DOI:
10.1039/C9RA00797K
(Paper)
RSC Adv., 2019,
9, 8529-8536
A highly selective colorimetric fluorescent probe for detection of Hg2+ and its application on test strips
Received
30th January 2019
, Accepted 2nd March 2019
First published on 13th March 2019
Abstract
An efficient fluorescent probe Pyr-Rhy based on pyrazole was developed, which can detect Hg2+ in water. Its fluorescence properties were studied by UV-vis and fluorescence spectroscopy, and the study results indicated that this probe can selectively detect Hg2+via complexation reaction, and then cause a remarkable color change from colorless to pink and a strong fluorescence enhancement can be observed. Furthermore, this probe showed high sensitivity with the detection limit down to 2.07 × 10−8 M, and its stoichiometric ratio toward Hg2+ ions was 1
:
1. The sensing mechanism was investigated by Job's plot 1H NMR titrations, and FT-IR spectra analysis, which demonstrated a chelation-enhanced fluorescence (CHEF) mechanism. More importantly, obvious color changes of sensor Pyr-Rhy can be observed when it was impregnated on filter paper testing strips and immersed in Hg2+ solution (water as solution), indicating its potential application for trace Hg2+ detection in environmental samples.
1. Introduction
Mercury (Hg), one of the most toxic pollutants,1 is widely distributed in the environment and exists in the state of mercury metal, inorganic mercury and organic mercury compounds.2,3 The organic mercury is the most harmful of the mercury-containing compounds, because it is hardly biodegradable and can be biomagnified in the food chain.4 Even at very low levels, organic mercury can seriously destroy the activity of proteins and enzymes, thereby causing various diseases, including brain damage, movement disorders, cognitive dysfunction and kidney failure.5–7 There are many previous studies for the detection of mercury ions with different methods, and we have compared the present method with the others, and a table was added (Table 1).8–11 While other methods can detect Hg2+, they can't be reused; and the synthesis methods of other methods are cumbersome and not easy to operate. Therefore, it is necessary to develop a sensitive, fast and low-cost method for detection of Hg2+.
Table 1 Compared the present method with the others
Structural of compound |
Molecular formula |
Solvent |
Repeatability |
Reference |
|
C24H22N4S |
Acetonitrile |
No |
8
|
|
C27H29N3OSR |
PBS buffer (containing 20% DMF as a co-solvent) |
No |
9
|
|
C42H45N5O3S |
Ethanol/water, (1 : 1) |
No |
10
|
|
C13H12N2O2S |
0.5 M H2SO4 aqueous solution |
No |
11
|
|
C3H34N6O2 |
DMSO/H2O (1/1, v/v) |
Yes |
This work |
In recent years, there have been many ways to detect cations and anions such as ratiometric fluorescent paper sensor,12 silica-based SERS chip.13,14 Schiff base fluorescent probes have been drawn great attention and widely used in detection of heavy metal ions.15 The C
N structure in Schiff base has strong chelating ability, and more importantly, these chelating processes always produce strong fluorescence release.16,17 Pyrazole derivatives are interesting heterocyclic compounds bearing five membered heterocycles and useful raw materials to synthesize various biological molecules. Due to their strong coordination ability with metal ions, pyrazole based derivatives have been widely used for the development of fluorescent probes to detect heavy metal ions, such as Zn2+, Cd2+, and Hg2+.18–20 Furthermore, pyrazole derivatives are always employed as important capping motifs in probing molecules, which can be used as ligands for selective recognition metal ions in coordination chemistry. Therefore, pyrazoles are attracting building blocks for designing and synthesizing a series of heterocyclic compounds, such as optical brighteners, and UV stabilizers, and fluorescent probes.21
In this paper, a novel fluorescent probe Pyr-Rhy was described, which was synthesized by modification of rhodamine with a pyrazole derivative (Fig. 1). This probe exhibited high fluorescent “turn-on” response toward Hg2+ in aqueous DMSO solution, and this process was accompanied with a sensitive fluorescence color change that could not be interfered by other coexisting metal ions. Test results showed that the recognition process of sensor Pyr-Rhy toward Hg2+ was completed instantly, and the detection limit was found to be as low as 2.07 × 10−8 M. Therefore, sensor Pyr-Rhy could be used for quantitative estimation of Hg2+ in aqueous media.
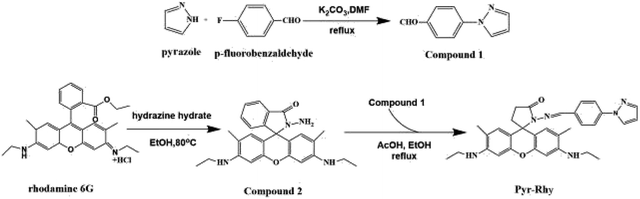 |
| Fig. 1 Synthesis of pyrazole-based fluorescent probe Pyr-Rhy. | |
2. Materials and methods
2.1. General information
In this experiment unless otherwise noted, all reagents and materials were derived from commercial, which without further refinement. Pyrazole, p-fluorobenzaldehyde, rhodamine 6G, hydrazine hydrate and glacial acetic acid were purchased from Aladdin. Distilled water was produced in our lab. Different metal ion solutions were prepared with the corresponding inorganic salts. Nuclear magnetic spectrometer is Bruker AV-400 spectrometer; infrared spectrometer is Bruker ALPHA FT-IR spectrometer fluorospectrophotometer is Hitachi F-4600 and scanning speed was 2400 nm min−1.
2.2. Synthesis
2.2.1 Synthesis of compound 1.
Pyrazole (1.36 g, 20 mmol), p-fluorobenzaldehyde (2.48 g, 20 mmol) and anhydrous potassium carbonate (6.90 g, 50 mmol) were dissolved in 50 mL of DMF, the mixture was allowed to warm 110 °C and stirred at this ambient temperature for 24 h. After TLC showed the conversion of the starting material, the mixture was cooled to room temperature, filtered.22 The filtrate was diluted with 400 mL of distilled water and the mixture was extracted with 100 mL of acetic ether, the organic layer was dried with anhydrous sodium sulfate and then evaporated, the resultant residue was purified by a column chromatography (petroleum/ethyl acetate, 7/1) to give compound 1 was a white powder (3.34 g, yield 91%); mp 82–84 °C; 1H NMR (400 MHz, DMSO-d6) δ 10.00 (s, 1H), 8.68 (d, J = 2.5 Hz, 1H), 8.06 (dd, J = 8.7 Hz, 4H), 7.85 (t, J = 6.4 Hz, 1H), 6.67–6.58 (m, 1H); 13C NMR: (100 MHz, DMSO-d6) δ 191.87, 143.67, 142.21, 133.65, 131.15, 128.44, 118.27, 108.88 ppm; ESI-TOF HRMS (m/z): calcd for C10H8N2O+, [M + H]+, 172.0729; found, 172.0731.
2.2.2 Synthesis of compound 3.
To the mixture of rhodamine 6G 2 (9.85 g, 20 mmol) in 300 mL of ethanol was added an excess of hydrazine hydrate (0.95 g, 20 mmol), the mixture was heated to 80 °C and refluxed for 12 h to give a dark red clear transparent solution. Then, the mixture was subjected to decompression and evaporation till a few solid was formed. After which, the mixture was poured into 200 mL of deionized water, rested for 3 hours, and filtered.23 The solid was washed alternately with distilled water and ethanol several times, and dried in vacuo to afford compound 3 as a pink solid (7.88 g, yield 70%); 1H NMR (400 MHz, DMSO-d6) δ 7.79–7.71 (m, 1H), 7.50–7.41 (m, 2H), 6.98–6.87 (m, 1H), 6.26 (s, 2H), 6.10 (s, 2H), 4.99 (t, J = 5.3 Hz, 2H), 4.21 (s, 2H), 3.32 (s, 1H), 1.88 (d, J = 9.9 Hz, 6H), 1.21 (t, J = 7.0 Hz, 6H); 13C NMR: (100 MHz, DMSO-d6) δ 165.19, 152.05, 151.33, 147.34, 132.27, 129.47, 127.98, 126.98, 123.40, 122.11, 117.77, 105.00, 95.86, 64.95, 37.44, 17.01, 14.19 ppm; ESI-TOF HRMS (m/z): calcd for C26H28N4O2, [M + H]+, 429.22; found, 429.64.
2.2.3 Synthesis of sensor Pyr-Rhy.
Compound 3 (1.72 g, 10 mmol) and compound 1 (4.56 g, 10 mmol) were dissolved in 40 mL of ethanol, the mixture was heated to 80 °C and refluxed for 2 h after a catalytic amount of acetic acid (3.46 mL, 10 mmol) was added. Then the mixture was cooled to room temperature to yield a rose pink precipitate. The mixture was filtered, the solid was washed with ethanol for several times and dried in vacuo to give sensor Pyr-Rhy (4.98 g, yield 79.3%); 1H NMR (400 MHz, DMSO-d6) δ 8.60 (s, 1H), 8.36 (d, J = 2.4 Hz, 1H), 7.78 (d, J = 7.0 Hz, 1H), 7.70 (d, J = 8.6 Hz, 2H), 7.61 (d, J = 1.3 Hz, 1H), 7.52–7.33 (m, 4H), 6.92 (d, J = 7.3 Hz, 1H), 6.44–6.37 (m, 1H), 6.21 (s, 2H), 6.05 (s, 2H), 4.94 (t, J = 5.3 Hz, 2H), 4.22 (t, J = 5.1 Hz, 1H), 1.07 (t, J = 7.1 Hz, 6H), 0.92 (t, J = 7.0 Hz, 4H); 13C NMR: (100 MHz, DMSO-d6) δ 164.36–164.05, 152.00–151.71, 151.48, 148.30, 146.45–146.22, 142.08–141.78, 141.17–140.81, 134.52–134.16, 132.50, 129.50–129.00, 128.29, 127.35, 124.49–124.07, 123.62–122.94, 118.82, 108.75, 105.45, 96.22, 66.05, 37.95, 19.18–18.89, 17.48, 14.64 ppm; ESI-TOF HRMS (m/z): calcd for C32H34N6O2, [M + H]+, 534.66; found, 534.68.
3. Results and discussion
3.1. UV-vis absorption spectral studies of sensor Pyr-Rhy
The UV-vis absorption spectra of sensor Pyr-Rhy toward different metal ions (Ag+, Al3+, Cd2+, Co2+, Cr3+, Cu2+, Hg+, Mg2+, Ni2+ and Zn2+) were studied through using UV-vis spectroscopy.24,25 As shown in Fig. 2, free sensor solution in DMSO/H2O (1/1, v/v) showed one distinct absorption band at 320 nm. However, with the addition of Hg2+ (10 μM, water as solution) to the above solution, a new the observed absorption band at 320 nm decreased obviously. Whereas, with the addition of other metal cations (Ag+, Al3+, Cd2+, Co2+, Cr3+, Cu2+, Mg2+, Ni2+ and Zn2+, water as solution) showed no significant decrease, which indicated the complexation of sensor Pyr-Rhy with Hg2+. These test results displayed that the UV-vis response of sensor Pyr-Rhy has highly specific selection for Hg2+.
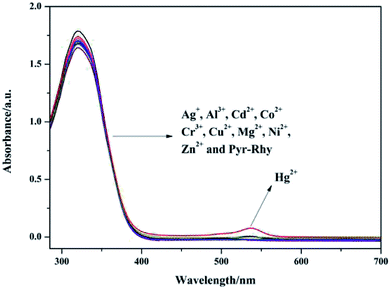 |
| Fig. 2 UV-vis absorption spectra of sensor Pyr-Rhy (10 μM) upon addition of different metal ions (10 μM) in DMSO/H2O (1/1, v/v) solution. | |
3.2. Fluorescence study of sensor Pyr-Rhy
The fluorescence emission behavior of sensor Pyr-Rhy toward different metal ions was investigated in DMSO/H2O (1/1, v/v) solution, and the test results can be seen from Fig. 3.26–28 Clearly, free sensor Pyr-Rhy exhibited one fluorescence emission band at about 575 nm with an excitation at 525 nm. But after the addition of Hg2+ (1.0 equiv.), a significant fluorescence enhancement can be observed with the emission maximum at 575 nm. In contrast, addition of other metal ions exhibited no significant fluorescence enhancement. This fluorescence response of sensor Pyr-Rhy to Hg2+ might be ascribed to the blocked photoinduced electron transfer (PET) process and the chelation-enhanced fluorescence (CHEF) behavior.29,30 Before adding metal ions, the isomerization of C
N double bond of sensor Pyr-Rhy exhibited weak fluorescence emission. While, addition of metal ions leaded to a new π-conjugated structure, which was accompanied with a selective chelation-enhanced fluorescence (CHEF) effect.
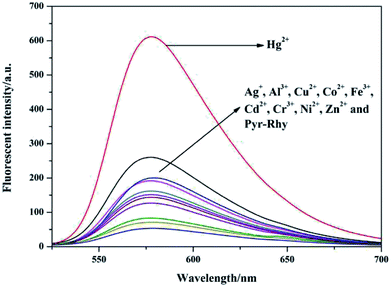 |
| Fig. 3 Fluorescence spectra of sensor Pyr-Rhy (10 μM) in the presence of various metal ions (10 μM) in DMSO/H2O (1/1, v/v) solution (λex = 525 nm). | |
The specificity of sensor Pyr-Rhy toward Hg2+ was investigated by colorimetric experiments. As we can see from Fig. 4a, addition of Hg2+ to the solution of sensor Pyr-Rhy in DMSO/H2O (10 μmol L−1, 1/99, v/v) resulted in a color change from colorless to pink in a few seconds, which suggested that sensor Pyr-Rhy could be used for naked eye recognition of Hg2+ with concentration as low as 10 μmol L−1. In addition, the fluorescence color change from blond to bright yellow was clearly observed under 365 nm UV light after addition of Hg2+. Addition of other metal cations showed no distinct influence on the fluorescence color (Fig. 4b). These outstanding findings demonstrated that sensor Pyr-Rhy could be used to detect Hg2+ qualitatively and quantitatively by changing the color and doubling the spectral signals.
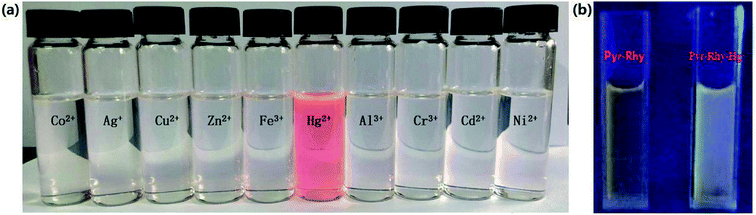 |
| Fig. 4 (a) Colorimetric performance of sensor Pyr-Rhy (10 μM) upon addition of different metal ions (1.0 equiv.) in DMSO/H2O (1/1, v/v) solution; (b) color change induced upon addition of Hg2+ under 365 nm UV lamp. | |
To explore the sensitivity of sensor Pyr-Rhy toward Hg2+, the fluorescence titration experiments were carried out and the results were shown in Fig. 5a. The fluorescence intensity of sensor Pyr-Rhy was gradually enhanced with the increasing of Hg2+ concentration. It was clearly found that the saturation state was reached in presence of 1.0 equiv. of Hg2+, which proved the 1
:
1 binding stoichiometry of sensor Pyr-Rhy with Hg2+. This fluorescent behavior was ascribed to the coordination of sensor Pyr-Rhy with Hg2+, which decreased the PET process and C
N isomerization. Moreover, there was an excellent linear relationship between the fluorescence intensity and the concentration of Hg2+ (R2 = 0.99163) (Fig. 5b). Based on this, the detection limits of sensor Pyr-Rhy with Hg2+ was calculated to be as low as 2.07 × 10−8 M (DL = 3Sb/S).
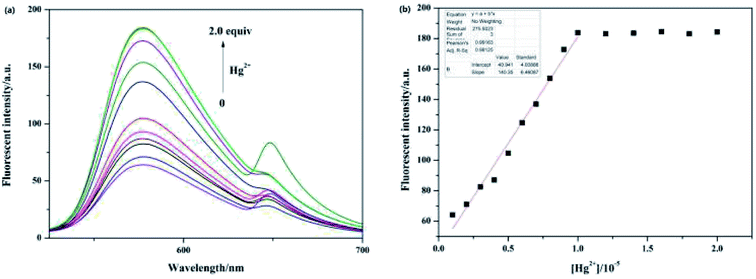 |
| Fig. 5 (a) The fluorescence spectra of sensor Pyr-Rhy (10 μM) with the increasing concentration of Hg2+ ion (0–2.0 equiv.) in ethanol solution; (b) the linear fit between sensor Pyr-Rhy and Hg2+ ion. | |
3.3. pH effects
The effect of pH on fluorescence response of sensor Pyr-Rhy toward Hg2+ was determined over a wide range of pH (1.0–14.0). In this test, hydrochloric acid and sodium hydroxide were used to adjust the pH values. As shown in Fig. 6, the fluorescence intensity of sensor Pyr-Rhy increased with the increasing of pH value (pH ≤ 4.0). And with the increasing of pH (pH = 5–12), the fluorescence intensity was observed to be held steady. These results proved that this probe has potential application value to test Hg2+ within a biological scale of pH values.
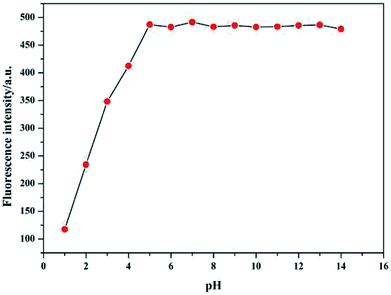 |
| Fig. 6 Effect of pH on the fluorescence intensity of sensor Pyr-Rhy toward Hg2+ in DMSO/H2O (1/1, v/v) solution. | |
3.4. Competition experiment and reversibility study
High selectivity toward Hg2+ over the other metal ions is an important parameter, which is helpful to study the performance of sensor Pyr-Rhy. Therefore, the competition experiments were carried out in the presence of Hg2+ (1.0 equiv.) mixed with other metal ions (1.0 equiv.) in aqueous DMSO media (DMSO/H2O, 1/1, v/v), and the result was shown in Fig. 7. The selectivity of sensor Pyr-Rhy toward Hg2+ ions over other competitive cations was remarkably high, illustrating that sensor Pyr-Rhy was capable of fluorescent recognition of Hg2+ with high selectivity and sensitivity in aqueous DMSO solution (DMSO/H2O, 1/1, v/v).
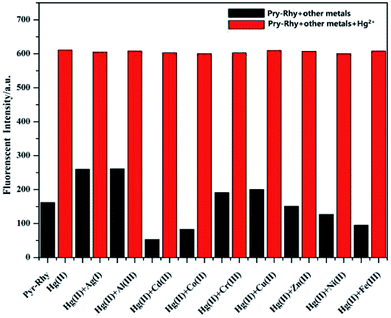 |
| Fig. 7 The black bars represent the fluorescent intensity of sensor Pyr-Rhy and various metal ions (1.0 equiv.) in DMSO/H2O (1/1, v/v) solution; the red bars represent the fluorescent intensity of sensor Pyr-Rhy and Hg2+ (1.0 equiv.) in DMSO/H2O (1/1, v/v) solution. | |
Reversibility study was carried out to explored the reuse property of sensor Pyr-Rhy toward Hg2+. Reversibility is a key prerequisite in designing and developing novel chemosensors for practical applications. The reversibility of the recognition process of sensor Pyr-Rhy was performed by adding a strong chelating agent EDA (Fig. 8).31,32 The addition of excessive EDA to a mixture of sensor Pyr-Rhy and Hg2+ in ethanol solution resulted in diminution of the fluorescence intensity, due to demetalation of Hg2+ from the corresponding complex. Meanwhile, the solution color was changed from fluorescent yellow to colorless, which indicated the regeneration of chemosensor Pyr-Rhy. However, when Hg2+ continued to be added to the mixed solution, a strong fluorescence enhancement was observed and the solution color was changed from colorless to fluorescent yellow, which demonstrated the excellent reversible property of sensor Pyr-Rhy.
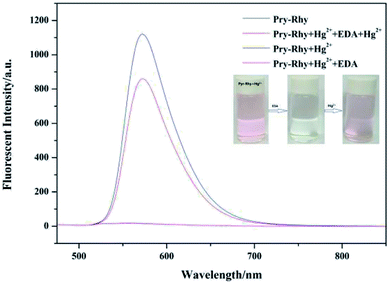 |
| Fig. 8 Changes in emission spectra of Pyr-Rhy in the presence of Hg2+ and EDA in ethanol solution. Inset: color change induced upon addition of EDA. | |
3.5. Contact mode detection between sensor Pyr-Rhy and Hg2+
The recognition of sensor Pyr-Rhy toward Hg2+ was tested on pretreated filter paper to explore its versatility application as a simple and efficient solid-state probe. The test strips were prepared by immersing the filter paper into a saturated solution of sensor Pyr-Rhy in DMSO for a few seconds. Then, these strips dried in air and subsequently treated with Hg2+ solution (1.0 × 10−3 mol L−1) in water for solid state experiments. The filter paper soaked with sensor Pyr-Rhy (1.0 × 10−3 mol L−1, DMSO as solution) showed white and purple colors under sunlight and 365 nm UV light (Fig. 9), respectively. After soaking with Hg2+ (1.0 × 10−3 mol L−1, water as solution), the colors were turned to pink and dull red under sunlight and 365 nm UV light, respectively. Therefore, this solid-state method offers a convenient and cost-effective strategy for naked-eye detection of Hg2+ ion.
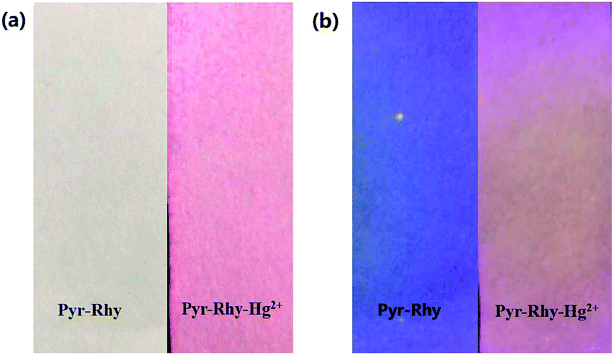 |
| Fig. 9 Photographs showing the color changes of sensor Pyr-Rhy before and after addition of Hg2+ under (a) sunlight and (b) 365 nm UV light. | |
3.6. Proposed binding mode of sensor Pyr-Rhy with Hg2+ ion
The binding mode of sensor Pyr-Rhy toward Hg2+ was investigated through IR spectra (Fig. 10). Free sensor Pyr-Rhy showed stretching vibration bands assignable to –OH and CH
N at 3422 and 1674 cm−1, respectively (Fig. 10a). While complexation of sensor Pyr-Rhy with Hg2+ resulted in the obvious shift of –OH stretching vibration band from 3422 cm−1 to 3432 cm−1 (Fig. 10b). Furthermore, the characteristic stretching vibration band of CH
N and phenolic O–H changed obviously, which indicated that the aldimine nitrogen atom of sensor Pyr-Rhy was involved in the chelating with Hg2+. This result was further confirmed by the 1H NMR spectra recorded in DMSO-d6 as shown in Fig. 11. The H proton signals of phenolic OH and aldimine CH
N in free sensor Pyr-Rhy were located at δ 8.74 and 8.50 ppm, respectively. However, upon addition of Hg2+ (1.0 equiv.), these two signals shifted to lower δ values (8.59 ppm for OH, and 8.36 ppm for CH
N). These data strongly supported the previous conclusion that complexation reaction of sensor Pyr-Rhy with Hg2+ occurred at the CH
N position, and thereby leading to the obvious migration of intramolecular electrons and H proton signals of CH
N and OH.
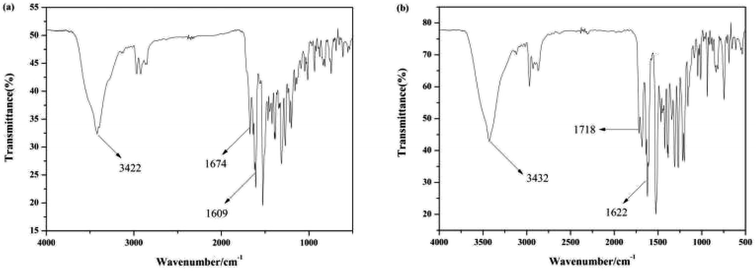 |
| Fig. 10 (a) The FT-IR spectrum of sensor Pyr-Rhy; (b) binding FT-IR spectrum of Pyr-Rhy–Hg2+. | |
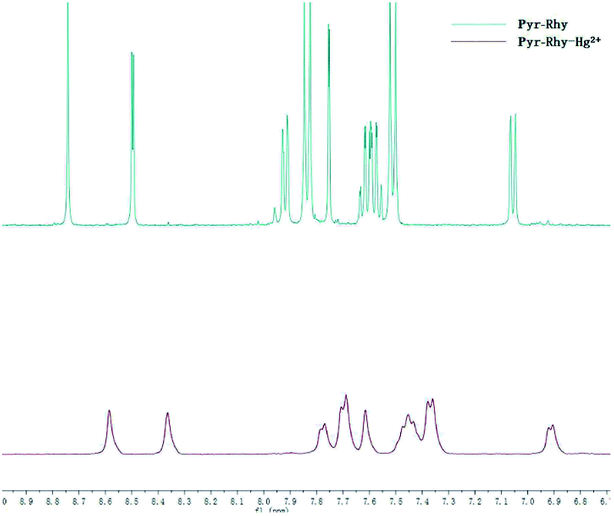 |
| Fig. 11
1H NMR spectra of sensor Pyr-Rhy and complex Pyr-Rhy–Hg2+ in DMSO-d6. | |
In addition, the Job's plot of sensor Pyr-Rhy with Hg2+ in DMSO/H2O (1/1, v/v) solution was also measured. As depicted in Fig. 12, the maximum emission intensity was measured for a molar fraction of 0.5, which gave a solid evidence for the formation of a 1
:
1 complex of sensor Pyr-Rhy with Hg2+. Furthermore, the R value also proved the 1
:
1 stoichiometric ratio for the formation of sensor Pyr-Rhy–Hg2+ complex. The 1
:
1 binding stoichiometry was further confirmed by Benesi–Hildebrand method, the plot of 1/(F − F0) against 1/[Hg2+] showed an excellent linearity (R2 = 0.99163) (Fig. 5b), which strongly supported the 1
:
1 binding stoichiometry of the sensor Pyr-Rhy and Hg2+. The proposed binding mechanism of sensor Pyr-Rhy with metal ions is shown in Fig. 13.33–36 Free sensor Pyr-Rhy contains two equilibrium conformations owing to the isomerization of C
N bond, which can lead to weak fluorescence emission. After adding specific metal ions, the isomerization of C
N double bond is severely restrained due to the block of photoinduced electron transfer (PET). This process generates a new π-conjugated structure, which can result in the increase of structure rigidity and the enhancement of fluorescence intensity.
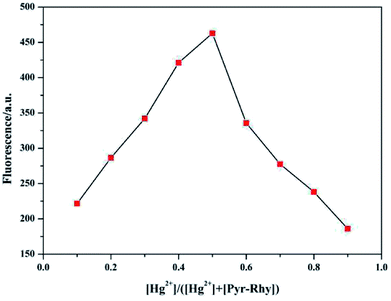 |
| Fig. 12 Job's plot for sensor Pyr-Rhy and Hg2+ in DMSO/H2O (1/1, v/v) solution. | |
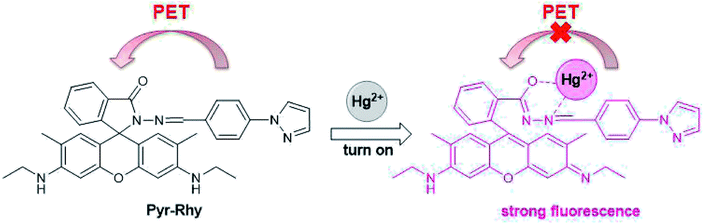 |
| Fig. 13 Proposed sensing mechanism of sensor Pyr-Rhy toward Hg2+. | |
4. Conclusions
In summary, a novel efficient fluorescent probe Pyr-Rhy for naked-eye detection of Hg2+ had been successfully synthesized, using the commercial available pyrazole and rhodamine 6G as the starting materials. This probe exhibits high anti-interference performance, rapid response, high sensitivity and excellent selectivity toward Hg2+ over other coexisted metal ions within wide pH range. The filter paper test strips demonstrate that probe Pyr-Rhy has high value in the practical applications. Importantly, the probe can be used to trace amounts of hazardous Hg2+ ions in water with good recoveries and less relative standard deviations, indicating that the developed probe has good accuracy and precision for the analysis of trace Hg2+ in practical samples.
Conflicts of interest
The authors declare no competing interests.
Acknowledgements
This work was funded by the Natural Science Foundation of Shandong Province (No. ZR2018PB007), the Shandong Provincial Natural Science Foundation of China (ZR2017LC005), the Key Research and Development Program of Shandong Province of China (2018 CXGC1107), and the Shandong Provincial Natural Science Foundation of China (ZR2016EMM13).
References
- N. Schüwer, M.-L. Tercier-Waeber, M. Danial and H.-A. Klok, Aust. J. Chem., 2012, 65, 1104–1109 CrossRef.
- S. Havarinasab and P. Hultman, Autoimmun. Rev., 2005, 4, 270–275 CrossRef CAS.
- R. Falter and H. F. Schöler, J. Chromatogr. A, 1994, 675, 253–256 CrossRef CAS.
- P. B. Tchounwou, W. K. Ayensu, N. Ninashvili and D. Sutton, Environ. Toxicol., 2003, 18, 149–175 CrossRef CAS.
- T. W. Clarkson, L. Magos and G. J. Myers, N. Engl. J. Med., 2003, 349, 1731–1737 CrossRef CAS.
- T. W. Clarkson and L. Magos, Crit. Rev. Toxicol., 2006, 36, 609–662 CrossRef CAS.
- M. R. Knecht and M. Sethi, Anal. Bioanal. Chem., 2009, 394, 33–46 CrossRef CAS.
- G. Hennrich, H. Sonnenschein and U. Resch-Genger, J. Am. Chem. Soc., 1999, 121, 5073–5074 CrossRef CAS.
- W. Lin, X. Cao, Y. Ding, L. Yuan and L. Long, Chem. Commun., 2010, 46, 3529–3531 RSC.
- Q.-J. Ma, X.-B. Zhang, X.-H. Zhao, Z. Jin, G.-J. Mao, G.-L. Shen and R.-Q. Yu, Anal. Chim. Acta, 2010, 663, 85–90 CrossRef CAS.
- K. Tsukamoto, Y. Shinohara, S. Iwasaki and H. Maeda, Chem. Commun., 2011, 47, 5073–5075 RSC.
- Y. Wang, C. Zhang, X. Chen, B. Yang, L. Yang, C. Jiang and Z. Zhang, Nanoscale, 2016, 8, 5977–5984 RSC.
- T. Xuan, X. Yang, S. Lou, J. Huang, Y. Liu, J. Yu, H. Li, K.-L. Wong, C. Wang and J. Wang, Nanoscale, 2017, 9, 15286–15290 RSC.
- J. Zhang, L. He, P. Chen, C. Tian, J. Wang, B. Liu, C. Jiang and Z. Zhang, Nanoscale, 2017, 9, 1599–1606 RSC.
- L. Wang, W. Qin and W. Liu, Inorg. Chem. Commun., 2010, 13, 1122–1125 CrossRef CAS.
- H. Miyasaka, N. Matsumoto, H. Ōkawa, N. Re, E. Gallo and C. Floriani, J. Am. Chem. Soc., 1996, 118, 981–994 CrossRef CAS.
- N. Raman, S. Ravichandran and C. Thangaraja, J. Chem. Sci., 2004, 116, 215–219 CrossRef CAS.
- M. Guerrero, J. Pons, M. Font-Bardia, T. Calvet and J. Ros, Aust. J. Chem., 2010, 63, 958–964 CrossRef CAS.
- V. S. Elanchezhian and M. Kandaswamy, Inorg. Chem. Commun., 2010, 13, 1109–1113 CrossRef.
- A. Ciupa, M. F. Mahon, A. Paul and L. Caggiano, Org. Biomol. Chem., 2012, 10, 8753–8757 RSC.
- B. Willy and T. J. Mueller, Eur. J. Org. Chem., 2008, 4157–4168 CrossRef CAS.
- X.-Y. Wang, C.-G. Niu, L.-J. Guo, L.-Y. Hu, S.-Q. Wu, G.-M. Zeng and F. Li, J. Fluoresc., 2017, 27, 643–649 CrossRef CAS.
- V. B. Bojinov, A. I. Venkova and N. I. Georgiev, Sens. Actuators, B, 2009, 143, 42–49 CrossRef.
- V. Amendola and M. Meneghetti, J. Phys. Chem. C, 2009, 113, 4277–4285 CrossRef CAS.
- W. Haiss, N. T. Thanh, J. Aveyard and D. G. Fernig, Anal. Chem., 2007, 79, 4215–4221 CrossRef CAS.
- J. Suurkuusk, B. Lentz, Y. Barenholz, R. Biltonen and T. Thompson, Biochemistry, 1976, 15, 1393–1401 CrossRef CAS.
- B. Ou, M. Hampsch-Woodill and R. L. Prior, J. Agric. Food Chem., 2001, 49, 4619–4626 CrossRef CAS.
- Y. Chen, C. Zhu, Z. Yang, J. Chen, Y. He, Y. Jiao, W. He, L. Qiu, J. Cen and Z. Guo, Angew. Chem., Int. Ed., 2013, 52, 1688–1691 CrossRef CAS.
- M. Y. Chae and A. W. Czarnik, J. Am. Chem. Soc., 1992, 114, 9704–9705 CrossRef CAS.
- L.-J. Fan, Y. Zhang and W. E. Jones, Macromolecules, 2005, 38, 2844–2849 CrossRef CAS.
- S. Zhang, Q. Niu, L. Lan and T. Li, Sens. Actuators, B, 2017, 240, 793–800 CrossRef CAS.
- T. Sun, Q. Niu, Y. Li, T. Li and H. Liu, Sens. Actuators, B, 2017, 248, 24–34 CrossRef CAS.
- L. Lan, Q. Niu, Z. Guo, H. Liu and T. Li, Sens. Actuators, B, 2017, 244, 500–508 CrossRef CAS.
- W. Zhu, L. Yang, M. Fang, Z. Wu, Q. Zhang, F. Yin, Q. Huang and C. Li, J. Lumin., 2015, 158, 38–43 CrossRef CAS.
- S. Erdemir, S. Malkondu and O. Alici, Color. Technol., 2015, 131, 32–37 CAS.
- H. N. Kim, M. H. Lee, H. J. Kim, J. S. Kim and J. Yoon, Chem. Soc. Rev., 2008, 37, 1465–1472 RSC.
|
This journal is © The Royal Society of Chemistry 2019 |