DOI:
10.1039/C6RA27299A
(Paper)
RSC Adv., 2017,
7, 3066-3071
Efficient two-step synthesis of water soluble BODIPY–TREN chemosensors for copper(II) ions†
Received
24th November 2016
, Accepted 20th December 2016
First published on 13th January 2017
Abstract
Two simple fluorescent sensors for Cu(II) ions were synthesized in two reaction steps, using recently developed C–H functionalization reactions for boron dipyrrins, starting from a standard 8-aryl-BODIPY dye and a tris(2-aminoethyl)amine (TREN) ligand. At pH 5, the resulting BODIPY–TREN conjugates are demonstrated to be promising, highly selective, water soluble, Cu(II) sensors that can permeate the cell membrane.
Introduction
BODIPY dyes (4,4-difluoro-4-bora-3a,4a-diaza-s-indacenes, also known as boron dipyrrins)1 are an important class of fluorophores with many excellent characteristics, such as a bright fluorescence in the visible spectral range and a good robustness towards light and chemicals.2 Another major attraction of these dyes is their rich functionalization chemistry,3 allowing practically unlimited structural modification which leads to sophisticated dyes with fine-tuned chemical and spectroscopic properties.4 Recently, several C–H functionalization strategies for boron dipyrrins have been reported.5–7 Particularly promising in this regard are the nucleophilic substitution of hydrogen6 and the radical functionalization reactions7 developed in our group. Their mild reaction conditions and broad substrate scopes make these protocols useful strategies to efficiently functionalize BODIPY dyes. These excellent properties and functionalization potential contribute to the growing importance of boron dipyrrins, as is clearly illustrated by the numerous applications being reported for these dyes, including their use as chemosensors.8
Fluorescent chemosensors for the recognition and measurement of transition metal ions are very sensitive and indispensable tools in chemistry, life science and biotechnology.9 For instance, the design of Cu(II) sensors has received much attention10,11 because of the importance of Cu(II) in environmental and biological systems. In fact, Cu(II) is the third most abundant transition metal in the human body,10 is required as a cofactor for many fundamental biological processes,12 is associated with neurodegenerative diseases, such as Alzheimer's disease,10,12,13 and has been identified as an environmental pollutant.11 Recently, several BODIPY based Cu(II) sensors have been reported.14 However, their synthesis required a long multi-step protocol and/or unstable intermediates. Moreover, most BODIPY based Cu(II) sensors are poorly water-soluble and are thus often characterized in non-aqueous systems or in mixtures of organic solvents with water. However, for many applications, water solubility is an important property of Cu(II) sensors.
The synthesis of new highly fluorescent BODIPY Cu(II) sensors might be improved by using the efficient C–H functionalization protocols developed for boron dipyrrins. Herein, we report the synthesis of two simple Cu(II) sensors based on a tris(2-aminoethyl)amine (TREN)15 ligand as the receptor moiety starting from a standard 8-aryl-BODIPY dye 1 using the C–H functionalization reactions recently developed by our group.6,7 The interaction of these sensors with protons and transition metal ions and their ability to permeate the cell membrane were investigated and their high selectivity for Cu(II) was demonstrated.
Results and discussion
Synthesis
The desired BODIPY–TREN conjugates were synthesized starting from an accessible 8-aryl-BODIPY dye 1.2,6b The TREN ligand could be directly incorporated onto its 3-position using an oxidative nucleophilic substitution of hydrogen6a in DMF under an oxygen atmosphere at room temperature. Unfortunately, the formed product turned out to be unstable and decomposed during work up. This might be caused by the two remaining primary amines of the formed BODIPY–TREN conjugate, as these might react further at the free 5-position. Blocking the 5-position with a substituent should prevent this side reaction, allowing the isolation of the desired BODIPY–TREN conjugate (Scheme 1). A phenyl or a cyclohexyl substituent can easily be placed on the 5-position using the radical functionalization reactions developed in our group.7 The phenyl group was introduced via a ferrocene catalyzed radical arylation7a with benzenediazonium tetrafluoroborate forming 5-phenyl-BODIPY 2 in a good yield of 58%. 5-Cyclohexyl-BODIPY 3, on the other hand, was synthesized in an excellent yield of 77% via an oxidative radical alkylation7b with potassium cyclohexyltrifluoroborate. Both 5-substituted dyes were successfully converted to the corresponding stable BODIPY–TREN conjugates 4 and 5 in a yield of about 60% using the oxidative nucleophilic substitution of hydrogen reaction with an excess of the TREN polyamine.
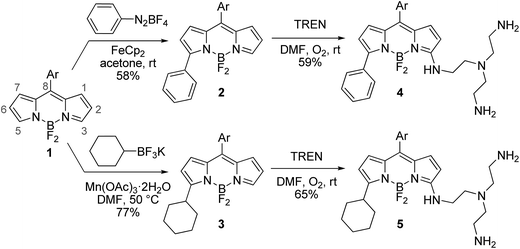 |
| Scheme 1 Synthesis of BODIPY–TREN conjugates 4 and 5 (Ar = 2,6-dichlorophen-1-yl, TREN = tris(2-aminoethyl)amine). | |
Influence of the pH on the spectroscopic properties
Both synthesized BODIPY–TREN conjugates 4 and 5 are soluble in water, hence their spectroscopic properties were determined in this medium (Table 1). Both compounds absorb visible light at a similar wavelength around 495 nm. However, the increased conjugation length in the 5-phenyl compound 4 causes a bathochromic shift in the emission wavelength of 24 nm compared to the 5-cyclohexyl derivative 5, which emits light at 551 nm, resulting in a larger Stokes shift for the 5-phenyl dye 4. Hence, the 5-substituent can be used to fine tune the emission wavelength of the resulting BODIPY–TREN conjugates. The Stokes shifts of both compounds are rather large compared to typical boron dipyrrin dyes, although 3-amino-BODIPYs often display large Stokes shifts in polar media.14f,16 In contrast to the absorption and emission wavelengths, the broadness of the absorption and emission peaks and the fluorescence quantum yield of both compounds are very similar. Both dyes have high fluorescence quantum yields (around 0.6) at pH 7.4 and pH 5, with the one at pH 7.4 being slightly lower.
Table 1 Spectroscopic data of BODIPY–TREN conjugates 4 and 5 in water
Compound |
λ
abs,max (nm) |
λ
em,max (nm) |
Stokes shift (cm−1) |
fwhmabs (cm−1) |
fwhmem (cm−1) |
Φ
f
a
,
b (pH 7.4) |
Φ
f
a
,
b (pH 5) |
Aqueous sodium cacodylate buffer was used.
Absolute fluorescence quantum yields obtained using an integrating sphere accessory.
1 equivalent of metal ion was added to the free ligand.
|
4
|
493 |
575 |
2893 |
3773 |
1880 |
0.58 |
0.62 |
5
|
496 |
551 |
2012 |
3487 |
1740 |
0.57 |
0.58 |
4 + Cu(II)c |
488 |
574 |
3070 |
4123 |
1768 |
0.02 |
0.42 |
5 + Cu(II)c |
496 |
549 |
1967 |
3913 |
1554 |
0.02 |
0.39 |
4 + Zn(II)c |
488 |
576 |
3116 |
3957 |
2001 |
0.40 |
0.60 |
5 + Zn(II)c |
493 |
552 |
2189 |
3608 |
1772 |
0.43 |
0.64 |
The primary amines of the synthesized ligands 4 and 5 are susceptible to protonation, hence the effect of the pH on the emission spectra was studied (Fig. 1 and 2). Both compounds displayed a decrease in fluorescence intensity when going from an acidic to a basic pH, with the largest change in the intensity occurring between pH 8 and pH 10 (Fig. 2 and S3†). The emission maxima, on the other hand, were unaffected by the pH (Fig. 1 and S2†). The fluorescence quenching as the degree of protonation of the ligand decreases, is a phenomenon that has been widely observed in similar compounds15 and has been attributed to a photoinduced electron transfer (PET) process from the lone pairs of the amines to the excited fluorophore.9 Protonation of the primary amines of the BODIPY–TREN conjugates blocks this PET-mediated quenching and hence leads to fluorescent compounds.
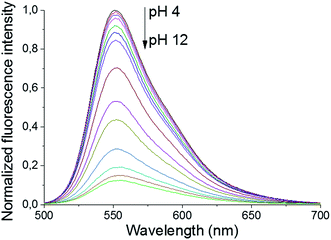 |
| Fig. 1 pH-Dependence of the fluorescent emission spectra of compound 5 (10 μM, λex = 472 nm) in water. | |
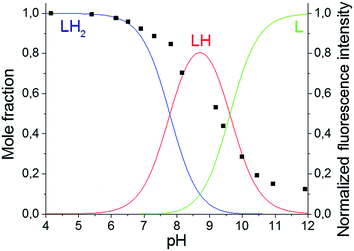 |
| Fig. 2 Fluorescence emission pH titration curve of compound 5 (10 μM, λex = 472 nm, λem = 552 nm) in water (black ■) and mole fraction distribution diagram for the various protonated species of this ligand (solid lines). | |
Using the pH titration data, two stepwise protonation constants could be calculated (Table 2). However, a third protonation step was not observed in the pH range studied.3–12 More acidic and basic pH values were not tested because BODIPY dyes are known to decompose in strongly acidic and strongly basic conditions.17 The two observed protonation steps correspond to the two primary amines of the TREN substituent. Protonation of the amine directly connected to the pyrrole ring is unlikely to occur due to the electron withdrawing nature of the BODIPY core.2 Furthermore, it is also not likely that the tertiary amine will be protonated before the primary amines as it is well known that tertiary nitrogen atoms are less basic in aqueous solution than primary and secondary ones.18 The protonation constants for the two synthesized BODIPY–TREN conjugates 4 and 5 (Table 2) are similar to each other and to the constants of other primary amines in general.18
Table 2 Stepwise protonation constants for compounds 4 and 5 and stepwise stability constants for their Cu(II) and Zn(II) complexes determined from fluorescence titration experimentsa,b
Reaction |
4
|
5
|
Logarithms of the constants are reported.
Numbers in parentheses are standard deviations in the last significant figure.
|
L + H+ ⇄ HL+ |
9.18(2) |
9.618(7) |
HL+ + H+ ⇄ H2L2+ |
8.6(1) |
7.78(3) |
L + Cu2+ ⇄ CuL2+ |
13.13(4) |
12.95(5) |
L + CuL2+ ⇄ CuL22+ |
10.9(1) |
10.2(1) |
L + Zn2+ ⇄ ZnL2+ |
7.71(2) |
7.24(2) |
Based on the determined protonation constants, the distribution diagrams for the different protonated species could be calculated in function of the pH (Fig. 2 and S3†). Comparing these distribution diagrams with the pH titration curves shows that the decrease in emission intensity, when going from acidic to basic pH, corresponds with a decreasing amount of fluorescent protonated species and an increasing amount of the PET-quenched non-protonated ligand.
Influence of the presence of a metal ion on the spectroscopic properties
The spectroscopic characteristics of the synthesized BODIPY–TREN conjugates in water in the presence of two different transition metal ions were studied next. Cu(II) and Zn(II) were chosen as representative examples (Table 1). Adding one equivalent of metal ion to ligands 4 and 5 did not significantly affect the position of the absorption and emission maxima, the Stokes shifts or the broadness of the absorption and emission peaks. However, the presence of a metal ion did affect the intensity of the emission spectra, resulting in lower quantum yields of fluorescence. At pH 7.4, addition of Cu(II) almost completely quenched the fluorescence of the BODIPY–TREN conjugates. Addition of Zn(II) at the same pH reduced the fluorescence quantum yield to a lesser extent. However, the differences in quantum yields of the free ligands and the complexes are not as pronounced at pH 5. At this pH, Cu(II) reduced the fluorescence quantum yield from 0.6 to 0.4 while Zn(II) had no noticeable effect.
Repeating the pH titration experiments in the presence of one equivalent of these two metal ions resulted again in a quenching of fluorescence at basic pH (Fig. 3). However, the titration curves themselves are different. The largest change in emission intensity in the presence of Cu(II) ions was already observed at a lower pH, between pH 4 and pH 6, in comparison to the free ligands (Fig. 3 and S6†). Furthermore, the Cu(II) complexes quenched the fluorescence of the BODIPY core stronger than the unprotonated free ligands. This effect, called a chelation enhanced quenching (CHEQ) effect, is often observed for Cu(II) complexes.9b In contrast, Zn(II) does not strongly shift the pH range where the largest change in emission intensity occurs (Fig. 3 and S9†). Moreover, at basic pH a larger emission intensity was observed for the Zn(II) complexes in comparison to the free ligands. This indicates that the unprotonated free ligands resulted in a stronger quenching of the fluorescence than the corresponding Zn(II) complexes. Hence, Zn(II) displayed a chelation enhanced fluorescence (CHEF) effect.9b
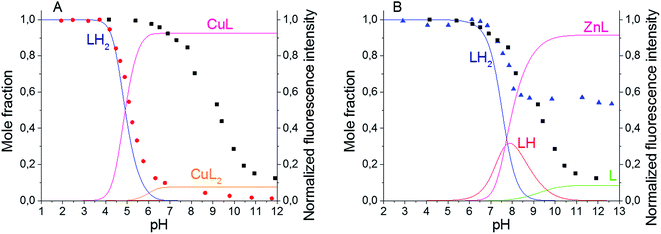 |
| Fig. 3 Fluorescence emission pH titration curves of compound 5 (10 μM, λex = 472 nm, λem = 552 nm) in water in the absence (black ■) or presence of 1 equivalent of metal ion, (A) Cu(II) (red ●) and (B) Zn(II) (blue ▲), and mole fraction distribution diagrams for the various species of this ligand under these conditions (solid lines). | |
Based on these titration data and on the calculated protonation constants, the stability constants for the Cu(II) and Zn(II) complexes of the BODIPY–TREN conjugates 4 and 5 could be calculated (Table 2). In the case of Cu(II), two different stability constants were found, one for the mono-(BODIPY–TREN)-complex [CuL]2+ and one for the bis-(BODIPY–TREN)-complex [CuL2]2+. However, only one stability constant could be obtained for the Zn(II) complexes.
Using these stability constants together with the protonation constants, the distribution diagrams for the different complexes and protonated species in the presence of one equivalent of metal ion could be calculated in function of the pH (Fig. 3, S6 and S9). Comparing these distribution diagrams with the pH titration curves shows again that the decrease in emission intensity, when going from acidic to basic pH, corresponds with a decreasing amount of fluorescent protonated species and an increasing amount of (partially) quenched complexes.
Afterwards, the effect of the Cu(II) and Zn(II) concentrations on the emission spectra was investigated at a constant pH of 7.4. Because both BODIPY–TREN conjugates 4 and 5 have the same spectroscopic properties, with the exception of their emission maxima, and the same response to pH and to the presence of Cu(II) and Zn(II), the metal titration experiments were done only for ligand 5 (Fig. 4). Similar to protonation, addition of Cu(II) influenced only the emission intensity resulting in a weaker fluorescence at a higher Cu(II) concentration (Fig. S10A†). Addition of Zn2+ had the same effect, however, in this case, also a slight bathochromic shift of a few nanometers was observed at higher Zn(II) concentrations (Fig. S10B†). The main difference between the two metal ions is that for Cu(II) the fluorescence intensity decreases more strongly and levels out after addition of about 0.5 equivalents of Cu(II). This intensity for the Zn(II) complex, on the other hand, levels out after about one equivalent of Zn(II) (Fig. 4).
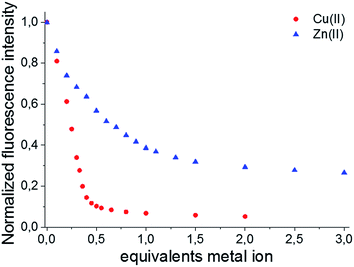 |
| Fig. 4 Fluorescence emission metal titration curve of compound 5 (10 μM, λex = 472 nm, λem = 552 nm) using Cu(II) (red ●) and Zn(II) (blue ▲) in an aqueous sodium cacodylate buffer solution (0.05 M, pH 7.4). | |
This difference, in amount of equivalents of metal ion, could be explained if a 2
:
1 stoichiometry for the 5–Cu(II) complex and a 1
:
1 stoichiometry for 5–Zn(II) complex are assumed. This hypothesis was verified, by determining the stoichiometry of the Cu(II) and Zn(II) BODIPY–TREN complexes of ligand 5, using a Job plot (Fig. S11†). Furthermore, these results agree with the two stability constants for the Cu(II) complex and the one stability constant for the Zn(II) complex that resulted from the calculations from the pH titration experiments (see above).
Selectivity towards Cu(II)
Given the difference in stability constants and stoichiometry of the Cu(II) and Zn(II) complexes of the synthesized BODIPY–TREN conjugates 4 and 5 it might be possible to use these ligands as ion-selective fluorescent probes. To this end, the fluorescence response of the two synthesized ligands towards an excess of a selection of metal ions was investigated at pH 7.4 and at pH 5 (Fig. 5). At pH 7.4, about half of the tested metal ions caused a reduction in the fluorescence intensity (Fig. 5A). Cu(II) had the largest effect on this intensity, almost completely quenching the fluorescence of the two BODIPY–TREN conjugates. Co(II), Ni(II), Zn(II) and Hg(II) also significantly (by about 60%) reduced the emission intensity. Lastly, Cd(II) reduced the fluorescence to a lesser extent (by 10–20%). The other metal ions (Na(I), Mn(II), Fe(III) and Pb(II)) did not create a significant reduction in fluorescence intensity. Most ions did not influence the emission maxima, however complexation to Hg(II) did lead to a bathochromic shift of about 10 nm for both ligands (Fig. S12†). Moreover, a slight bathochromic shift of a few nanometers was detected upon addition of an excess of Zn(II) to ligand 5, yet a similar effect was not observed for ligand 4 (Fig. S12†).
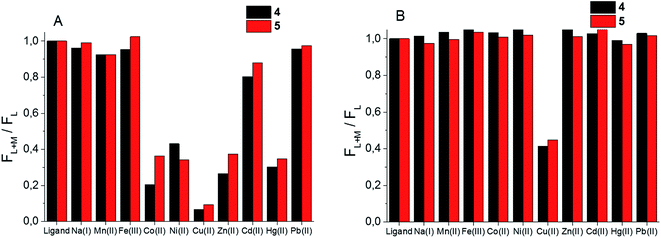 |
| Fig. 5 Fluorescence response of ligands 4 (10 μM, λex = 472 nm, λem = 576 nm) and 5 (10 μM, λex = 472 nm, λem = 552 nm) to an excess (4 equivalents) of selected metal ions in an aqueous sodium cacodylate buffer solution (0.05 M) at (A) pH 7.4 and (B) pH 5. FL and FL+M denote the normalized fluorescence intensity of the free ligand and the ligand in the presence of the metal ion, respectively. | |
The effect of the presence of an excess of these metal ions is quite different at pH 5. At this pH only Cu(II) resulted in a reduction of the emission intensity, all other tested metal ions left the fluorescence of the free ligand intact (Fig. 5B). This is probably because only Cu(II) binds strongly enough with the BODIPY–TREN conjugates 4 and 5 to successfully compete with protons at pH 5. The fluorescence quenching of Cu(II) at this pH is not as strong as at pH 7.4, reducing the emission intensity to about 40%, compared to the free ligands, instead of almost completely quenching it. Nonetheless, the good selectivity for Cu(II) ions at pH 5 means that the synthesized BODIPY–TREN conjugates 4 and 5 are promising fluorescent sensors for Cu(II).
Cell permeability study
The ability of ligands 4 and 5 to permeate cellular membranes was checked with HeLa cell cultures by flow cytometry and fluorescence spectroscopy (Fig. 6). Both compounds were incubated for 24 and 48 hours showing their incorporation in cells as denoted by the observation of the fluorescence of the BODIPY–TREN conjugates 4 and 5 in the 550–570 nm range (Fig. S15–S18†). On the other hand, the flow cytometry technique reveals a normal cellular growth up to about 30 μM concentrations of the compounds (Fig. S13 and S14†).
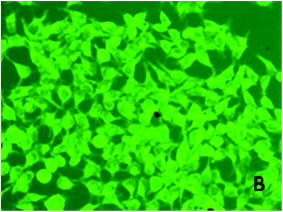 |
| Fig. 6 Confocal fluorescence image of live HeLa cells incubated with 5 μM of ligand 5 for 24 hours. | |
Conclusion
Two simple and novel fluorescent sensors for Cu(II) ions based on a BODIPY fluorophore and a TREN ligand were synthesized in an efficient way from a standard boron dipyrrin dye using C–H functionalization reactions. The ease and resulting high yields of these reactions demonstrated the utility of nucleophilic substitution of hydrogen and radical reactions in the construction of functionalized BODIPY dyes. The synthesized BODIPY–TREN conjugates are water soluble compounds that can permeate the cell membrane. The interaction of these ligands with different transition metal ions was investigated and it was shown that at pH 5 they possess a high selectivity for Cu(II) ions. Hence, these BODIPY–TREN conjugates are promising fluorescent sensors for Cu(II) at this pH.
Acknowledgements
The FWO-Vlaanderen (travel grant V404416N), KU Leuven, and Ministerie voor Wetenschapsbeleid are thanked for continuing financial support. Financial support by the Spanish MINECO and FEDER (Projects and Consolider-Ingenio, Project CSD2010-000652010 and CTQ2013-48917-C3-1-P and “Unidad de Excelencia María de Maeztu” MDM-2015-0538) and Generalitat Valenciana (PROMETEOII 2015/002) are also gratefully acknowledged.
Notes and references
- A. Treibs and F.-H. Kreuzer, Liebigs Ann. Chem., 1968, 718, 208–223 CrossRef CAS.
-
(a) A. Loudet and K. Burgess, Chem. Rev., 2007, 107, 4891–4932 CrossRef CAS;
(b) G. Ulrich, R. Ziessel and A. Harriman, Angew. Chem., Int. Ed., 2008, 47, 1184–1201 CrossRef CAS.
-
(a) Y. Ni and J. Wu, Org. Biomol. Chem., 2014, 12, 3774–3791 RSC;
(b) V. Lakshmi, M. R. Rao and M. Ravikanth, Org. Biomol. Chem., 2015, 13, 2501–2517 RSC;
(c) N. Boens, B. Verbelen and W. Dehaen, Eur. J. Org. Chem., 2015, 6577–6595 CrossRef CAS.
- H. Lu, J. Mack, Y. Yang and Z. Shen, Chem. Soc. Rev., 2014, 43, 4778–4823 RSC.
-
(a) C. Thivierge, R. Bandichhor and K. Burgess, Org. Lett., 2007, 9, 2135–2138 CrossRef CAS;
(b) J. Chen, M. Mizumura, H. Shinokubo and A. Osuka, Chem.–Eur. J., 2009, 15, 5942–5949 CrossRef CAS;
(c) S. M. Crawford and A. Thompson, Heterocycles, 2011, 83, 311–322 CrossRef CAS;
(d) B. Verbelen, V. Leen, L. Wang, N. Boens and W. Dehaen, Chem. Commun., 2012, 48, 9129–9131 RSC;
(e) L. Luo, D. Wu, W. Li, S. Zhang, Y. Ma, S. Yan and J. You, Org. Lett., 2014, 16, 6080–6083 CrossRef CAS;
(f) X. Zhou, Q. Wu, Y. Yu, C. Yu, E. Hao, Y. Wei, X. Mu and L. Jiao, Org. Lett., 2016, 18, 736–739 CrossRef CAS.
-
(a) V. Leen, V. Z. Gonzalvo, W. M. De Borggraeve, N. Boens and W. Dehaen, Chem. Commun., 2010, 46, 4908–4910 RSC;
(b) V. Leen, M. Van der Auweraer, N. Boens and W. Dehaen, Org. Lett., 2011, 13, 1470–1473 CrossRef CAS.
-
(a) B. Verbelen, S. Boodts, J. Hofkens, N. Boens and W. Dehaen, Angew. Chem., Int. Ed., 2015, 54, 4612–4616 CrossRef CAS;
(b) B. Verbelen, L. C. D. Rezende, S. Boodts, J. Jacobs, L. Van Meervelt, J. Hofkens and W. Dehaen, Chem.–Eur. J., 2015, 21, 12667–12675 CrossRef CAS.
-
(a) N. Boens, V. Leen and W. Dehaen, Chem. Soc. Rev., 2012, 41, 1130–1172 RSC;
(b) A. N. Kursunlu, E. Şahin and E. Güler, RSC Adv., 2015, 5, 5951–5957 RSC;
(c) A. N. Kursunlu, RSC Adv., 2015, 5, 41025–41032 RSC;
(d) W. Qin, W. Dou, V. Leen, W. Dehaen, M. Van der Auweraer and N. Boens, RSC Adv., 2016, 6, 7806–7816 RSC;
(e) N. Kaur, P. Kaur, G. Bhatia, K. Singh and J. Singh, RSC Adv., 2016, 6, 82810–82816 RSC.
-
(a) A. P. Silva, H. Q. N. Gunaratne, T. Gunnlaugsson, A. J. M. Huxley, C. P. McCoy, J. T. Rademacher and T. E. Rice, Chem. Rev., 1997, 97, 1515–1566 CrossRef;
(b) M. Formica, V. Fusi, L. Giorgi and M. Micheloni, Coord. Chem. Rev., 2012, 256, 170–192 CrossRef CAS.
- E. L. Que, D. W. Domaille and C. J. Chang, Chem. Rev., 2008, 108, 1517–1549 CrossRef CAS.
- R. Kramer, Angew. Chem., Int. Ed., 1998, 37, 772–773 CrossRef CAS.
-
(a) H. Tapiero, D. M. Townsend and K. D. Tew, Biomed. Pharmacol. J., 2003, 57, 386–398 CrossRef CAS;
(b) E. Gaggelli, H. Kozlowski, D. Valensin and G. Valensin, Chem. Rev., 2006, 106, 1995–2044 CrossRef CAS.
- K. J. Barnham, C. L. Master and A. I. Bush, Nat. Rev. Drug Discovery, 2004, 3, 205–214 CrossRef CAS.
-
(a) Y. Mei, P. A. Bentley and W. Wang, Tetrahedron Lett., 2006, 47, 2447–2449 CrossRef CAS;
(b) X. Qi, E. J. Jun, L. Xu, S.-J. Kim, J. S. J. Hong, Y. J. Yoon and J. Yoon, J. Org. Chem., 2006, 71, 2881–2884 CrossRef CAS;
(c) S. H. Choi, K. Pang, K. Kim and D. G. Churchill, Inorg. Chem., 2007, 46, 10564–10577 CrossRef CAS;
(d) V. Csokai, M. Kádár, D. L. H. Mai, O. Varga, K. Tóth, M. Kubinyi, A. Grün and I. Bitter, Tetrahedron, 2008, 64, 1058–1063 CrossRef CAS;
(e) L. Jiao, J. Li, S. Zhang, C. Wei, E. Hao and M. G. H. Vicente, New J. Chem., 2009, 33, 1888–1893 RSC;
(f) S. Yin, V. Leen, S. Van Snick, N. Boens and W. Dehaen, Chem. Commun., 2010, 46, 6329–6331 RSC;
(g) H. Y. Lee, H. Son, J. M. Lim, J. Oh, D. Kang, W. S. Han and J. H. Jung, Analyst, 2010, 135, 2022–2027 RSC;
(h) Z. Li, Q.-Y. Chen, P.-D. Wang and Y. Wu, RSC Adv., 2013, 3, 5524–5528 RSC;
(i) R. Xie, Y. Yi, Y. He, X. Liu and Z.-X. Liu, Tetrahedron, 2013, 69, 8541–8546 CrossRef CAS;
(j) A. N. Kursunlu, Z. E. Koc, A. Y. Obali and E. Güler, J. Lumin., 2014, 149, 215–220 CrossRef CAS.
-
(a) L. Prodi, M. Montalti, N. Zaccheroni, F. Dallavalle, G. Folesani, M. Lanfranchi, R. Corradini, S. Pagliari and R. Marchelli, Helv. Chim. Acta, 2001, 84, 690–706 CrossRef CAS;
(b) J. García-Martín, R. López-Garzón, M. L. Godino-Salido, R. Cuesta-Martos, M. D. Gutiérrez-Valero, P. Arranz-Mascarós and H. Stoeckli-Evans, Eur. J. Inorg. Chem., 2005, 3093–3103 CrossRef;
(c) M. Dakanali, E. Roussakis, A. R. Kay and H. E. Katerinopoulos, Tetrahedron Lett., 2005, 46, 4193–4196 CrossRef CAS;
(d) M. L. Godino-Salido, R. López-Garzón, P. Arranz-Mascarós, M. D. Gutiérrez-Valero, A. Santiago-Medina and J. García-Martín, Polyhedron, 2009, 28, 3781–3787 CrossRef CAS;
(e) E. B. Veale, J. A. Kitchen and T. Gunnlaugsson, Supramol. Chem., 2013, 25, 101–108 CrossRef CAS.
-
(a) T. Rohand, M. Baruah, W. Qin, N. Boens and W. Dehaen, Chem. Commun., 2006, 266–268 RSC;
(b) W. Qin, V. Leen, T. Rohand, W. Dehaen, P. Dedecker, M. Van der Auweraer, K. Robeyns, L. Van Meervelt, D. Beljonne, B. Van Averbeke, J. N. Clifford, K. Driesen, K. Binnemans and N. Boens, J. Phys. Chem. A, 2009, 113, 439–447 CrossRef CAS.
-
(a) L. Yang, R. Simionescu, A. Lough and H. Yan, Dyes Pigm., 2011, 91, 264–267 CrossRef CAS;
(b) E. V. Rumyantsev, S. N. Alyoshin and Y. S. Marfin, Inorg. Chim. Acta, 2013, 408, 181–185 CrossRef CAS.
- A. Bencini, A. Bianchi, E. Garcia-España, M. Micheloni and J. A. Ramirez, Coord. Chem. Rev., 1999, 188, 97–156 CrossRef CAS.
Footnotes |
† Electronic supplementary information (ESI) available: Experimental procedures, characterization data, absorption and emission spectra, titration data, Job plots and cell permeability study. See DOI: 10.1039/c6ra27299a |
‡ Current address: Institute of Nanoscience & Nanotechnology, NCSR “Demokritos”, Terma Patr. Gregoriou & Neapoleos, Aghia Paraskevi, 15310, Attikis, Greece. |
|
This journal is © The Royal Society of Chemistry 2017 |
Click here to see how this site uses Cookies. View our privacy policy here.