DOI:
10.1039/C1AN15537G
(Paper)
Analyst, 2012,
137, 113-117
A glucose/oxygen enzymatic fuel cell based on redox polymer and enzyme immobilisation at highly-ordered macroporous gold electrodes†
Received
28th June 2011
, Accepted 4th October 2011
First published on 21st October 2011
Abstract
Highly ordered macroporous electrodes are prepared by electro-deposition of gold through a polystyrene sphere template. Drop-coating redox polymer and either glucose oxidase, for the anode, or Melanocarpus albomyceslaccase, for the cathode on the macroporous gold provides film-coated electrodes for assembly of membrane-less glucose/oxygen enzymatic fuel cells (EFC) in pH 7.4 buffer containing 10 mM glucose and 0.15 M NaCl. Under these conditions the maximum power density of 17 μWcm−2 for EFCs using films adsorbed to planar gold electrodes increased to 38 μWcm−2 for films adsorbed to 2½ sphere gold macroporous electrodes.
Introduction
Enzymatic fuel cells have the potential to provide power to implanted and portable devices as they are simple in design and easy to miniaturise.1–9 An enzymatic fuel cell can consist of two electrodes containing immobilised enzymes, to specifically oxidize/reduce substrates, obviating the need for membranes or casing/tubing.1,2 A series of reports focused on systematic selection and optimisation of enzyme, poly-N-vinylimidazole (PVI)-bound Os complexes as mediators, and carbon surfaces for anode and cathode, and their combination to provide prototype glucose/oxygen EFCs.1,2,10,11 For example, a glucose/oxygen EFC in 37 °C pH 5 buffer and chloride-free medium used carbon fibre electrodes, modified by cross-linking glucose oxidase (GOx) to [Os(4,4′-dimethyl-2,2′-bipyridine)2(PVI)Cl]+ and a laccase to [Os(4,4′-dimethyl-2,2′-bipyridine)2(2′,6′,2′′-terpyridine)]2+ tethered to a PVI polymer yielded a power density of 137 μWcm−2 at 0.37 V.10 A more flexible tether to PVI improved charge transport for the anode and cathode, providing a power density of 350 μWcm−2 at 0.88 V, again in pH 5 buffer.11 The use of electrodes of high surface area, such as carbon fibres and cloth, in these devices is crucial to achieving the relatively high current and power densities reported. Little attention has been paid to date on how the surface area contributes to delivering such improved signals, apart from excellent recent contributions highlighting the need for normalization of reported figures of merit for EFCs to specific electrode areas/volumes.12,13
The self-assembly of ordered structures onto surfaces can be used as templates for the fabrication of materials with periodic porous structure, with applications in catalysis, separations, scaffolds for tissue engineering and photonics.14–19 Colloidal templates can be used to prepare macroporous electrodes by means of metal electro-deposition from a plating solution around the template, followed by template removal to yield metal scaffolds with interconnected spherical voids.18–22 When redox mediator and enzymes are immobilised within these multilayered structures, current density signals for detection of enzyme substrate20,21 or EFCs22 can be increased compared to that for a planar electrode. A layer-by-layer assembly of gold nanoparticles and NADH-dependent glucose dehydrogenase as anode and laccase as cathode on macroporous gold electrodes yielded a power density of 178 μWcm−2 at 0.23 V in pH 6.0 chloride-free buffer containing 5 mM NADH and 30 mM glucose, almost 16 times larger than an EFC based on a planar electrode.22
The use of oxygen-reducing enzymes, such as bilirubin oxidase (BOx), as cathode component provide for power generating devices more suited to operation in pseudo-physiological conditions. For example, Mano and Heller23 demonstrated that Os-polymer mediated BOx carbon fibre-based cathodes can provide glucose/oxygen EFCs with power density of 244 μWcm−2 at 0.37 V in 15 mM glucose, pH 7.4, PBS. A laccase isolated from Melanocarpus albomyces24 (MaL) can also catalyse oxygen reduction under pseudo-physiological conditions, providing appreciable oxygen-reduction current density when co-immobilised with Os-polymers.25,26
Here we report on glucose/oxygen EFCs based on co-immobilisation of Os-polymers and GOx or MaL on macroporous gold supports. The EFCs do not require addition of NADH co-factor to provide power, and can operate under pseudo-physiological conditions.
Experimental
Materials and reagents
Polystyrene colloidal suspensions (500 nm diameter) were purchased from Duke Scientific Corporation (Brookhaven Instruments Ltd., UK). Gold plating solution (ECF60, 10 g L−1gold content) was from Metalor Technologies Group (Switzerland). Gold substrates were from Evaporated Metal Inc. and were constructed by vapour depositing gold onto titanium coated glass slides. Glucose oxidase from Aspergillus niger was from Sigma-Aldrich (Ireland) and used as received. Purified recombinant Melanocarpus albomyceslaccase was donated by VTT, Finland (K. Kruus). All chemicals were, unless otherwise stated, purchased from Sigma-Aldrich (Ireland) and used as received. All solutions were made from Milli-Q (18.2 MΩ cm) water unless otherwise stated. Synthesis of PVI-bound osmium complexes was carried out according to published procedures.27,28
Apparatus
Scanning Electron Microscopy (SEM) was carried out using an Oxford Instruments S—4700 Scanning Electron Microscope. Cyclic voltammetry (CV) was carried out in a conventional one compartment cell with a CH Instruments 620 potentiostat at ambient temperature (20 ± 2 °C). All solutions were purged with nitrogen for 15 min prior to use. Potentials were measured with respect to a commercial Ag/AgCl (3 M KCl) reference electrode (IJ Cambria, UK) and the counter electrode was a platinum foil (1 cm2, Goodfellow, UK). Assembled membrane-less EFCs were studied with both anode and cathode dipped in a cell containing 200 mL of 0.05 M phosphate buffer, pH 7.4, thermostated at 37 °C, containing glucose at approximate physiological concentrations (10 mM) and 0.15 M of sodium chloride. Both electrodes were externally connected through a resistance box (IET Labs Resistance Decade Box RS-200W) over a resistance range of 5 MΩ to 1 kΩ, and the voltage between the electrodes was measured with a multimeter (Keithley, UK) for each load.
Methods
Surface pre-treatment
Evaporated gold slides used as substrates were washed with ethanol for about 15 min to ensure the removal of any organic impurity on the gold surface. A circular area of the gold substrate, 5 mm diameter, was defined by insulation of the surrounding area with nail varnish. Gold substrates were functionalised by immersing in an ethanolic solution of 10 mM cysteamine hydrochloride for 3 days to allow for a self assembled monolayer to form.
Template assembly by vertical deposition on gold substrates
Polystyrene microsphere suspensions were diluted to the desired concentration with deionised water, and 1 mL of the dilute colloidal suspension was then placed in a polystyrene spectrophotometer cuvette, internal diameter 1 cm. The pre-treated gold substrate was placed along the internal wall of the cuvette vertically in the colloidal suspension. The colloidal suspension was maintained at 55 °C in a temperature controlled bath until it evaporated to dryness, after about 22 h.
Templated electrodeposition
A proprietary gold plating solution (ECF60, Metalor, UK) was purged with nitrogen for 20 min prior to use. The colloidal templates were transferred to a gold plating bath and allowed to soak for 15 min prior to plating at fixed potentials for growth of the gold films around the template. Once electro-deposition was complete, electrodes were transferred into a solution of dimethylformamide (DMF), for 45 min followed by ultrasonication in DMF for a further 10 min to ensure complete dissolution of the polystyrene template.
Planar and macroporous electrodes were modified by drop-coating a solution containing the redox polymer (3 μL of an 8 mg mL−1 solution/suspension in water) and polyoxyethylene bis(glycidyl ether) (6 μL of a 15 mg mL−1 solution in water) as a cross-linker followed by at least 24 h drying of deposited films in a dessicator. For biocatalytic electrodes the enzyme (6 μL of 860 nkat/ml laccase, or 800 nkat/ml glucose oxidase, solutions in water) was also deposited, followed by at least 24 h drying of deposited films in a dessicator.
Results and discussion
The formation of a thiol-based self assembled monolayer on underlying gold substrates has been suggested to increase the efficiency of polystyrene template assembly by increasing substrate–particle interaction.19–21 More recent reports, comparing the use of different thiols for template assembly prior to electrodeposition of gold, attribute the SAM effect to both improvement in surface wettability and the etching of asperities off gold surfaces29 by a “digestive-ripening” effect,30 smoothing the surface and aiding assembly.
Once the initial surface is treated, vertical deposition31 of polystyrene spheres of 500 nm diameter produce well ordered colloidal crystals, seen in the typical SEM image, Fig. 1, for assembled polystyrene spheres on treated gold surfaces. These ordered templates can be utilised to prepare macroporous electrodes by means of electro-deposition of gold from a proprietary plating solution.17–22 A potential for gold electro-deposition of −0.72 V vs.Ag/AgCl, is selected from a cyclic voltammogram (not shown), obtained using a planar gold disc electrode immersed in the plating solution. Inflections observed in the current signal as a function of time during controlled deposition at this potential are indicative of increasing numbers of electroplated layers.21,32,33 Once the electro-deposition process is complete, template removal is achieved by immersion of the samples in a solution of DMF followed by ultra-sonication, to yield macroporous gold structures. Voids and interconnected spaces can be seen through the pores, as shown in the typical SEM image of a 2½ sphere gold structure Fig. 2, demonstrating the production of an interconnected macroporous network.
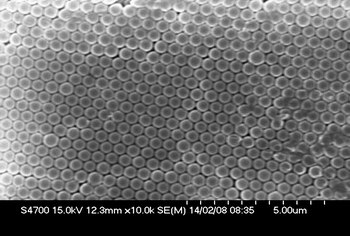 |
| Fig. 1
SEM image of colloidal polystyrene spheres (500 nm diameter) assembled by vertical deposition onto a pre-treated gold substrate in a temperature controlled bath (55 °C). | |
 |
| Fig. 2
SEM images, at different magnification of a macroporous gold scaffold obtained upon electro-depositing though templates formed by vertical deposition of 500 nm diameter polystyrene spheres to yield 2½ deposited layers of gold. | |
Redox
polymer-modified macroporous electrodes
In order to establish if the increased surface area of the macroporous gold is available for adsorption and electrochemical addressing of redox polymer-based mediators, electrodes were modified as per previous protocols for planar electrodes,10,11,25,26 by drop-coating a solution containing a redox polymer, [Os(2,2′-bipyridine)2(PVI)10Cl]+ and a di-epoxide cross-linker, followed by at least 24 h drying of deposited films in a dessicator. Electrodes were subsequently immersed in 0.05 M phosphate buffer, pH 7.4, 0.15 M NaCl, and allowed to equilibrate for 15 min prior to use, to facilitate electrolyte penetration throughout the macroporous networks and hydrogel swelling. Surface concentrations of immobilised Os, calculated from the charge under the Os(II/III) redox transition when the films undergo comprehensive electrolysis at slow scan rates in a cyclic voltammetric experiment (5 mV s−1), of 0.15, 0.39, 0.73 and 1.75 nmol were observed for the planar, ½ sphere, 1½ sphere and 2½ sphere electrodes, respectively. These represent comparable osmium coverages of 0.76, 1.10, 1.09 and 0.98 nmol cm−2 of the estimated electrode area. The surface coverage therefore scales with macroporous area, as observed by others for adsorbed species.19–21,32,33 The slight increase in surface coverage of the macroporous electrodes over that observed for the planar electrode could be due to increased film thickness within the pores, whilst the relatively lower coverage for the 2½ sphere electrodes could be due to blocked pores in the macroporous structure.
Enzymatic fuel cells utilising porous electrodes
The oxygen-reducing MaL enzyme has a T1 copper, substrate-oxidising, potential of 0.27 V vs.Ag/AgCl and a pH optimum of between 6.5 and 7.5.24 Various Os-polymer candidates for oxygen reduction mediation with the MaL have been examined, leading to selection of [Os(2,2′-bipyridine)2(PVI)10Cl]+ (Eo′ = 0.22 V vs.Ag/AgCl) as cathode redox polymer mediator.
Laccase from MaL was incorporated into a hydrogel matrix with [Os(2,2′-bipyridine)2(PVI)10Cl]+ at planar gold electrodes, and macroporous electrodes with a ½, 1½ and 2½ sphere gold layer. These electrodes were immersed in a slowly stirred solution of 0.05 M phosphate buffer, pH 7.4 with 0.15 M NaCl and in the presence of 10 mM glucose, to evaluate the bioelectrocatalytic current signal. Cyclic voltammograms at a scan rate 5 mV s−1 exhibited bioelectrocatalytic steady-state currents for oxygen reduction that increased with increasing sphere layers, Fig. 3.
![Cyclic voltammograms at planar (black), ½ sphere (red), 1 ½ sphere (green) and 2 ½ sphere (blue) gold layer electrodes modified with [Os(2,2′-bipyridine)2(PVI)10Cl]+ and MaL recorded in 0.05 M phosphate buffer, pH 7.4, 0.15 M NaCl, 10 mM glucose. Scan rate 5 mV s−1 and current is normalised to projected geometric area of underlying gold substrate (0.1964 cm2).](/image/article/2012/AN/c1an15537g/c1an15537g-f3.gif) |
| Fig. 3
Cyclic voltammograms at planar (black), ½ sphere (red), 1 ½ sphere (green) and 2 ½ sphere (blue) gold layer electrodes modified with [Os(2,2′-bipyridine)2(PVI)10Cl]+ and MaL recorded in 0.05 M phosphate buffer, pH 7.4, 0.15 M NaCl, 10 mM glucose. Scan rate 5 mV s−1 and current is normalised to projected geometric area of underlying gold substrate (0.1964 cm2). | |
Steady-state oxygen reduction currents increase by 1.8, 3 and 3.8-fold when altering from planar to a ½, 1½ and 2½ sphere gold layer, respectively. Whilst an increase for each layer is observed, the current does not scale directly to the increase in osmium surface coverage of 2.6, 4.8 and 11.7-fold for ½, 1½ and 2½ sphere gold layers, respectively. The current does, however scale, for the ½ and 1½ sphere gold layers, to the estimated increase in surface roughness of 1.8 and 3.4, but not for the 2½ sphere gold layer response. It seems that blockage of access to the pores by the redox polymer/enzyme films becomes an issue as increasing layers of inter-connecting pores of this dimension are assembled.Various Os-polymer candidates can be chosen for the catalytic oxidation of glucose by the enzyme glucose oxidase (GOx). For this study an [Os(4-4′-dimethyl-2,2′-bipyridine)2(PVI)10Cl]+ (Eo′ = 0.1 V vs.Ag/AgCl) was selected as anode redox polymer mediator.10,34Glucose oxidase was incorporated into these hydrogel matrices at planar gold electrodes, and macroporous electrodes with ½, 1½ and 2½ sphere gold layers, and electrodes subsequently immersed in slowly stirred solution of 0.05 M phosphate buffer, pH 7.4 with 0.1 M NaCl and in the presence of 10 mM glucose to evaluate the bioelectrocatalytic current signal. Cyclic voltammograms at a scan rate 5 mV s−1 exhibited bioelectrocatalytic current signals for glucose oxidation that increased with increasing sphere layers, Fig. 4. A relative increase of 1.1, 1.5 and 1.8-fold, over that observed for the planar electrode is obtained for the [Os(4-4′-dimethyl-2,2′-bipyridine)2(PVI)10Cl]+ on ½, 1½ and 2½ sphere gold layer electrodes.
![Cyclic voltammograms at planar (black), ½ sphere (red), 1½ sphere (green) and 2½ sphere (blue) gold layer electrodes modified with [Os(4-4′-dimethyl-2,2′-bipyridine)2(PVI)10Cl]+ and glucose oxidase. Conditions as in Fig. 3.](/image/article/2012/AN/c1an15537g/c1an15537g-f4.gif) |
| Fig. 4
Cyclic voltammograms at planar (black), ½ sphere (red), 1½ sphere (green) and 2½ sphere (blue) gold layer electrodes modified with [Os(4-4′-dimethyl-2,2′-bipyridine)2(PVI)10Cl]+ and glucose oxidase. Conditions as in Fig. 3. | |
Fuel
cells comprising of [Os(2,2′-bipyridine)2(PVI)10Cl]+ and MaL cathode with [Os(4-4′-dimethyl-2,2′-bipyridine)2(PVI)10Cl]+ and GOx anode were assembled in 0.05 M phosphate buffer, containing 10 mM glucose, 0.15 M NaCl, pH 7.4, thermostated to 37 °C and under mild stirring. Electrodes employed consisted of planar gold electrodes, and macroporous electrodes with ½, 1½ and 2½ sphere gold layers electroplated. The maximum power densities for these EFCs increase as a function of increasing sphere layers, Table 1. With a planar system, maximum power densities of 17 ± 10 μW cm−2 are extracted at a cell voltage of 0.25 V, whilst the maximum power density obtained with ½, 1½, and 2½, sphere gold layer electrodes represent relative increases of 1.3, 1.6 and 2.2-fold over that observed for the planar electrode, respectively. The cell voltage at maximum power density remained at ∼ 0.25 V. In our system, the anode was identified as the limiting factor (comparing cyclic voltammograms in Fig. 3 and 4), and the relative increase in power density scales approximately with the increase in current density observed for the anode (Fig. 4).
Table 1 Maximum power densities and cell voltages at that power density obtained using GOx anodes and MaL cathodes immersed in 0.05 M phosphate buffer, containing 10 mM glucose, dissolved O2, 0.15 M NaCl, 37 °C at pH 7.4 (n = 3)
Sphere layer |
Power density/μWcm−2 |
Cell Voltage/V |
0 |
17 (±10) |
0.25 (±0.08) |
½ |
23 (±2) |
0.22 (±0.05) |
1 ½ |
28 (±7) |
0.28 (±0.08) |
2 ½ |
38 (±5) |
0.30 (±0.08) |
This output compares well with the maximum power density of 16 μWcm−2 at a cell voltage of 0.25 V obtained under similar conditions using films of GOx crosslinked to [Os(4,4′-diamino-2,2′-bipyridine)2(PVI)Cl]+ and a fungal laccase crosslinked to [Os(1,10-phenanthroline)2(PVI)2]2+ on graphite electrodes as anode and cathode.36 Improvements have been reportedly achieved by replacement of acidophilic laccase with BOx, more active at neutral pH, and crosslinking with [Os(4,4′-dichloro-2,2′-bipyridine)2(PVI)Cl]+ on carbon fibre microelectrodes to yield a power density of 50 μWcm−2 at 0.5 V.37 Inclusion of flexible tethers between Os redox complexes and PVI polymer improves charge transport within the mediating films, yielding a power density of 430 μWcm−2 at a cell voltage of 0.52 V for a carbon-fiber based GOx/BOx membraneless fuel cell.38
Conclusions
A glucose/oxygen enzymatic fuel cell was assembled from macroporous electrodes, prepared by electro-deposition of gold through a polystyrene sphere template. Use of [Os(2,2′-bipyridine)2(PVI)10Cl]+ and MaL cathode with [Os(4-4′-dimethyl-2,2′-bipyridine)2(PVI)10Cl]+ and GOx anode permitted EFC operation under pseudo-physiological conditions, providing maximum power density of 17 μWcm−2 for EFCs using films adsorbed to planar gold electrodes, that increased to 38 μWcm−2 for films adsorbed to 2½ sphere gold macroporous electrodes, with the anode identified as being the limiting factor. Increased power densities may be achievable using improved anodes, based on redox polymers that display improved redox potentials or charge transport rates and/or improved mass transport to and within the gold macroporous electrodes.
Acknowledgements
VTT Finland is gratefully acknowledged for donation of MaL. Helpful discussions with P.N. Bartlett are acknowledged. Funding for this research was provided by a Charles Parsons Energy Research Award, through Science Foundation Ireland, and by an EU FP6 NMP4-ct-2006-17350 ‘Biomednano’ award.
Notes and references
- A. Heller, Phys. Chem. Chem. Phys., 2004, 3, 209 RSC.
- A. Heller, AIChE J., 2005, 51, 1054 CrossRef CAS.
- S. C. Barton, J. Gallaway and P. Atanassov, Chem. Rev., 2004, 104, 4867 CrossRef CAS.
- F. Davis and S. P. J. Higson, Biosens. Bioelectron., 2007, 22, 1224 CrossRef CAS.
- S. D. Minteer, B. Y. Liaw and M. J. Cooney, Curr. Opin. Biotechnol., 2007, 18, 228 CrossRef CAS.
- J. A. Cracknell, K. A. Vincent and F. A. Armstrong, Chem. Rev., 2008, 108, 2439 CrossRef CAS.
- M. J. Cooney, V. Svoboda, C. Lau, G. Martin and S. D. Minteer, Energy Environ. Sci., 2008, 1, 320 CAS.
- I. Willner, Y. M. Yan, B. Willner and R. Tel-Vered, Fuel Cells, 2009, 9, 7 CrossRef CAS.
- M. H. Osman, A. A. Shah and F. C. Walsh, Biosens. Bioelectron., 2011, 26, 3087 CrossRef CAS.
- T. Chen, S. Calabrese Barton, G. Binyamin, Z. Gao, Y. Zhang, H. H. Kim and A. Heller, J. Am. Chem. Soc., 2001, 123, 8630 CrossRef CAS.
- V. Soukharev, N. Mano and A. Heller, J. Am. Chem. Soc., 2004, 126, 8368 CrossRef CAS.
- S. Rubenwolf, O. Strohmeier, A. Kloke, S. Kerzenmacher, R. Zengerle and F. von Stetten, Biosens. Bioelectron., 2010, 26, 841 CrossRef CAS.
- V. Svoboda, M. Cooney, B. Y. Liaw, S. Minteer, E. Piles, D. Lehnert, S. Calabrese Barton, R. Rincon and P. Atanassov, Electroanalysis, 2008, 20, 1099 CrossRef CAS.
- H. Yan, C. F. Blanford, B. T. Holland, W. H. Smyrl and A. Stein, Chem. Mater., 2000, 12, 1134 CrossRef CAS.
- J. E. G. J. Wijnhoven, S. J. M. Zevenhuizen, M. A. Hendriks, D. Vanmaekelbergh, J. J. Kelly and W. L. Vos, Adv. Mater., 2000, 12, 888 CrossRef CAS.
- L. Xu, W. L. Zhou, C. Frommen, R. H. Baughman, A. A. Zakhidov, L. Malkinski, J. Q. Wang and J. B. Wiley, Chem. Commun., 2000, 997 RSC.
- P. Bartlett, P. Birkin and M. Ghanem, Chem. Commun., 2000, 1671 RSC.
- P. Jiang, G. N. Ostojic, R. Narat, D. M. Mittleman and V. L. Colvin, Adv. Mater., 2001, 13, 389 CrossRef CAS.
- S. Ben-Ali, D. A. Cook, S. A. G. Evans, A. Thienpont, P. N. Bartlett and A. Kuhn, Electrochem. Commun., 2003, 5, 747 CrossRef CAS.
- S. Ben-Ali, D. A. Cook, P. N. Bartlett and A. Kuhn, J. Electroanal. Chem., 2005, 579, 181 CrossRef CAS.
- R. Szamocki, A. Velichko, F. Mucklich, S. Reculusa, S. Ravaine, S. Neugebauer, W. Schuhmann, R. Hempelmann and A. Kuhn, Electrochem. Commun., 2007, 9, 2121 CrossRef CAS.
- L. Deng, F. Wang, H. Chen, L. Shang, L. Wang, T. Wang and S. Dong, Biosens. Bioelectron., 2008, 24, 329 CrossRef CAS.
- N. Mano and A. Heller, J. Electrochem. Soc., 2003, 150, A1136 CrossRef CAS.
- L.-L. Kiiskinen, L. Viikari and K. Kruus, Appl. Microbiol. Biotechnol., 2002, 59, 198 CrossRef CAS.
- P. Kavanagh, P. Jenkins and D. Leech, Electrochem. Commun., 2008, 10, 970 CrossRef CAS.
- P. Kavanagh, S. Boland, P. Jenkins and D. Leech, Fuel Cells, 2009, 9, 79 CrossRef CAS.
- R. J. Forster and J. G. Vos, Macromolecules, 1990, 23, 4372 CrossRef CAS.
- E. M. Kober, J. V. Caspar, B. P. Sullivan and T. J. Meyer, Inorg. Chem., 1988, 27, 4587 CrossRef CAS.
-
S. Mahajan, PhD Thesis, University of Southampton, 2008.
- B. L. V. Prasad, S. I. Stoeva, C. M. Sorensen and K. J. Klabunde, Chem. Mater., 2003, 15, 935 CrossRef CAS.
- P. Jiang, J. F. Bertone, K. S. Hwang and V. L. Colvin, Chem. Mater., 1999, 11, 2132 CrossRef CAS.
- R. Szamocki, S. Reculusa, S. Ravaine, P. N. Bartlett, A. Kuhn and R. Hempelmann, Angew. Chem., Int. Ed., 2006, 45, 1317 CrossRef CAS.
- R. Szamocki, A. Velichko, C. Holzapfel, F. Mucklich, S. Ravaine, P. Garrigue, N. Sojic, R. Hempelmann and A. Kuhn, Anal. Chem., 2007, 79, 533 CrossRef CAS.
- T. Delumley Woodyear, P. Rocca, J. Lindsay, Y. Dror, A. Freeman and A. Heller, Anal. Chem., 1995, 67, 1332 CrossRef CAS.
- P. A. Jenkins, S. Boland, P. Kavanagh and D. Leech, Bioelectrochemistry, 2009, 76, 162 CrossRef CAS.
- F. Barrière, P. Kavanagh and D. Leech, Electrochim. Acta, 2006, 51, 5187 CrossRef.
- H. H. Kim, N. Mano, Y. Zhang and A. Heller, J. Electrochem. Soc., 2003, 150, A209 CrossRef CAS.
- N. Mano, F. Mao and A. Heller, J. Am. Chem. Soc., 2002, 124, 12962 CrossRef CAS.
Footnote |
† This article is part of a web theme in Analyst and Analytical Methods on Future Electroanalytical Developments, highlighting important developments and novel applications. Also in this theme is work presented at the Eirelec 2011 meeting, dedicated to Professor Malcolm Smyth on the occasion of his 60th birthday. |
|
This journal is © The Royal Society of Chemistry 2012 |