DOI:
10.1039/D3NA00926B
(Paper)
Nanoscale Adv., 2024,
6, 985-989
First-principles calculations of inorganic metallocene nanowires†
Received
26th October 2023
, Accepted 29th December 2023
First published on 22nd January 2024
Abstract
Inspired by the recently synthesized inorganic metallocene derivatives Fe(P4)22−, we have identified four stable inorganic metallocene nanowires, MP4 (M = Sc, Ti, Cr and Fe) in configurations of either regular quadrangular prism (Q-type) or anticube (A-type), and further investigated their magnetic and electronic characteristics utilizing the first-principles calculation. It shows that CrP4 is a ferromagnetic metal, while other nanowires are semiconducting antiferromagnets with bandgaps of 0.44, 1.88, and 2.29 eV within the HSE06 level. It also shows that both ScP4 and TiP4 can be stabilized in the Q-type and A-type, corresponding to the antiferromagnetic and ferromagnetic ground states, respectively, indicating a configuration-dependent magnetism. The thermodynamic and lattice stabilities are confirmed by the ab initio molecular dynamics and phonon spectra. This study has unmasked the structural and physical properties of novel inorganic metallocene nanowires, and revealed their potential application in spintronics.
Introduction
Since the milestone discovery of ferrocene,1 the multidecker molecular complexes in the form of sandwich clusters and their infinitely extended nanowires have attracted great research interest due to the distinct structural, optical,2,3 chemical,4 electrical,5 and magnetic properties,6 rendering them potential candidates in catalysis,7 electronics and spintronics.8–12 Especially, the tunable coordination mode between the central metal and organic molecules accompanied by diverse charge transfer and crystal field splitting can lead to unpaired d electrons and form intriguing magnetic ordering phenomena.13–15 For instance, the organometallic complexes composed of vanadium and benzene, Vn(Bz)m (Bz = C6H6), were confirmed to be molecular magnets and the magnetic moments of the complexes increase with the central metal atoms.16,17 Then, theoretical study revealed that the one-dimensional [V(Bz)]∞ and [Mn(Bz)]∞ are quasi- or half-metallic.18 Also, (VCp)∞ (Cp = C5H5), (VBzVCp)∞, and (V2Ant)∞ wires (Ant = anthracene) are ferromagnetic half-metals.19 Based on pentalene (Pn = C8H6), double metallocene nanowires were predicted, in which the PnMn2 nanowire exhibits ferromagnetic behavior.20 Among the homo-bimetallic naphthalene (Np
C10H8), namely NpTM2, (TM = V, Mn, Ti, Nb) nanowires, NpMn2 is ferromagnetic, NpTi2 and NpNb2 are antiferromagnetic, and NpV2 exhibits carrier-tunable magnetic ordering.21 Then, the hetero-bimetallic naphthalene nanowires, [Np2V2Cr2]∞, [Np2V2Mn2]∞, and [Np2V2Fe2]∞ are shown as half-metals, while [Np2V2Cr2]∞ is quasi-half-metallic,22 and the Vn−1Npn nanowire also exhibits carrier-tunable magnetic ordering.23 Theoretical studies also demonstrated that the sandwiched EunCOTn+1 (Eu = europium, COT = C8H8, n = 1–4) clusters are molecular magnets.24 By using the chemical functionalization, the multiferroic organometallic molecular nanowires were predicted.25 A recent study showed that ferromagnetic half-metallicity can be realized in metal trihydride molecular nanowires.15 As a result, exploring novel metallocene-analogue molecular clusters and nanowires with magnetism is highly desirable due to their high potential application as one-dimensional spintronics.
To enrich the family of multidecker sandwich complexes, great efforts have been made to explore molecules similar to ferrocene and it is assumed that a heteroelement derivative can be obtained by the isolobal replacement of CH with group 15 phosphorus, which may possess distinct properties with various bond types and coordination environment.26 Despite the high stability of Fe(η5-P5)2 confirmed by the theoretical calculation,27 experimental realization has not been achieved, and only a molecular complex Ti(η5-P5)2 was reported to be synthesized so far.28 Cyclo-P4, which can accommodate two electrons, is believed to be more useful in contrast to the monoanionic cyclo-P5.29 Very recently, Wang et al. has synthesized a ferrocene derivative [Fe(P4)2]2− by using Zintl ions as precursors,30 which may serve as the building block for the inorganic metallocene complexes.
Enlighted by the recently synthesized Fe(P4)22−, inorganic metallocene nanowires, MP4 (M = Sc, Ti, Cr and Fe) are reported and the structural, electrical, and magnetic characteristics are investigated within the first-principles calculation. Our results show that ScP4, TiP4, CrP4 and FeP4 can form stable one-dimensional nanowires in either regular quadrangular prism (Q-type) or anticube (A-type) configurations. Among them, ScP4, TiP4 and FeP4 nanowires have antiferromagnetic (AFM) ground state and CrP4 nanowires are ferromagnetic (FM). AIMD simulation and phonon spectrum calculations have confirmed their thermodynamic and lattice stabilities.
Methods
First-principles calculations were performed by using the Perdew–Burke–Ernzerhof (PBE) functional and Projector Augmented Wave (PAW) potential within the Vienna ab initio simulation (VASP) package.31–35 A cut-off energy of 400 eV was adopted, and the atomic positions and lattice constant along the extended direction were relaxed until the force and energy were smaller than 0.01 eV Å−1 and 1 × 10−5 eV. A Γ-centered 1 × 1 × 8 k-points was used. According to the previous research,36,37 a Hubbard Ueff value of 3 eV was adopted to account for the strong correlation effect. To obtain a more accurate electronic structure, the Heyd–Scuseria–Ernzerhof hybrid functional (HSE06) was adopted.38 One-dimensional chains were chosen to extend along the c-axis, while a certain vacuum layer thickness (around 10 Å) is added in the other directions of the unit cell to avoid repetitive disturbances of the force field under the periodic boundary conditions. Considering the van der Waals (vdW) interactions, the Grimme DFT-D3 correction was utilized.39 Additionally, ab initio molecular dynamics (AIMD) simulations were executed with the supercell of 1 × 1 × 4 and the total time was 5 ps with each step of 1 fs, using the NVT ensemble. The phonon spectra were calculated with density-functional-perturbation theory (DFPT) using PHONOPY.40 The post-processing calculations for the relevant calculations were performed using the VESTA software41 and VASPKIT code.42
Results and discussion
According to Fig. 1(a), the MP4 molecular complex is composed of one transition metal atom sandwiched between two square cyclo-P4 rings, owning to the C4v symmetry and forming an η4
:
η4 coordination pattern. The P4 rings can arrange into two configurations, namely, regular quadrangular prism (Q-type) (Fig. 1(b)) or anticube (A-type) (Fig. 1(c)). Hence, two types of MP4 nanowires are considered, as shown in Fig. 1(d) and (e), respectively. Then, the geometrical configurations of MP4 nanowires (M = Sc to Ni) in Q-type and A-type are relaxed and it shows that ScP4, CrP4, and FeP4 are energetically more stable in the Q-type and TiP4 is energetically more stable in the A-type, as displayed in Table S1.† Cu and Zn are excluded due to their absence of metallocene forms. The lattice stability of the energetically stable MP4 is identified according to their phonon spectra. As displayed in Fig. S1,† imaginary frequencies are absent for the ScP4, CrP4, and FeP4, and a small imaginary frequency in TiP4 nanowires is partly attributed to the flat potential energy surface at its ground state. Hence, the calculations below are based on the ScP4, TiP4, CrP4 and FeP4 nanowires.
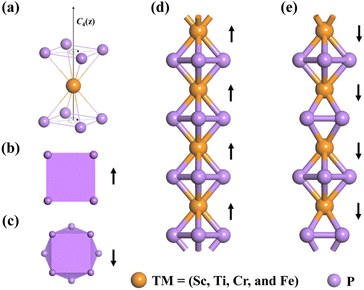 |
| Fig. 1 Models of (a) MP4 molecular complex, (b) regular quadrangular prism (Q-type) ligand field, (c) anticube (A-type) ligand field, (d) Q-type MP4 nanowire, and (e) A-type MP4 nanowire. The “up” and “down” arrows indicate the different stacking models of cyclo-P4 rings. | |
As demonstrated in Table 1, the M–P distances exhibit a range of 2.46 to 2.70 Å, descending from Sc to Fe, and the P–P distances among adjacent P atoms vary from 2.24 to 2.28 Å, indicating the elongated P–P distance compared to black phosphorus (2.18 Å).43 To further determine their stability, we calculated the averaged formation energy per unit cell, which is described as below, in which ENW[MP4], E[M], and E[P4] are the energies of the nanowire, single metal atom, and cyclo-P4 ring, respectively.
Ef[MP4] = ENW[MP4] − E[M] − E[P4] |
Table 1 Metal–phosphorus bond distance (dMP, Å), phosphorus–phosphorus bond distance (dPP, Å), averaged formation energy per unit cell (Ef, eV), transferred charge from the metal to phosphorus (C, e), and atomic magnetic moment (M, μB) on each metal atom, ground state (GS, in which S and M denote the semiconductor and metal), and energy difference (ΔE = E(FM) − E(AFM), eV) between ferromagnetic (FM) and antiferromagnetic (AFM) states per unit cell
|
ScP4 |
TiP4 |
CrP4 |
FeP4 |
d
MP
|
2.70 |
2.55 |
2.52 |
2.46 |
d
PP
|
2.26 |
2.28 |
2.24 |
2.24 |
E
f
|
−8.00 |
−7.99 |
−5.79 |
−6.43 |
C
|
1.67 |
1.55 |
1.01 |
0.76 |
M
|
0.16 |
0.80 |
3.31 |
3.21 |
GS |
AFM S |
AFM S |
FM M |
AFM S |
ΔE |
0.02 |
0.68 |
−0.58 |
0.66 |
The calculated formation energies vary from −5.79 to −8.00 eV, in which the negative formation energies indicate that the formation of MP4 nanowires from the P4 ring and metal atom is exothermic, and ScP4 nanowire has the largest formation energy. These values are larger or comparable to those for other organic multidecker nanowires, including transition metal benzene nanowires (−1.71 to −5.33 eV) or metallocene nanowires.18,44
In addition, we also examined the thermodynamic stability of the MP4 nanowires by conducting the ab initio molecular dynamics (AIMD) simulation at temperatures increasing up to 700 K. The evolution of total energy and magnetic moments along with time, and the last snapshots for the AIMD simulations are shown in Fig. S4.† It can be observed that the structural motif A-type TiP4 nanowires can be maintained at 300 K. CrP4 nanowires are able to maintain their structure at 400 K, FeP4 at 500 K, and for ScP4, the temperature can reach up to 700 K. The difference in thermodynamic stability can be elucidated by the energy difference between the A-type and the Q-type structures in the same magnetic ground state per unit cell, as shown in Table S1.† The energy difference between the Q-type and A-type CrP4 nanowires in the FM state is the smallest (0.196 eV), and ScP4 has the largest energy difference (0.759 eV), leading to different thermodynamic stabilities. Meanwhile, the poor thermodynamic stability of TiP4 is partly attributed to the low oxidation state of central Ti.
Next, the magnetic ground states of the MP4 nanowires are confirmed and three magnetic states are considered, ferromagnetic (FM), antiferromagnetic (AFM) states, and nonmagnetic states (NM). The ScP4, TiP4 and FeP4 nanowires are identified to be antiferromagnetic, in which the energy differences are 0.02 eV, 0.68 eV and 0.66 eV between the FM and AFM states. Particularly, the CrP4 nanowires are ferromagnetic in their ground state, which is 0.58 eV energetically lower than the AFM state. The atomic magnetic moments of Sc, Ti, Cr, and Fe atoms are 0.16, 0.80, 3.31, and 3.21 μB, respectively. We have provided the element-resolved density of states and the spatial distribution of CBM/VBM decomposed charge density in the real space for the three semiconducting nanowires at the same isosurface level in Fig. S5 and S9.† It shows that for ScP4 (Fig. S9(a and b)†) the VBM and CBM are contributed by dxy/dx2−y2, for TiP4 (Fig. S9(c and d)†) the VBM and CBM are contributed by dxy/dx2−y2 and dz2, respectively, for FeP4 (Fig. S9(e and f)†) the VBM and CBM are contributed by dxz/dyz and dz2, respectively, which is well-accorded with the calculated electronic structures in Fig. 2 and S6.†
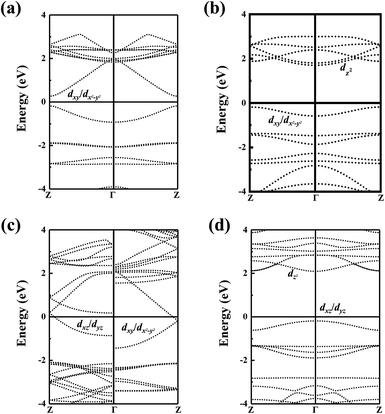 |
| Fig. 2 The electronic band structures for (a) ScP4, (b) TiP4, (c) CrP4, and (d) FeP4 nanowires at the HSE06 level. | |
Then, the band structures of the MP4 nanowires are illustrated in Fig. 2. ScP4 and FeP4 nanowires have direct band gaps with bandgaps of 0.44 eV and 2.29 eV, and the TiP4 nanowires have an indirect bandgap with bandgaps of 1.88 eV at the HSE06 level, respectively. Meanwhile, CrP4 nanowires are metallic.
Next, we have tried to understand the origin of magnetism of the MP4 nanowires. In a regular quadrangular prism crystal field, five 3d orbitals of metal atoms are readily split into a doubly degenerate band e (dxz, dyz), and three singly degenerate bands a1, a2, a3 (dxy, dx2−y2, and dz2) for the MP4 nanowire except for CrP4. For CrP4, the residual unpaired electrons can fill one of the doubly degenerate orbitals and the other is empty, resulting in five single d orbitals. In anticube configurations, five 3d orbitals of metal atoms can be split into doubly degenerate bands e1(dxy, dx2−y2), e2(dxz, dyz), and a singly degenerate band a(dz2). Their density of states is shown in Fig. S5.† It also shows that the direction of electron transfer is from the metal atom to the P4 ring using the Bader charge analysis (Table 1), and residual unpaired d electrons account for the existence of magnetism.
Furthermore, we have plotted the electronic configuration of metal atoms to understand the diverse magnetic ground states in CrP4 and FeP4 nanowires. Based on the crystal field splitting theory and Bader charge analysis, the electronic configuration of metal ions in CrP4 and FeP4 nanowires are shown in Fig. S10.† As shown, both Cr and Fe ions have half-filled orbitals and the cation–anion–cation bond angle is 180°. According to the super exchange interaction theory,45 two magnetic ions with half-occupied orbitals coupled through an intermediary non-magnetic ion lead to a strong anti-ferromagnetic coupling, which confirmed the antiferromagnetic coupling in FeP4 nanowires (Fig. S10(a)†). However, for CrP4, the P4 ring is slightly magnetized according to the spin charge density (Fig. S8(c)†), leading to the spin exchange pathway in Fig. S10(b)† and ferromagnetic coupling between Cr ions.
Considering the rotation of P4 rings, we have also investigated the meta-stable phase of MP4 nanowires and it shows that ScP4 and TiP4 can be stabilized in both regular quadrangular prism (Q-type) and anticube (A-type) configurations (Fig. S3†), corresponding to AFM and FM ground states, respectively, indicating a novel configuration-dependent magnetism. According to their electronic band structures (Fig. S7†), ScP4 is a BMS (Bipolar Magnetic Semiconductor)46 material of A-type and TiP4 is a ferromagnetic metal of Q-type, showing a configuration tunable magnetism.
Based on the Mermin–Wagner theorem,47 long-range magnetic order is absent for any infinitely strict one-dimensional isotropic system at non-zero temperature. However, the relatively large magnetic crystalline anisotropic energy can help to hinder thermal fluctuation and stabilize the long-range ordering in one-dimensional or quasi-one-dimensional systems.48 Then, non-collinear calculations are conducted and the results in Table S2† show that easy-axis of MP4 (M = Sc, Ti, Cr, Fe) is normal to the nanowires and the magnetic anisotropic energy values are 0.18, 13.58, 77.86, and 326.18 μeV per atom. TiP4, CrP4 and FeP4 are higher or comparable to the bulk b.c.c.Fe (−1.4 μeV per atom), bulk h.c.p.Co (−65 μeV per atom), bulk f.c.c.Co (1.8 μeV per atom), and bulk f.c.c.Ni (2.7 μeV per atom),49,50 suggesting the possibility of maintaining the long-range magnetic ordering. Also, the long-range magnetic order can be stabilized by the interchain coupling of MP4 nanowires. Then, we calculated the inter-chain coupling between two CrP4 nanowires with an optimized distance of about 3.8 Å (Fig. S11†). It was found that the inter-chain magnetic coupling is FM, which is 0.72 meV M−1 lower than AFM coupling in energy with a J value of 0.26 meV. This value is relatively larger than that of p-nitrophenyl nitrooxide (p-NPNN), which exhibits a Curie temperature of 0.65 K and the interchain coupling J is estimated to be about 0.1 K (0.009 meV).51
Conclusions
In summary, this work reports four types of MP4 inorganic metallocene nanowires (M = Sc, Cr, Ti, Fe) that exhibit different electronic and magnetic properties. Our calculations show that MP4 clusters can form stable one-dimensional nanowires. ScP4, TiP4 and FeP4 nanowires are AFM semiconductors, while CrP4 is an FM metal in the ground state. Phonon spectrum calculations and AIMD simulations demonstrate the lattice and thermodynamic stability of these nanowires. The formation of MP4 nanowires via assembly of the P4 ring and metal atoms is exothermic. Both ScP4 and TiP4 can be stabilized in the Q-type and A-type, corresponding to AFM and FM ground states, respectively, suggesting a novel configuration-dependent magnetism. These results reveal the potential application of MP4 nanowires in spintronics. This work can promote the development of one-dimensional spintronics based on the inorganic molecular complexes.
Author contributions
X. W. and H. L. designed the project. Y. J. and H. L. performed the calculation and analysed the data. Y. J., H. L., and X. W. wrote the manuscript.
Conflicts of interest
There are no conflicts to declare.
Acknowledgements
The authors gratefully acknowledge the financial support from the National Natural Science Foundation for Distinguished Young Scholars (Grant 22225301), the National Natural Science Foundation of China (Grants 22303092, 22321001), the Strategic Priority Research Program of the Chinese Academy of Sciences (Grant XDB0450101), and the support from the Super Computer Center of USTCSCC and SCCAS.
References
- T. J. Kealy and P. L. Pauson, Nature, 1951, 168, 1039–1040 CrossRef CAS.
- S.-J. Wang, Y.-F. Wang and C. Cai, J. Phys. Chem. C, 2015, 119, 16256–16262 CrossRef CAS.
- S.-J. Wang, Y. Li, D. Wu, Y.-F. Wang and Z.-R. Li, J. Phys. Chem. A, 2012, 116, 9189–9196 CrossRef CAS PubMed.
- X. Liu, Y. Tan, Z. Ma and Y. Pei, J. Phys. Chem. C, 2016, 120, 27980–27988 CrossRef CAS.
- N. Hosoya, R. Takegami, J.-I. Suzumura, K. Yada, K. Miyajima, M. Mitsui, M. B. Knickelbein, S. Yabushita and A. Nakajima, J. Phys. Chem. A, 2014, 118, 8298–8308 CrossRef CAS PubMed.
- T. Xie and W. Qin, J. Phys. Chem. C, 2016, 120, 24498–24502 CrossRef CAS.
- L. Münzfeld, S. Gillhuber, A. Hauser, S. Lebedkin, P. Hädinger, N. D. Knöfel, C. Zovko, M. T. Gamer, F. Weigend, M. M. Kappes and P. W. Roesky, Nature, 2023, 620, 92–96 CrossRef PubMed.
- J. L. Wang, X. Y. Zhang, P. V. R. Schleyer and Z. F. Chen, J. Chem. Phys., 2008, 128, 104706 CrossRef PubMed.
- Y. X. Wang, C. C. Sun, Z. D. Yang, H. Yu, P. Pan, L. Pei, G. L. Zhang and Y. Y. Hu, Chem. Phys., 2019, 523, 1–6 CrossRef CAS.
- X. Liu, Y. Tan, G. Zhang and Y. Pei, J. Phys. Chem. C, 2018, 122, 16168–16177 CrossRef CAS.
- Z. Yang, B. L. Zhang, X. G. Liu, Y. Z. Yang, X. Y. Li, S. J. Xiong and B. S. Xu, Org. Electron., 2013, 14, 2916–2924 CrossRef CAS.
- P. Gain, R. Jana and A. Datta, J. Phys. Chem. A, 2021, 125, 3362–3368 CrossRef CAS PubMed.
- L. Zhou, S.-W. Yang, M.-F. Ng, M. B. Sullivan, V. B. C. Tan and L. Shen, J. Am. Chem. Soc., 2008, 130, 4023–4027 CrossRef CAS PubMed.
- L. Wang, X. Gao, X. Yan, J. Zhou, Z. Gao, S. Nagase, S. Sanvito, Y. Maeda, T. Akasaka, W. N. Mei and J. Lu, J. Phys. Chem. C, 2010, 114, 21893–21899 CrossRef CAS.
- X. Li, H. Lv, J. Dai, L. Ma, X. C. Zeng, X. Wu and J. Yang, J. Am. Chem. Soc., 2017, 139, 6290–6293 CrossRef CAS PubMed.
- K. Miyajima, A. Nakajima, S. Yabushita, M. B. Knickelbein and K. Kaya, J. Am. Chem. Soc., 2004, 126, 13202–13203 CrossRef CAS PubMed.
- J. Wang, P. H. Acioli and J. Jellinek, J. Am. Chem. Soc., 2005, 127, 2812–2813 CrossRef CAS PubMed.
- H. Xiang, J. Yang, J. G. Hou and Q. Zhu, J. Am. Chem. Soc., 2006, 128, 2310–2314 CrossRef CAS PubMed.
- L. Wang, Z. Cai, J. Wang, J. Lu, G. Luo, L. Lai, J. Zhou, R. Qin, Z. Gao, D. Yu, G. Li, W. N. Mei and S. Sanvito, Nano Lett., 2008, 8, 3640–3644 CrossRef CAS PubMed.
- X. Wu and X. C. Zeng, J. Am. Chem. Soc., 2009, 131, 14246–14248 CrossRef CAS PubMed.
- Z. Zhang, X. Wu, W. Guo and X. C. Zeng, J. Am. Chem. Soc., 2010, 132, 10215–10217 CrossRef CAS PubMed.
- X. Zhang, J. Han, Y. Liu and J. Wang, J. Phys. Chem. C, 2012, 116, 5414–5419 CrossRef CAS.
- Y. Li, Z. Zhou and Z. Chen, J. Phys. Chem. A, 2012, 116, 1648–1654 CrossRef CAS PubMed.
- X. Zhang, M.-F. Ng, Y. Wang, J. Wang and S.-W. Yang, ACS Nano, 2009, 3, 2515–2522 CrossRef CAS PubMed.
- M. Wu, J. D. Burton, E. Y. Tsymbal, X. C. Zeng and P. Jena, J. Am. Chem. Soc., 2012, 134, 14423–14429 CrossRef CAS PubMed.
- N. Kuhn, E.-M. Horn, R. Boese and N. Augart, Angew Chem. Int. Ed. Engl., 1988, 27, 1368–1369 CrossRef.
- G. Frenking, K. Wichmann, N. Fröhlich, C. Loschen, M. Lein, J. Frunzke and V. M. Rayón, Coord. Chem. Rev., 2003, 238, 55–82 CrossRef.
- E. Urnezius, W. W. Brennessel, C. J. Cramer, J. E. Ellis and P. V. Schleyer, Science, 2002, 295, 832–834 CrossRef CAS PubMed.
- J. Trygg, B. Johansson, O. Eriksson and J. M. Wills, Phys. Rev. Lett., 1995, 75, 2871–2874 CrossRef CAS PubMed.
- Z.-C. Wang, L. Qiao, Z.-M. Sun and M. Scheer, J. Am. Chem. Soc., 2022, 144, 6698–6702 CrossRef CAS PubMed.
- G. Kresse and J. Furthmuller, Phys. Rev. B: Condens. Matter Mater. Phys., 1996, 54, 11169–11186 CrossRef CAS PubMed.
- G. Kresse and J. Furthmuller, Comput. Mater. Sci., 1996, 6, 15–50 CrossRef CAS.
- J. P. Perdew, K. Burke and M. Ernzerhof, Phys. Rev. Lett., 1996, 77, 3865–3868 CrossRef CAS PubMed.
- P. E. Blöchl, Phys. Rev. B: Condens. Matter Mater. Phys., 1994, 50, 17953–17979 CrossRef PubMed.
- G. Kresse and D. Joubert, Phys. Rev. B: Condens. Matter Mater. Phys., 1999, 59, 1758–1775 CrossRef CAS.
- Y. G. Zhang, Z. Cui, B. S. Sa, N. H. Miao, J. Zhou and Z. M. Sun, Nanoscale Horiz., 2022, 7, 276–287 RSC.
- S. Y. Wang, N. X. Miao, K. H. Su, V. A. Blatov and J. J. Wang, Nanoscale, 2021, 13, 8254–8263 RSC.
- J. Heyd, G. E. Scuseria and M. Ernzerhof, J. Chem. Phys., 2006, 124, 219906 CrossRef.
- S. Grimme, J. Comput. Chem., 2006, 27, 1787–1799 CrossRef CAS PubMed.
- A. Togo and I. Tanaka, Scr. Mater., 2015, 108, 1–5 CrossRef CAS.
- K. Momma and F. Izumi, J. Appl. Crystallogr., 2011, 44, 1272–1276 CrossRef CAS.
- V. Wang, N. Xu, J. C. Liu, G. Tang and W. T. Geng, Comput. Phys. Commun., 2021, 267, 108033 CrossRef CAS.
- P. Chen, N. Li, X. Chen, W.-J. Ong and X. Zhao, 2D Mater., 2017, 5, 014002 CrossRef.
- L. Shen, S. W. Yang, M. F. Ng, V. Ligatchev, L. P. Zhou and Y. P. Feng, J. Am. Chem. Soc., 2008, 130, 13956–13960 CrossRef CAS PubMed.
-
J. B. Goodenough, Magnetism and the Chemical Bond, Wiley-Interscience, New-York, 1963 Search PubMed.
- H. D. Wang, Q. Q. Feng, X. X. Li and J. L. Yang, Research, 2022, 9857631 CAS.
- N. D. Mermin and H. Wagner, Phys. Rev. Lett., 1966, 17, 1133–1136 CrossRef CAS.
- P. Gambardella, A. Dallmeyer, K. Maiti, M. C. Malagoli, W. Eberhardt, K. Kern and C. Carbone, Nature, 2002, 416, 301–304 CrossRef CAS PubMed.
- A. N. Anisimov, M. Farle, P. Poulopoulos, W. Platow, K. Baberschke, P. Isberg, R. Wäppling, A. M. N. Niklasson and O. Eriksson, Phys. Rev. Lett., 1999, 82, 2390–2393 CrossRef CAS.
- J. Trygg, B. Johansson, O. Eriksson and J. M. Wills, Phys. Rev. Lett., 1995, 75, 2871–2874 CrossRef CAS.
- M. Takahashi, P. Turek, Y. Nakazawa, M. Tamura, K. Nozawa, D. Shiomi, M. Ishikawa and M. Kinoshita, Phys. Rev. Lett., 1991, 67, 746–748 CrossRef CAS.
Footnote |
† Electronic supplementary information (ESI) available: Phonon spectrum calculations; AIMD results; density of states; energy differences between the A-type and Q-type nanowires; magnetic anisotropy energy for the nanowires. See DOI: https://doi.org/10.1039/d3na00926b |
|
This journal is © The Royal Society of Chemistry 2024 |
Click here to see how this site uses Cookies. View our privacy policy here.