubMed.
- D. Li, W. Li, H. Zhang, X. Zhang, J. Zhuang, Y. Liu, C. Hu and B. Lei, Far-Red Carbon Dots as Efficient Light-Harvesting Agents for Enhanced Photosynthesis, ACS Appl. Mater. Interfaces, 2020, 12, 21009–21019 CrossRef CAS.
- Y. Shen, J. Li, R. Gu, X. Zhan and B. Xing, Proteomic analysis for phenanthrene-elicited wheat chloroplast deformation, Environ. Int., 2019, 123, 273–281 CrossRef CAS.
- J. Lian, L. Zhao, J. Wu, H. Xiong, Y. Bao, A. Zeb, J. Tang and W. Liu, Foliar
spray of TiO2 nanoparticles prevails over root application in reducing Cd accumulation and mitigating Cd-induced phytotoxicity in maize (Zea mays L.), Chemosphere, 2020, 239, 124794 CrossRef CAS.
- Y. Wang, L. Xiang, F. Wang, Z. Wang, Y. Bian, C. Gu, X. Wen, F. O. Kengara, A. Schäffer, X. Jiang and B. Xing, Positively Charged Microplastics Induce Strong Lettuce Stress Responses from Physiological, Transcriptomic, and Metabolomic Perspectives, Environ. Sci. Technol., 2022, 56, 16907–16918 CrossRef CAS PubMed.
- T. E. Shull, J. Kurepa and J. A. Smalle, Anatase TiO2 Nanoparticles Induce Autophagy and Chloroplast Degradation in Thale Cress (Arabidopsis thaliana), Environ. Sci. Technol., 2019, 53, 9522–9532 CrossRef CAS.
- M. Izumi, J. Hidema, S. Wada, E. Kondo, T. Kurusu, K. Kuchitsu, A. Makino and H. Ishida, Establishment of monitoring methods for autophagy in rice reveals autophagic recycling of chloroplasts and root plastids during energy limitation, Plant Physiol., 2015, 167, 1307–1320 CrossRef CAS PubMed.
- J. Y. Yue, Y. J. Wang, J. L. Jiao and H. Z. Wang, Silencing of ATG2 and ATG7 promotes programmed cell death in wheat via inhibition of autophagy under salt stress, Ecotoxicol. Environ. Saf., 2021, 225, 112761 CrossRef CAS PubMed.
- R. Dixit, L. Agrawal, S. P. Singh, Prateeksha, P. C. Singh, V. Prasad and P. S. Chauhan, Paenibacillus lentimorbus induces autophagy for protecting tomato from Sclerotium rolfsii infection, Microbiol. Res., 2018, 215, 164–174 CrossRef.
|
This journal is © The Royal Society of Chemistry 2024 |