DOI:
10.1039/D4RA03837A
(Review Article)
RSC Adv., 2024,
14, 29039-29051
Magnetic nanoparticles and possible synergies with cold atmospheric plasma for cancer treatment
Received
24th May 2024
, Accepted 29th July 2024
First published on 12th September 2024
Abstract
The biomedical applications of magnetic nanoparticles (MNPs) have gained increasing attention due to their unique biological, chemical, and magnetic properties such as biocompatibility, chemical stability, and high magnetic susceptibility. However, several critical issues still remain that have significantly halted the clinical translation of these nanomaterials such as the relatively low therapeutic efficacy, hyperthermia resistance, and biosafety concerns. To identify innovative approaches possibly creating synergies with MNPs to resolve or mitigate these problems, we delineated the anti-cancer properties of MNPs and their existing onco-therapeutic portfolios, based on which we proposed cold atmospheric plasma (CAP) to be a possible synergizer of MNPs by enhancing free radical generation, reducing hyperthermia resistance, preventing MNP aggregation, and functioning as an innovative magnetic and light source for magnetothermal- and photo-therapies. Our insights on the possible facilitating role of CAP in translating MNPs for biomedical use may inspire fresh research directions that, once actualized, gain mutual benefits from both.
1. Introduction
Nanoparticles (NPs), defined as solid colloidal particles ranging in size from 10 to 1000 nm, have been extensively used in biomedical applications due to the many benefits they have demonstrated as compared with larger materials such as increased magnetic properties and surface-to-volume ratio.1 NPs can be roughly grouped into four nanosystems, i.e., metallic NPs such as gold (Au) and silver (Ag), bimetallic or alloy NPs, including iron-platinum (FePt) and iron–cobalt (FeCo), metal oxide NPs such as titanim dioxide (TiO2), cerium dioxide (CeO2), silica (SiO2) and zinc oxide (ZnO), and magnetic NPs (MNPs) such as magnetite (Fe3O4) and cobalt ferrite (CoFe2O4).2
Due to unique biological, chemical, and magnetic properties of MNPs such as biocompatibility, chemical stability, and high magnetic susceptibility, MNPs have gained the most popularity among NPs in biomedical applications.3 MNPs can act as diagnostic probes for multimodal tracking and contrast agents for magnetic resonance imaging by virtue of their unique physical properties, which have been extensively reviewed.4 In addition, MNPs provide high magnetic moments that render them attractive for hyperthermia onco-therapy. For instance, the feasibilities of using CoFe2O4 NPs and MnFe2O4 NPs for magnetic hyperthermia have been proposed due to their high chemical stability, favorable biocompatibility, high magnetization and good magnetic susceptibility.5–13 Despite advances on the medical applications of MNPs, several obstacles still remain such as the relatively low treatment efficacy and therapeutic resistance that mitigate the clinical outcome of MNP-based modalities, and the biosafety issue that may impose environmental hazards and health risks.
By introducing the material basics and anti-cancer properties of MNPs, as well as their existing onco-therapeutic modalities and remaining issues, we explored possible synergies of MNPs with cold atmospheric plasma (CAP), an emerging redox modulatory tool for cancer treatment, towards improved therapeutic outcome of MNP-based treatment regimens with mitigated remaining issues, with the aim of truly translating MNPs into clinical use and gaining mutual benefits from both therapeutics.
2. Basics of MNPs
Iron oxide has different oxidation states including iron(II) oxide (FeO), iron(III) oxide (Fe2O3) and iron(II,III) oxide (Fe3O4), where Fe2O3 has different crystalline polymorphs, i.e., α-Fe2O3, β-Fe2O3, γ-Fe2O3 and ε-Fe2O3 (Fig. 1). Among these MNPs, γ-Fe2O3 (also named maghemite) and Fe3O4 have the highest biocompatibility, with Fe3O4 being widely investigated for biomedical use14 such as magnetic hyperthermal therapy15 and phototherapy.16,17 However, Fe3O4 is easily oxidized and thus requires coating with a biocompatible shell that may prevent MNP agglomeration and enable additional functionalization or conjugation. Example coatings include polymers,18–21 ceramics22,23 and metals.24 While the internal inorganic metal core takes on the primary functions under the magnetic field, the coated shell prevents the MNPs from agglomeration through forming a homogeneous and dimensionally stable hydrocolloid dispersion system and attenuating immune reactions.25 The magnetic properties of iron oxide NPs can be further improved by doping with magnetically susceptible elements such as manganese (Mn), cobalt (Co) and nickel (Ni),9 with Mn and Co doped ferrites conferring the most promise for biomedical applications.
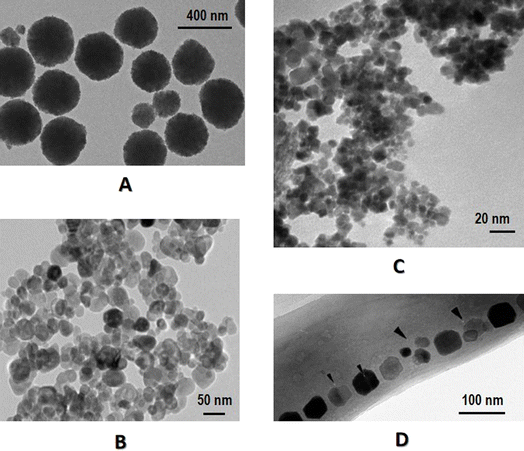 |
| Fig. 1 Transmission electron microscopy images of different types of MNPs. (A) Fe3O4, (B) Fe2O3, (C) FeO, (D) magnetosome. Panel A was reproduced from Fig. 3A of ref. 108 with permission. Panel B was reproduced from Fig. 2A of ref. 109 under Creative Commons Attribution License (CC 4.0). Panel C was reproduced from Fig. 1A of ref. 110 under Creative Commons Attribution 3.0 Unported License. Panel D was reproduced from Fig. 2A of ref. 111 with permission. | |
The presence of magnetosomes, unique organelles composed of membrane-enveloped crystals of a magnetic iron mineral, makes genetically magnetizing non-magnetotactic cells possible and opens an avenue for exciting innovative biotechnological applications. For instance, one would be able to design MNPs harboring tailored magnetic properties via combining genes from cells producing diverse magnetosomes for numerous nanotechnological and biomedical applications. This, however, may require adaptations of both the implanted gene cassettes and the host to achieve successful transfer of magnetosome biogenesis pathways into distant non-magnetotactic cells and optimized functionalities26 such as gene-based contrast for magnetic resonance imaging.27
2.1 Characteristics
MNPs have superparamagnetism and magneto-thermal effect, and are featured with large surface area and high adsorption capacity. Superparamagnetism refers to a form of magnetism appearing in small MNPs (typically with a diameter of less than 50 nanometers) that can randomly flip directions under the influence of temperature. MNPs are considered to be at the superparamagnetic state when the time used to measure NP magnetization is much longer than the Néel relaxation time (defined as the typical time between two flips) in the absence of an external magnetic field. MNPs can be magnetized by an external magnetic field when being attracted at the superparamagnetic state.28 MNPs show the magneto-thermal effect in high-frequency magnetic fields that can be used to kill tumor cells. This is known to rely on two heating mechanisms, i.e., relaxation loss and hysteresis loss for alternating magnetic field (AMF)-induced MNPs.29 Besides the therapeutic role of MNPs, they can also act as the delivery vehicles due to their large surface area that allows them to carry a wide variety of small molecules such as proteins and RNAs30 and high adsorption capacity that enables them for effective enrichment, separation, and directional movement.31
2.2 Preparation
The inorganic metal core of MNPs can be prepared by co-precipitation,32 pyrolysis,33 and microemulsions.34 Co-precipitation is one of the most commonly used and simplest approaches for MNP core preparation that is based on the simultaneous precipitation of Fe2+ and Fe3+ saline solutions by adding weak or strong bases. Using this method, spherical MNP cores can be achieved in the range of 30–100 nm; yet strong MNP aggregation may be caused due to the large specific surface area of the prepared MNP cores. Many methods have been proposed to resolve this issue, such as modifying the surface of MNP cores with a bioactive coating, preparing them under a magnetic field, or using alkanolamines as the bases. Pyrolysis refers to the preparation of MNP cores via thermal decomposition of organo-iron precursors in high boiling point organic solvents in the presence of stabilizing surfactants.33 MNP cores obtained using this approach can have a relatively high crystallinity. The microemulsion method refers to the mutual dispersion of two incompatible liquids that are thermodynamically stable. The main advantage of this approach lies in the controllability of the size and morphology of MNP cores it forms that is actualized by varying the size of micelles.34
The organic shells can be coated on MNP cores by organic or inorganic modifications. Commonly used organic materials for MNP encapsulation include, e.g., polymers,35 fatty acids (e.g., oleic acid,36 citric acid,36 lauric acid), and amino acids, among which polymers are the most widely used coating materials. Out of the diversified types of polymer coatings, polyethylene glycol (PEG) and dextran share the highest popularity, where polyethylene glycolized coatings such as PEG ester (C2H4O)n and polyethylene glycolized starch are known to be highly biocompatible. For example, Wang et al. utilized PEG to modify Mn–ZnFe2O4 that was further linked with hyaluronic acid (HA) for targeting tumors over-expressing CD44. The final nanocomposite, namely HA-modified Mn–ZnFe2O4 magnetic NPs (MZF-HA), was shown capable of being enriched in human lung adenocarcinoma cells A549 that expressed high levels of CD44.37 In addition, many stimuli-responsive polymer coatings responsive to, e.g., temperature, pH, light, enzyme, ionic strength, electric and magnetic fields, have emerged. Among these stimuli, temperature and pH are the most widely used in MNP shell design as MNPs swell under acidic environment and temperature control that enable their rapid release at, typically, the target site. Specifically, MNP release is more rapid in the acidic TME as compared with physiological conditions, and the rate of MNP swelling is affected by the temperature. For example, Marziyeh Fathi et al. fabricated pH- and temperature-responsive MNPs by conjugating methotrexate to chitosan (CS) for targeting ovarian cancers; attributing to the protonation of the NH2 functional group of the CS chain that increased MNP swelling and was under pH and temperature control, these MNPs were released faster under cancer-mimicking conditions (40 °C, pH = 5.5) and slower under physiological conditions (37 °C, pH = 7.4), enabling drug release at the target location.38
Inorganic materials such as metals and SiO2 are also prevalent modifiers of MNP cores.39 For instance, Tao et al. utilized Au for MNP surface amendment (Fe3O4–Au) that significantly enhanced the biocompatibility and aqueous stability of MNPs.40 SiO2 is prized for its hydrophilicity, biocompatibility, and non-toxicity that render it more amenable for biomedical applications. For example, Lin et al. synthesized Fe3O4@SiO2 core–shell structured NPs with an appropriate silica layer thickness that achieved good saturation magnetization; by modifying this silica surface with L-selenocystine and folate, they obtained a novel nano-radiosensitizer with enhanced radiotherapeutic effects against HeLa cervical carcinoma and MDA-MB-231 breast cancer cells and little cytotoxicity.41
2.3 Physiochemical characterization
Characterization of MNPs can be classified into physiochemical in vitro and biological in vivo methods. While physiochemical approaches help achieve the best formulation for desired in vivo performance, biological in vivo methods evaluate the longevity, targetability, cellular uptake, pharmacokinetics, safety, efficacy, and toxicity, among others. Here we focus on some commonly considered physiochemical in vitro characterization methodologies.
The size of a MNP can influence cellular uptake and tumor permeability, among others, due to their impact on the enthalpic and entropic properties that govern the adhesion strength between MNPs and cellular receptors.42 The size of the magnetic core of a MNP can be determined using transmission electron microscopy (TEM) (Fig. 1), X-ray diffraction (XRD), small angle X-ray scattering (SAXS), and small angle neutron scattering (SANS), among which TEM is the most commonly used that provides information on the internal microstructure or ultrastructure of core besides the external structure.25 Although TEM measures the actual radius of samples, it suffers from the small sample size due to the time-consuming sample preparation process that may also involve many subjective factors. One way to reduce such subjective influences is to take many images at sufficient magnification.
The local concentration of MNPs exposed to each cell is deterministic on the amount of nanoparticles taken up by a cell despite the stochastic nature of the uptake process itself that some cells will take up more nanoparticles than others purely by chance.43 Techniques determining the concentration of particles in suspension are also available. For example, the concentration of iron oxide materials can be determined using UV-vis spectroscopy. If the iron to be detected was at a low concentration or multiple components need to be measured, inductively coupled plasma (ICP) equipped with either a mass spectrometer (MS) or atomic emission spectroscopy (OES) can be adopted.44
The surface chemistry of MNPs has been shown to affect their cytotoxic effects by altering the amount of serum proteins adsorbed on the surface, where more serum protein absorption is typically associated with lower cell uptake and higher possibility of causing protein conformational changes and cellular immune responses.45 To measure the amount of organic materials on the surface of MNPs, thermal gravimetric analysis is most commonly used. Using this approach, one could gain knowledge on the weight loss and char yield of the organic layer, together with the size distribution of the inorganic core typically obtained from TEM, the number of chains per nm2 on the surface of MNPs can be determined.46,47 Yet, it requires a special caution that this approach assumes only one organic species on the surface of MNPs. To characterize surface modifications of MNPs, nuclear magnetic resonance (NMR) spectroscopy and Fourier transform infrared (FTIR) spectroscopy are commonly used approaches. While NMR may produce undiscernible images due to peak broadening as a result of large field inhomogeneity created by magnetic MNPs, FTIR offers a better option that measures the vibrational energy of the bonds. In addition, Raman spectroscopy (RS) and X-ray photoelectron spectroscopy (XPS) are useful in determining the crystal phase of MNPs, where RS is particularly useful for chemically characterizing ferrite particles.25
The electrostatic potential at the electrical double layer surrounding a MNP in solution is measured by the zeta potential. While MNPs with a zeta potential fell into the range of −10 to +10 mV are considered neutral, those with zeta potentials <−30 or >+30 mV are highly anionic and cationic, respectively. The zeta potential can affect the tendency of a MNP to permeate membranes, with cationic MNPs being more easily to disrupt negatively charged cell membranes and thus showing higher toxicities.48
The colloidal stability and aggregation of MNPs in suspension can be evaluated using the hydrodynamic size distribution of stabilized MNPs, which can be measured using dynamic light scattering (DLS), SAXS and SANS. DLS gains popularity due to its noninvasive and simple nature that measures the fluctuations of the light scattered by dilute colloidal suspensions.49
The saturation magnetization and any presence of hysteresis of MNPs, often reported as the specific absorption rate (SAR, defined as the magnetic power absorbed per unit mass), can be performed via traditional approaches such as superconducting quantum interference devices (SQUID) and vibrating sample magnetometers (VSM).25
3. Therapeutic efficacy of MNPs for cancer treatment
3.1 Inducing cancer cell death
Programmed cell death (PCD) is a form of cell death that can be regulated by a variety of biomolecules and manifested in diversified forms such as apoptosis, autophagic cell death and ferroptosis. Given the crucial roles it played in maintaining cellular homeostasis by removing damaged and senescent cells, escaping PCD becomes a fundamental hallmark of cancer.50
As MNPs can induce magnetic hyperthermia and generate free radicals through, e.g., Fenton reaction, they are able to induce various cell death programs such as cancer cell apoptosis and autophagic cell death (Fig. 2). For example, Sulaiman et al. prepared MNPs that induced apoptosis among human breast cancer cells AMJ13 and MCF7 by causing DNA breaks.51 Using MNPs as drug carriers, Wang et al. loaded paclitaxel (PTX) into fluorescent magnetic poyethy lenimine (PEI)-poly(lactic-co-glycolic acid) (PLGA) NPs for simultaneous cell imaging and drug delivery, which triggered autophagic cell death of human glioblastoma U251 cells by initiating autophagosome formation.52
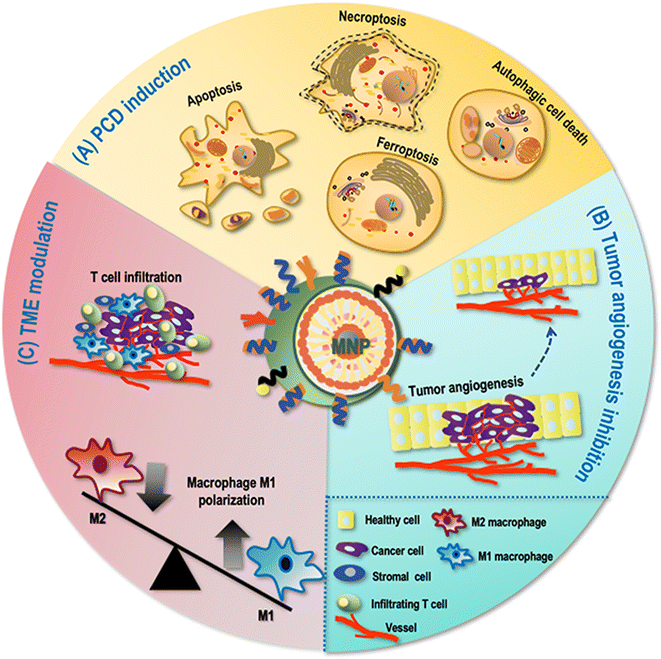 |
| Fig. 2 Onco-therapeutic features of MNPs and possible synergies with CAP. Through inducing magnetic hyperthermia and generating free radicals, MNPs can (A) induce various programmed death of cancer cells such as apoptosis, autophagic cell death and ferroptosis, (B) inhibit cancer cell angiogenesis, (C) and modulate the TME by polarizing macrophage towards the M1 state and enhancing cytotoxic T cell infiltration. CAP can selectively induce various PCDs, reverse the EMT program, and prime macrophages to the M1 state in the TME, and thus may synergize with MNPs due to enhanced ROS generation. | |
Ferroptosis is iron-dependent and featured by lipid peroxidation.53 As iron is commonly used as the magnetic core of MNPs, ferroptosis is a typical PCD induced by MNPs. For instance, Zhang et al. proposed that superparamagnetic iron oxide (SPIO-serum) could induce ferroptosis among ovarian cancer cells by interfering with mitochondrial redox homeostasis; specifically, SPIO-serum led to the accumulation of intracellular irons that triggered oxidative stress within the mitochondria, and decreased expression of glutathione peroxidase 4 (GPX4) that was associated with increased lipid peroxidation.54
3.2 Attenuating cancer cell angiogenesis and migration
Tumor angiogenesis is usually produced in tumor cells in response to various stimuli such as hypoxia, ischemia, and acidosis, abnormal regulation of which plays a crucial role in tumor invasion and metastasis, which is another important cancer hallmark.50
MNPs can inhibit cancer cell angiogenesis through generating the magnetic hyperthermia effect (Fig. 2). Salimi et al. explored the effect of magnetic hyperthermia using fourth-generation polyamidoamine (PAMAM) dendrimer (G4)-functionalized iron oxide NPs (IONPs) in treating breast cancer cells, and found that G4@IONPs were able to reduce tumor angiogenesis via magnetic hyperthermia both in vitro and in vivo.55 Mulens-Arias et al. investigated the effects of polyethyleneimine-SPIO NPs (PEI-SPIO NPs) on endothelial cells and macrophages, and found that PEI-SPIO NPs reduced the number of blood vessels and promoted macrophage infiltration towards reduced endothelial cell migration and angiogenesis both in vitro and in vivo.56
3.3 Modulating the tumor microenvironment
The tumor mass is composed of not only a heterogeneous group of cancer cells but also the tumor microenvironment (TME). The composition of TME, though varying among tumor types and evolving with tumor progression, typically includes immune cells, stromal cells, infiltrating host cells, blood vessels, and extracellular matrix. The TME can not only determine whether the primary tumor is eradicated, metastasizes or establishes dormant micrometastases, but also shape the therapeutic response and immune sensitivity of tumors.
As an important part of the innate immune system and a professional tumor antigen presenting cell, macrophages are affected by iron oxide NPs which have two functionally distinct states, i.e., the M1 pro-inflammatory and anti-tumorigenic state and the M2 anti-inflammatory and pro-tumorigenic state. Specifically, iron oxide NPs were reported capable of shifting macrophages towards the M1 state due to increased reactive oxygen species (ROS) accumulation.57 These M1 macrophages produce and release, e.g., interleukin-1 (IL-1) and vascular endothelial growth factor (VEGF), to support tumor progression by inducing angiogenesis58 (Fig. 2). Zanganeh et al. showed that ferumoxytol can induce macrophage M1 polarization and a concomitant increase in ROS production, leading to significantly inhibited growth of subcutaneous adenocarcinomas and liver metastases in mice.59 Fang et al. emphasized the use of magnetic hyperthermia immunotherapy (through magnetic NPs) in promoting cytotoxic T cell infiltration by altering the phenotype of tumor-associated macrophages and activating dendritic cells.60
4. MNP-based therapeutics
4.1 Magnetothermal therapy
Magnetothermal therapy refers to a therapy in which tumor killing is achieved by exposing MNPs to an appropriate AMF taking advantages of the thermomagnetic effect.61 Cancer cells are more sensitive to hyperthermia than their healthy peers due to the acidic TME. The disorganized vascular network leads to reduced blood flow in cancerous tissues that further decreases the convective cooling rate of the tumor, whereas healthy tissues can dissipate additional heat to adjacent tissues through organized blood flow. Due to the superior stability and safety of metal oxides in vivo, various metals that have been used in MNP design for thermotherapy that include, e.g., Fe, Ni, Mn, Mg and their oxides. Superparamagnetism is a unique phenomenon that occurs in ferromagnetic NPs, and superparamagnetic NPs do not have any residual magnetization once AMF is removed, making them suitable for biomedical applications. By injecting MNPs directly into the tissue or locating them to the tumor site through magnetic field manipulation and applying AMF to heat these MNPs, magnetic hyperthermia can be achieved for cancer cell killing (Fig. 3). For instance, de la Encarnación et al. introduced a hybrid colloidal nanostructure consisting of plasmonic Au nanorods (AuNR) covered by silica shells that was further modified with iron oxide NPs (IONPs); the resulting hybrid nanocomposite, in response to either external magnetic field or near-infrared radiation, demonstrated photothermal effect in treating human glioblastoma cells.62 Similarly, Araya et al. significantly reduced the tumor volume of mice carrying A549 non-small cell lung cancer cells using carboxydextran-coated synthetic superparamagnetic IONP coupled with AMF exposure for 20 min.63 Pan et al. prepared Zn–CoFe2O4@Zn–MnFe2O4 superparamagnetic NPs (ZCMFs) that showed good and highly controllable magneto-thermal properties due to the exchange-coupled magnetism between the core–shell and the doping of Zn2+; using ZCMFs they almost completely inhibited the growth of in situ hepatocellular carcinoma cells.15
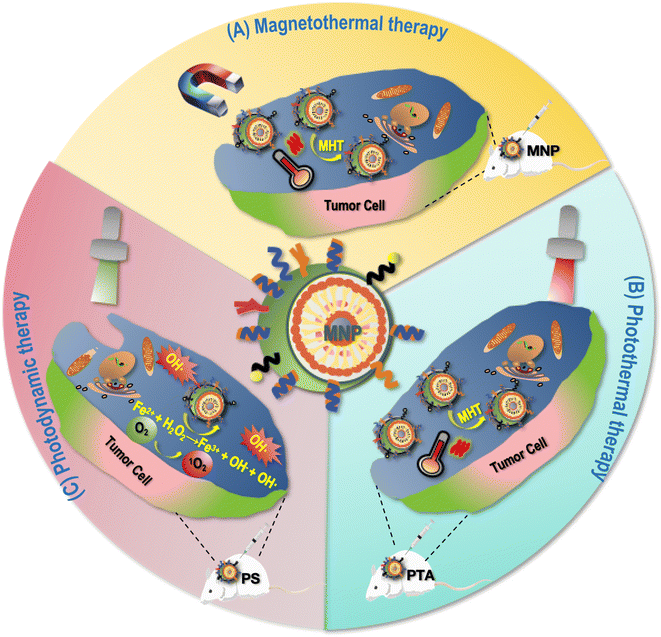 |
| Fig. 3 Onco-therapeutics based on MNPs and possible synergies with CAP. (A) MNPs can be used for magnetothermal therapy where tumor cells are killed by exposing MNPs to an appropriate AMF taking advantages of the thermomagnetic effect. (B) MNPs can be used as PTAs for PTT where MNPs are injected into the body and gathered around tumor cells to convert light energy into heat for cell killing. (C) MNPs can be used as PS for PDT where MNPs are used to absorb and transfer the energy of a specific wavelength of laser to the surrounding oxygen molecules towards the generation of highly cytotoxic ROS for cell killing. CAP can function as a reliable source of light to synergize with MNPs for PTT and PDT, as the spectrum of CAP covers the range of 250–800 nm with a solid peak at 400 nm; and act as a novel source of electromagnetic field for magnetothermal therapy to synergize with MNPs. | |
4.2 Phototherapy
MNP-mediated phototherapies can be categorized into photothermal therapy (PTT) and photodynamic therapy (PDT). While PTT relies on photo-thermal agents (PTAs) to convert light energy into heat for cell killing that are injected into the body and gathered around tumor cells,17 PDT takes advantages of photosensitizer (PS) to absorb and transfer the energy of a specific wavelength of laser to the surrounding oxygen molecules towards the generation of highly cytotoxic ROS for cell killing64 (Fig. 3).
The PTT effect of Fe3O4 magnetic NPs for treating cancers has been demonstrated by many studies. For example, Fe3O4 NPs in spherical, hexagonal, and linear shapes were able to be taken up by esophageal cancer cells without significantly affecting the structure and activity of cells and rapidly generate heat by red and near-infrared laser irradiation (e.g., 808 nm laser irradiation), resulting in significantly damaged cancer cells in vitro and arrested tumor growth in vivo.16
As an example of MNP-mediated PDT, Kuo et al. prepared methylene blue immobilized copper ferrite NPs (MB-CuFe NPs), where CuFe NPs acted as a Fenton catalyst to convert hydrogen peroxide (H2O2) to ROS, and the MB photosensitizer was adsorbed on the surface of this nanocomposite to facilitate the entry of drugs into cells for improved photodynamic therapy under 660 nm laser irradiation and depletion of cervical cancer cells.17 In addition, the efficacy of PDT can be further enhanced by integrating photosensitizers with metal particles utilizing plasmon resonance effects.65,66 For instance, Yao et al. established plasmon-enhanced photodynamic therapy for treating gastric cancers via integrating photosensitizers with gold nanorods.67
5. Issues hindering clinical translation of MNPs
5.1 Low therapeutic efficacy
Although magnetic hyperthermia is non-invasive and the magnetic field makes it possible to target some deep tumors, the limited magneto-thermal efficiency of intravenously injected MNPs and their insufficient accumulation in the tumor site result in a low therapeutic efficiency (Fig. 4). For example, Pan et al. fabricated a nanomaterial consisting of zinc-doped cobalt–iron oxides for liver cancer treatment, which exhibited limited magnetothermal efficiency in vivo due to the relatively low saturation magnetization strength and specific power of loss as well as insufficient accumulation of intravenously injected magnetic nanomaterials at the tumor site as a result of mononuclear phagocyte system (MPS) clearance.15 Thus, understanding the working mechanism of magnetic hyperthermia effect against cancers is the key to resolve such a problem.
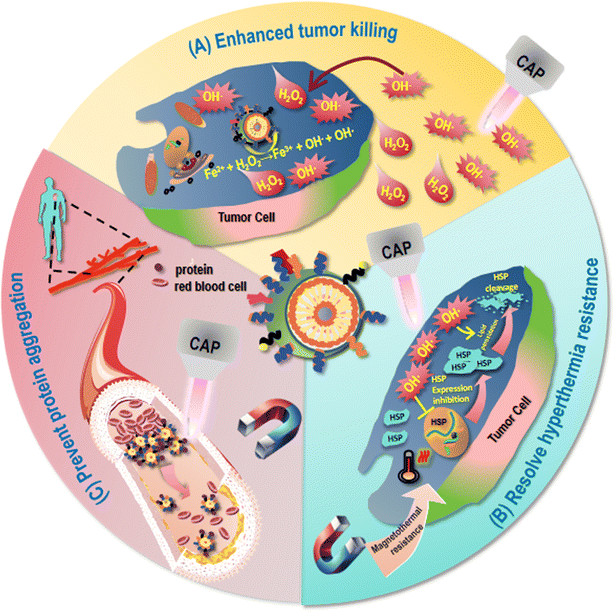 |
| Fig. 4 Issues remained in MNP-based onco-therapeutics and possible solutions brought by CAP. (A) MNPs have relatively low therapeutic efficacy due to the limited magneto-thermal efficiency after intravenous injection and insufficient accumulation in the tumor site. CAP can synergize with MNPs for continuous and enhanced generation of OH˙, and increase H2O2 levels within cancer cells that can be further converted into OH˙ through the Fenton reaction with the presence of iron oxide NPs for enhanced tumor-killing. (B) MNPs may lead to hyperthermia resistance as a result of induced synthesis and accumulation of HSPs in tumor cells. CAP may help resolve hyperthermia resistance by reducing HSP expression given its short-lived component OH˙ and cleaving HSPs by causing lipid peroxidation as a result of ROS accumulation. (C) MNPs may not be degradable due to chemical inertness that may block microcirculation and cause cell dysfunction or death. The excretion of MNPs from the body depends on the size, shape, surface properties of these particles as well as their absorption state in the organism. CAP may mitigate the biosafety issue of MNPs by preventing the formation of clusters of proteins adsorbed on the surface of MNPs for reduced aggregation and secretion blockage. | |
Eukaryotic cells have evolved complex systems to regulate ROS production and cellular responses that control different aspects of the behavior of a cell, the dysregulation of which is a shared feature of cancer cells.68 The basic mechanism of action for MNPs to take on their anti-cancer activities lies in ROS generation. For instance, Maqusood et al. prepared copper ferrite NPs and used them to treat MCF7 breast cancer cells, and found that they reduced the activity of cancer cells through ROS production and GSH depletion.32 Hang et al. isolated CD44+CD133+ human ovarian cancer stem cells (HuOCSCs) from tumors of clear cell ovarian cancer patients, and reported that SPIO NPs inhibited the proliferation, autophagy, invasion, drug resistance, and tumorigenicity of ovarian cancer cells, as well as activated ferroptosis via inducing ROS accumulation and oxidative stress in HuOCSCs.69 Thus, therapeutic strategies relying on redox modulation may create synergies with MNPs for enhanced anti-cancer efficacies that hold a great promise in cancer treatment.
5.2 Hyperthermia resistance
Though MNPs have achieved multiple medical miracles, mild hyperthermia treatment by MNPs may lead to the development of therapeutic resistance as a result of induced synthesis and accumulation of heat shock proteins (HSPs) in tumor cells35,36 (Fig. 4). For instance, HSP70 is activated in response to temperature rise, leading to thermotherapeutic resistance as a result of reduced protein denaturation and enhanced cell survival.70–72 Thus, therapeutic modalities possibly rewiring such a treatment resistance through, e.g., targeting HSPs, shed light on the way of translating MNP-based therapies into clinics.
5.3 Biosafety issue
Another critical issue hindering the translation of MNPs into clinical practice and may be extended to the majority of NPs is the safety issue.73 Degradable NPs can be phagocytosed by cells for degradation into small molecules or dissolved in body fluids on their own after metabolism and excretion. Non-degradable NPs are difficult to be degraded due to chemical inertness and, thus, may block microcirculation and cause cell dysfunction or death.74 As most MNPs suffer from low specific absorption rate (SAR) that requires high levels of internal MNP concentrations for desirable magnetic heat efficacy, the toxicity issue is even more severe for MNPs (Fig. 4). For instance, NanoTherm®, an aminosilane-coated Fe3O4 MNP approved by the European Union, although has demonstrated satisfactory clinical trial results in treating glioblastoma and prostate cancers, required a very high Fe concentration (112 mg mL−1)32 due to its low magneto-thermal conversion efficiency, i.e., 738–985 pH-m2 kg−1. The toxicity of NPs is affected by a variety of factors such as size, shape, and surface features.75 The larger the surface area of the NP is and more chemical interactions are involved, the more difficult it is to control the toxicity, as the surface area of a NP is positively proportional to the amount of ions it releases that may induce inflammatory responses.76 The aggregation state of NPs in organisms plays a dual role in determining their toxicity. That is, aggregation, on one hand, may decrease the toxicity of NPs due to reduced surface area and, on the other hand, increase the local toxicity by accumulating aggregated NPs in specific tissues such as liver and spleen.77 Surface coating or functionalization can be used to reduce the toxicity of NPs. For example, SPIO surface-modified with PEG exhibited improved intracellular distribution, metabolism, biocompatibility, and reduced cytotoxicity.78 However, surface modification cannot fundamentally resolve the toxicity issue. Understanding the excretion mechanisms of MNPs in vivo, and exploring innovative strategies capable of improving the biosafety of MNPs through, e.g., synergistic use may be a possible recipe.
The excretion of MNPs from the body depends on the size, shape, surface properties of these particles as well as their absorption state in the organism. In general, MNPs smaller than 5.5 nm can be excreted through the kidneys, and MNPs larger in size may be metabolized by the liver and excreted by the bile.79 Certain surface modifications can alter the circulation time of NPs in the bloodstream and affect their biochemical stability in vivo, thus affecting their excretion. For example, by absorbing a small amount of proteins on the surface, Au–S strong ligand-bound PEG-modified Au NPs (PEG-GNPs, 6 nm) showed a good dispersion stability under the physiological microenvironment that was conducive to their entry into the liver parenchymal cells for excretion through the bile ducts, and their pass through the filtration barrier of the glomerulus for excretion via the urine. However, Au–N weakly ligand-bound polyethyleneimine-modified Au NPs (PEI-GNPs, 6 nm) and electrostatically bound CS-modified Au NPs (CS-GNPs, 6 nm) were unfavorable for liver clearance due to the large amount of serum proteins absorbed on the surface that led to the formation of large particle clusters identified and ingested by hepatic macrophages and hepatic sinusoidal endothelial cells.80 In addition, tissue and organ structural characteristics, as well as physiological factors are also crucial factors influencing the clearance profiles of nanomaterials in the liver and kidney that are beyond the scope of discussion here. Therefore, therapeutic strategies blocking the formation of large NP clusters may help enhance the secretion and safety of MNPs for clinical use.
6. CAP: a possible synergizer of MNPs for cancer treatment
CAP, produced by exciting a gas into a plasma state (the fourth state of matter) by applying an electric field under the atmospheric pressure and close to room temperature, is comprised of ROS and reactive nitrogen species (RNS).81 These reactive species can be grouped into short-lived such as hydroxyl radical (OH˙), singlet oxygen (O), superoxide (O2−), nitric oxide (NO˙), and long-lived species such as H2O2, ozone (O3), anion (OONO−) and protonated form of peroxynitrite (ONOOH). While the activity of long-lived species can be relatively easily preserved that can enter cells via penetrating through membrane channels such as aquaporins, short-lived species can quench within nanoseconds (e.g., half-life of ˙OH is 10−9 s in vitro and in vivo82) and function through generating secondary short-lived species during interactions with cell membrane or other CAP components.83–86
CAP has been widely used in a variety of medical fields including, e.g., sterilization,87 wound healing,88–90 coagulation,88,91 and cancer therapy.92–95 Ever since the first report on the oncological efficacy of CAP, its therapeutic effects against cancer cells have been demonstrated in various types of cancers, such as triple-negative breast cancer,92 pancreatic cancer93 and lung cancer,94,96 and melanoma.95 The first FDA-approved clinical trial evaluating the efficacy and safety of CAP as an anti-cancer approach has been initiated in 2019 and completed in 2021, where 17 out of 20 late-stage solid cancer patients remained alive, supporting the use of CAP combined with surgery as a safe approach with selectivity against cancer cells and exceptional control on local regional recurrence.97
Yet, the clinical translation of CAP is primarily hindered by several of its unresolved limitations.98 First, given the limited penetration in-depth of CAP, it cannot reach deep tumors and has largely been used for treating surficial cancers such as skin malignancies. Second, the short life span of short-lived species and their leading roles in arresting cancer cells92 make the current use of CAP for cancer treatment primarily limited to be combined with surgery. These make it necessary to involve other techniques towards extended scenarios feasible for receiving CAP treatment.
One possible synergy may be actualized via combining CAP with MNPs given their shared mechanism of action and diversified application forms of MNPs for cancer treatment. The contribution of CAP brought to MNPs for improved treatment efficacy and mitigated side effects can be summarized into ‘enhanced ROS generation’, ‘reduced hyperthermia resistance’, and ‘increased particle secretion’.
6.1 Enhanced ROS generation
CAP has been shown capable of selectively killing cancer cells without harming their healthy peers by causing the oxidative stress of transformed cells due to their more easily disrupted intracellular redox homeostasis. This may be attributed to the higher expression of aquaporins on cancer cells that promote the transport of long-lived free radicals into cells, and the lower cholesterol levels of cancer cell membrane that make them more susceptible to oxidative stress induced damage. When the intracellular oxidative stress of cancer cells exceeds the capacity of the antioxidant defense system, cell death will be triggered through several signaling cascades such as the NRF2 and the NFκB pathways.
CAP can synergize with MNPs for continuous and enhanced generation of the primary short-lived species OH˙ (Fig. 4). Although tumor cells produce more H2O2 than normal cells, these substances are not sufficient to cause effective cancer cell death. CAP can increase H2O2 levels within cancer cells that can be further converted into OH˙ through the Fenton reaction with the presence of iron oxide NPs for enhanced tumor-killing. For instance, CAP and MNPs showed synergistic therapeutic effects on A549 lung cancer cells by significantly inhibiting cell growth via suppressing ERK and AKT signaling, and arresting cancer cell migration via reversing the epithelial–mesenchymal transition (EMT) program.99 Besides, CAP has also been reported capable of regulating macrophage polarization,100 further supporting its possible synergy with MNPs for cancer control (Fig. 2).
6.2 Reduced hyperthermia resistance
It has been found that ROS such as OH˙ can mitigate thermal resistance under hyperthermia by impairing heat shock protein (HSP) expression,101,102 and lipid peroxidation as a result of ROS accumulation can cleave HSPs, thereby attenuating high-temperature-induced heat resistance.103 Given that CAP is enriched with ROS and can effectively induce lipid peroxidation,53 it may help reduce thermal resistance of MNPs induced under mild magnetic hyperthermia (Fig. 4).
6.3 Increased particle secretion
CAP generated using helium as the carrying inert gas could induce protein self-assembly with intact secondary structural features, as evidenced by fluorescence lifetime measurement and SEM analysis.104 These results not only give an outlook for using CAP in achieving protein self-assembly without denaturing the proteins, but also shed light on the possibility of preventing the formation of clusters of proteins adsorbed on the surface of MNPs, mitigating their likelihood of gaining aggregation and secretion blockage (Fig. 4).
6.4 Novel light and electromagnetic field source
CAP may also function as a reliable source of light and electromagnetic field to synergize with MNPs for photo- and magnetothermal therapies. As the spectrum of CAP covers the range of 250–800 nm with a solid peak at 400 nm, it can be used as a novel light source in PDT. For example, protoporphyrin IX (a photosensitizer) encapsulated in polymers was released in response to CAP exposure and resulted in PDT against melanoma cells.105 Besides photons, electrons, ions and neutral radicals, CAP also includes electromagnetic fields,106,107 the possibility of which in synergizing with MNPs for magnetothermal therapy deserves intensive investigations (Fig. 2).
7. Conclusions
Given the roles of MNPs on inducing cancer cell death especially ferroptosis, attenuating cancer cell migration and rewiring the TME, various promising MNP-based therapeutics have been proposed for cancer treatment in the form of, e.g., magnetothermal therapy and phototherapy. However, their limited therapeutic efficacy, hyperthermia treatment resistance and biosafety issue have remained unresolved that largely limited the clinical translation of MNPs for cancer treatment. CAP, an emerging onco-therapeutic modality relying on redox modulation, can possibly synergize with MNPs for enhanced and prolonged ROS generation within cancer cells due to Fenton reactions, and may reduce hyperthermia resistance of MNPs via suppressing HSPs, increase MNP secretion via reducing their likelihood of aggregation, and act as novel light and magnetic field source of MNPs for synergized photo- or magnetothermal therapies. Though promising, relatively little has been devoted to this field, and long-term effect and safety of synergized CAP and MNP treatment regimen require extensive examinations. This paper aims at unveiling these possibilities that deserve underscoring and may guide the future direction.
Abbreviation
MNPs | Magnetic nanoparticles |
CAP | Cold atmospheric plasma |
AMF | Alternating magnetic field |
TME | Tumor microenvironment |
PCD | Programmed cell death |
EMT | Epithelial–mesenchymal transition |
ROS | Reactive oxygen species |
RNS | Reactive nitrogen species |
OH | Hydroxyl radical |
NO | Nitric oxide |
H2O2 | Hydrogen peroxide |
O3 | Ozone |
MPS | Mononuclear phagocyte system |
HSPs | Heat shock proteins |
PTT | Photothermal therapy |
PDT | Photodynamic therapy |
PTAs | Photo-thermal agents |
PS | Photosensitizer |
SPIO | Superparamagnetic iron oxide |
IONPs | Iron oxide nanoparticles |
PEI | Polyethyleneimine |
PLGA | Poly(lactic-co-glycolic acid) |
SAR | Specific absorption rate |
SQUID | Superconducting quantum interference device |
VSM | Vibrating dample magnetometer |
NMR | Nuclear magnetic resonance |
FTIR | Fourier transform infrared |
RS | Raman spectroscopy |
XPS | X-ray photoelectron spectroscopy |
DLS | Dynamic light scattering |
SAXS | Small angle X-ray scattering |
SANS | Small angle neutron scattering |
PAMAM | Polyamidoamine |
G4 | Fourth-generation |
NRF2 | Nuclear factor erythroid 2-related factor 2 |
NFκB | Nuclear factor kappa-light-chain-enhancer of activated B cells |
Data availability
No primary research results, software or code have been included and no new data were generated or analysed as part of this review.
Author contributions
XD conceptualized the insights, and prepared the initial draft. YZ provided the financial support.
Conflicts of interest
All authors have read and agreed the content of the manuscript as well as its submission.
Acknowledgements
This study was funded by the National Natural Science Foundation of China (Grant No. 81972789) and the Key R&D Project of Shaanxi Province (Grant No. 2020GXLH-Z-001). Not applicable.
References
- K. McNamara and S. A. Tofail, Nanosystems: the use of nanoalloys, metallic, bimetallic, and magnetic nanoparticles in biomedical applications, Phys. Chem. Chem. Phys., 2015, 17(42), 27981–27995 RSC.
- C. Xia, et al., Optimistic and possible contribution of nanomaterial on biomedical applications: a review, Environ. Res., 2023, 218, 114921 CrossRef CAS PubMed.
- Z. Karimi, L. Karimi and H. Shokrollahi, Nano-magnetic particles used in biomedicine: core and coating materials, Mater. Sci. Eng., C, 2013, 33(5), 2465–2475 CrossRef CAS PubMed.
- T. D. Schladt, et al., Synthesis and bio-functionalization of magnetic nanoparticles for medical diagnosis and treatment, Dalton Trans., 2011, 40(24), 6315–6343 RSC.
- J. H. Lee, et al., Artificially engineered magnetic nanoparticles for ultra-sensitive molecular imaging, Nat. Med., 2007, 13(1), 95–99 CrossRef CAS PubMed.
- H. M. Joshi, et al., Effects of shape and size of cobalt ferrite nanostructures on their MRI contrast and thermal activation, J. Phys. Chem. C Nanomater. Interfaces, 2009, 113(41), 17761–17767 CrossRef CAS PubMed.
- H. Wu, et al., Solvothermal synthesis of cobalt ferrite nanoparticles loaded on multiwalled carbon nanotubes for magnetic resonance imaging and drug delivery, Acta Biomater., 2011, 7(9), 3496–3504 CrossRef CAS PubMed.
- S. Amiri and H. Shokrollahi, The role of cobalt ferrite magnetic nanoparticles in medical science, Mater. Sci. Eng., C, 2013, 33(1), 1–8 CrossRef CAS PubMed.
- S. Sabale, et al., Superparamagnetic MFe2O4 (M = Ni, Co, Zn, Mn) nanoparticles: synthesis, characterization, induction heating and cell viability studies for cancer hyperthermia applications, J. Mater. Sci. Mater. Med., 2015, 26(3), 127 CrossRef PubMed.
- J. Lu, et al., Manganese ferrite nanoparticle micellar nanocomposites as MRI contrast agent for liver imaging, Biomaterials, 2009, 30(15), 2919–2928 CrossRef CAS PubMed.
- H. Yang, et al., Water-soluble superparamagnetic manganese ferrite nanoparticles for magnetic resonance imaging, Biomaterials, 2010, 31(13), 3667–3673 CrossRef CAS PubMed.
- B. Sahoo, et al., Biocompatible mesoporous silica-coated superparamagnetic manganese ferrite nanoparticles for targeted drug delivery and MR imaging applications, J. Colloid Interface Sci., 2014, 431, 31–41 CrossRef CAS PubMed.
- D. H. Kim, D. E. Nikles and C. S. Brazel, Synthesis and characterization of multifunctional chitosan-MnFe2O4 Nanoparticles for magnetic hyperthermia and drug delivery, Materials, 2010, 3(7), 4051–4065 CrossRef CAS PubMed.
- V. Nica, et al., Cell-membrane-coated and cell-penetrating peptide-conjugated trimagnetic nanoparticles for targeted magnetic hyperthermia of prostate cancer cells, ACS Appl. Mater. Interfaces, 2023, 15(25), 30008–30028 CrossRef CAS PubMed.
- J. Pan, et al., Mild magnetic hyperthermia-activated innate immunity for liver cancer therapy, J. Am. Chem. Soc., 2021, 143(21), 8116–8128 CrossRef CAS PubMed.
- M. Chu, et al., Near-infrared laser light mediated cancer therapy by photothermal effect of Fe3O4 magnetic nanoparticles, Biomaterials, 2013, 34(16), 4078–4088 CrossRef CAS PubMed.
- S. H. Kuo, et al., Fabrication of anisotropic Cu ferrite-polymer core-shell nanoparticles for photodynamic ablation of cervical cancer cells, Nanomaterials, 2020, 10(12), 2429 CrossRef CAS PubMed.
- N. K. Sahu, J. Gupta and D. Bahadur, PEGylated FePt-Fe3O4 composite nanoassemblies (CNAs): in vitro hyperthermia, drug delivery and generation of reactive oxygen species (ROS), Dalton Trans., 2015, 44(19), 9103–9113 RSC.
- S. Mohammadi-Samani, et al., Preparation and assessment of chitosan-coated superparamagnetic Fe3O4 nanoparticles for controlled delivery of methotrexate, Res. Pharm. Sci., 2013, 8(1), 25–33 CAS.
- G. Zhao, et al., Facile solvothermal synthesis of mesostructured Fe3O4/chitosan nanoparticles as delivery vehicles for pH-responsive drug delivery and magnetic resonance imaging contrast agents, Chem. Asian J., 2014, 9(2), 546–553 CrossRef CAS PubMed.
- Y. Ding, et al., Design and construction of polymerized-chitosan coated Fe3O4 magnetic nanoparticles and its application for hydrophobic drug delivery, Mater. Sci. Eng., C, 2015, 48, 487–498 CrossRef CAS PubMed.
- F. Ye, et al., Uniform mesoporous silica coated iron oxide nanoparticles as a highly efficient, nontoxic MRI T(2) contrast agent with tunable proton relaxivities, Contrast Media Mol. Imaging, 2012, 7(5), 460–468 CrossRef CAS PubMed.
- M. Z. Iqbal, et al., Silica-coated super-paramagnetic iron oxide nanoparticles (SPIONPs): a new type contrast agent of T(1) magnetic resonance imaging (MRI), J. Mater. Chem. B, 2015, 3(26), 5172–5181 RSC.
- T. Ahmad, et al., Gold-coated iron oxide nanoparticles as a T2 contrast agent in magnetic resonance imaging, J. Nanosci. Nanotechnol., 2012, 12(7), 5132–5137 CrossRef CAS PubMed.
- S. E. Sandler, B. Fellows and O. T. Mefford, Best practices for characterization of magnetic nanoparticles for biomedical applications, Anal. Chem., 2019, 91(22), 14159–14169 CrossRef CAS PubMed.
- R. Uebe and D. Schuler, Magnetosome biogenesis in magnetotactic bacteria, Nat. Rev. Microbiol., 2016, 14(10), 621–637 CrossRef CAS PubMed.
- D. E. Goldhawk, et al., Using the magnetosome to model effective gene-based contrast for magnetic resonance imaging, Wiley Interdiscip. Rev. Nanomed. Nanobiotechnol., 2012, 4(4), 378–388 CrossRef CAS PubMed.
- M. A. Shevtsov, et al., Detection of experimental myocardium infarction in rats by MRI using heat shock protein 70 conjugated superparamagnetic iron oxide nanoparticle, Nanomedicine, 2016, 12(3), 611–621 CrossRef CAS PubMed.
- R. Dinali, et al., Iron oxide nanoparticles in modern microbiology and biotechnology, Crit. Rev. Microbiol., 2017, 43(4), 493–507 CrossRef CAS PubMed.
- X. Li, et al., Current investigations into magnetic nanoparticles for biomedical applications, J. Biomed. Mater. Res. A, 2016, 104(5), 1285–1296 CrossRef CAS PubMed.
- Y. Moliner-Martinez, et al., Preconcentration of emerging contaminants in environmental water samples by using silica supported Fe3O4 magnetic nanoparticles for improving mass detection in capillary liquid chromatography, J. Chromatogr. A, 2011, 1218(16), 2276–2283 CrossRef CAS PubMed.
- M. Ahamed, et al., Copper ferrite nanoparticle-induced cytotoxicity and oxidative stress in human breast cancer MCF-7 cells, Colloids Surf., B, 2016, 142, 46–54 CrossRef CAS PubMed.
- J. Park, et al., Ultra-large-scale syntheses of monodisperse nanocrystals, Nat. Mater., 2004, 3(12), 891–895 CrossRef CAS PubMed.
- N. S. Remya, et al., Toxicity, toxicokinetics and biodistribution of dextran stabilized iron oxide nanoparticles for biomedical applications, Int. J. Pharm., 2016, 511(1), 586–598 CrossRef CAS PubMed.
- B. K. Sodipo and A. A. Aziz, One minute synthesis of amino-silane functionalized superparamagnetic iron oxide nanoparticles by sonochemical method, Ultrason. Sonochem., 2018, 40(Pt A), 837–840 CrossRef CAS PubMed.
- W. Li, et al., Free-radical cascade generated by AIPH/Fe3O4-coloaded nanoparticles enhances MRI-guided chemo/thermodynamic hypoxic tumor therapy, ACS Appl. Mater. Interfaces, 2022, 14(26), 29563–29576 CrossRef CAS PubMed.
- Y. Wang, et al., Enhancing targeted cancer treatment by combining hyperthermia and radiotherapy using Mn-Zn ferrite magnetic nanoparticles, ACS Biomater. Sci. Eng., 2020, 6(6), 3550–3562 CrossRef CAS PubMed.
- M. Fathi, et al., Methotrexate-conjugated chitosan-grafted pH- and thermo-responsive magnetic nanoparticles for targeted therapy of ovarian cancer, Int. J. Biol. Macromol., 2020, 154, 1175–1184 CrossRef CAS PubMed.
- M. Mahmoudi and V. Serpooshan, Silver-coated engineered magnetic nanoparticles are promising for the success in the fight against antibacterial resistance threat, ACS Nano, 2012, 6(3), 2656–2664 CrossRef CAS PubMed.
- S. Moraes Silva, et al., Gold coated magnetic nanoparticles: from preparation to surface modification for analytical and biomedical applications, Chem. Commun., 2016, 52(48), 7528–7540 RSC.
- H. Lin, et al., Design of functionalized magnetic silica multi-core composite nanoparticles for synergistic magnetic hyperthermia/radiotherapy in cancer cells, Colloids Surf., B, 2022, 219, 112814 CrossRef CAS PubMed.
- N. Hoshyar, et al., The effect of nanoparticle size on in vivo pharmacokinetics and cellular interaction, Nanomedicine, 2016, 11(6), 673–692 CrossRef CAS PubMed.
- C. Aberg, et al., Sources of variability in nanoparticle uptake by cells, Nanoscale, 2021, 13(41), 17530–17546 RSC.
- I. Lavilla, et al., Elemental fingerprinting of tumorous and adjacent non-tumorous tissues from patients with colorectal cancer using ICP-MS, ICP-OES and chemometric analysis, BioMetals, 2009, 22(6), 863–875 CrossRef CAS PubMed.
- R. Tedja, et al., Effects of serum adsorption on cellular uptake profile and consequent impact of titanium dioxide nanoparticles on human lung cell lines, ACS Nano, 2012, 6(5), 4083–4093 CrossRef CAS PubMed.
- R. Zirbs, et al., Melt-grafting for the synthesis of core-shell nanoparticles with ultra-high dispersant density, Nanoscale, 2015, 7(25), 11216–11225 RSC.
- T. A. Grunewald, et al., Core-shell structure of monodisperse poly(ethylene glycol)-grafted iron oxide nanoparticles studied by small-angle X-ray scattering, Chem. Mater., 2015, 27(13), 4763–4771 CrossRef PubMed.
- J. D. Clogston and A. K. Patri, Zeta potential measurement, Methods Mol. Biol., 2011, 697, 63–70 CrossRef CAS PubMed.
- P. M. Carvalho, et al., Application of light scattering techniques to nanoparticle characterization and development, Front. Chem., 2018, 6, 237 CrossRef PubMed.
- D. Hanahan, Hallmarks of cancer: new dimensions, Cancer Discov., 2022, 12(1), 31–46 CrossRef CAS PubMed.
- G. M. Sulaiman, A. T. Tawfeeq and A. S. Naji, Biosynthesis, characterization of magnetic iron oxide nanoparticles and evaluations of the cytotoxicity and DNA damage of human breast carcinoma cell lines, Artif. Cells Nanomed. Biotechnol., 2018, 46(6), 1215–1229 CrossRef CAS PubMed.
- X. Wang, et al., Fluorescent magnetic PEI-PLGA nanoparticles loaded with paclitaxel for concurrent cell imaging, enhanced apoptosis and autophagy in human brain cancer, Colloids Surf., B, 2018, 172, 708–717 CrossRef CAS PubMed.
- W. S. Yang and B. R. Stockwell, Ferroptosis: death by lipid peroxidation, Trends Cell Biol., 2016, 26(3), 165–176 CrossRef CAS PubMed.
- Y. Zhang, et al., p53 promoted ferroptosis in ovarian cancer cells treated with human serum incubated-superparamagnetic iron oxides, Int. J. Nanomed., 2021, 16, 283–296 CrossRef PubMed.
- M. Salimi, et al., Treatment of breast cancer-bearing BALB/c mice with magnetic hyperthermia using dendrimer functionalized iron-oxide nanoparticles, Nanomaterials, 2020, 10(11), 2310 CrossRef CAS PubMed.
- V. Mulens-Arias, et al., Polyethylenimine-coated superparamagnetic iron oxide nanoparticles impair in vitro and in vivo angiogenesis, Nanomedicine, 2019, 21, 102063 CrossRef CAS PubMed.
- M. Costa da Silva, et al., Iron induces anti-tumor activity in tumor-associated macrophages, Front. Immunol., 2017, 8, 1479 CrossRef PubMed.
- A. Sica, P. Allavena and A. Mantovani, Cancer related inflammation: the macrophage connection, Cancer Lett., 2008, 267(2), 204–215 CrossRef CAS PubMed.
- S. Zanganeh, et al., Iron oxide nanoparticles inhibit tumour growth by inducing pro-inflammatory macrophage polarization in tumour tissues, Nat. Nanotechnol., 2016, 11(11), 986–994 CrossRef CAS PubMed.
- Y. Fang, et al., Magnetism-mediated targeting hyperthermia-immunotherapy in “cold” tumor with CSF1R inhibitor, Theranostics, 2021, 11(14), 6860–6872 CrossRef CAS PubMed.
- P. Das, M. Colombo and D. Prosperi, Recent advances in magnetic fluid hyperthermia for cancer therapy, Colloids Surf., B, 2019, 174, 42–55 CrossRef CAS PubMed.
- C. de la Encarnacion, et al., Hybrid core-shell nanoparticles for cell-specific magnetic separation and photothermal heating, J. Mater. Chem. B, 2023, 11(24), 5574–5585 RSC.
- T. Araya, et al., Antitumor effects of inductive hyperthermia using magnetic ferucarbotran nanoparticles on human lung cancer xenografts in nude mice, Oncol. Targets Ther., 2013, 6, 237–242 CrossRef PubMed.
- E. P. Gusti-Ngurah-Putu, L. Huang and Y. C. Hsu, Effective combined photodynamic therapy with lipid platinum chloride nanoparticles therapies of oral squamous carcinoma tumor inhibition, J. Clin. Med., 2019, 8(12), 2112 CrossRef CAS PubMed.
- M. L. Brongersma, N. J. Halas and P. Nordlander, Plasmon-induced hot carrier science and technology, Nat. Nanotechnol., 2015, 10(1), 25–34 CrossRef CAS PubMed.
- A. J. Nozik, Utilizing hot electrons, Nat. Energy, 2018, 3(3), 170–171 CrossRef.
- J. Xin, et al., Plasmon-enhanced photodynamic therapy for gastric cancer by integrating targeted gold nanorods and photosensitizer, J. Biomed. Nanotechnol., 2022, 18(5), 1302–1315 CrossRef CAS.
- E. C. Cheung and K. H. Vousden, The role of ROS in tumour development and progression, Nat. Rev. Cancer, 2022, 22(5), 280–297 CrossRef CAS PubMed.
- Y. Huang, et al., Superparamagnetic iron oxide nanoparticles induce ferroptosis of human ovarian cancer stem cells by weakening cellular autophagy, J. Biomed. Nanotechnol., 2020, 16(11), 1612–1622 CrossRef CAS PubMed.
- Z. Albakova, et al., HSP70 multi-functionality in cancer, Cells, 2020, 9(3), 587 CrossRef CAS PubMed.
- S. K. Calderwood and A. Asea, Targeting HSP70-induced thermotolerance for design of thermal sensitizers, Int. J. Hyperthermia, 2002, 18(6), 597–608 CrossRef CAS PubMed.
- H. Jiang, et al., Magnetic-manipulated NK cell proliferation and activation enhance immunotherapy of orthotopic liver cancer, J. Am. Chem. Soc., 2023, 145(24), 13147–13160 CrossRef CAS PubMed.
- N. Pothayee, et al., Magnetic nanoclusters with hydrophilic spacing for dual drug delivery and sensitive magnetic resonance imaging, J. Mater. Chem. B, 2013, 1(8), 1142–1149 RSC.
- H. Su, et al., Potential applications and human biosafety of nanomaterials used in nanomedicine, J. Appl. Toxicol., 2018, 38(1), 3–24 CrossRef CAS PubMed.
- M. Ferrari, Cancer nanotechnology: opportunities and challenges, Nat. Rev. Cancer, 2005, 5(3), 161–171 CrossRef CAS PubMed.
- A. Nel, et al., Toxic potential of materials at the nanolevel, Science, 2006, 311(5761), 622–627 CrossRef CAS PubMed.
- J. P. Almeida, et al., In vivo biodistribution of nanoparticles, Nanomedicine, 2011, 6(5), 815–835 CrossRef CAS PubMed.
- A. S. Arbab, et al., Characterization of biophysical and metabolic properties of cells labeled with superparamagnetic iron oxide nanoparticles and transfection agent for cellular MR imaging, Radiology, 2003, 229(3), 838–846 CrossRef PubMed.
- H. S. Choi, et al., Renal clearance of quantum dots, Nat. Biotechnol., 2007, 25(10), 1165–1170 CrossRef CAS PubMed.
- X. Li, et al., Surface chemistry governs the sub-organ transfer, clearance and toxicity of functional gold nanoparticles in the liver and kidney, J. Nanobiotechnol., 2020, 18(1), 45 CrossRef CAS PubMed.
- S. Gangemi, et al., Cold atmospheric plasma targeting hematological malignancies: potentials and problems of clinical translation, Antioxidants, 2022, 11(8), 1592 CrossRef CAS PubMed.
- J. Sun, et al., A hybrid plasma electrocatalytic process for sustainable ammonia production, Energy Environ. Sci., 2021, 14, 865–872 RSC.
- E. Freund, et al., Hypochlorous acid selectively promotes toxicity and the expression of danger signals in human abdominal cancer cells, Oncol. Rep., 2021, 45(5), 71 CrossRef CAS PubMed.
- S. Wenske, et al., Nonenzymatic post-translational modifications in peptides by cold plasma-derived reactive oxygen and nitrogen species, Biointerphases, 2020, 15(6), 061008 CrossRef CAS PubMed.
- G. Pasqual-Melo, et al., The progression of metastatic melanoma augments a pro-oxidative milieu locally but not systemically, Pathol. Res. Pract., 2020, 216(11), 153218 CrossRef CAS PubMed.
- S. Bekeschus, et al., Medical gas plasma jet technology targets murine melanoma in an immunogenic fashion, Adv. Sci., 2020, 7(10), 1903438 CrossRef CAS PubMed.
- T. VON Woedtke, et al., Plasma medicine: a field of applied redox biology, In Vivo, 2019, 33(4), 1011–1026 CrossRef CAS PubMed.
- G. Daeschlein, et al., Skin and wound decontamination of multidrug-resistant bacteria by cold atmospheric plasma coagulation, J. Dtsch. Dermatol. Ges., 2015, 13(2), 143–150 Search PubMed.
- S. Arndt, et al., Cold atmospheric plasma (CAP) activates angiogenesis-related molecules in skin keratinocytes, fibroblasts and endothelial cells and improves wound angiogenesis in an autocrine and paracrine mode, J. Dermatol. Sci., 2018, 89(2), 181–190 CrossRef CAS PubMed.
- C. Duchesne, et al., Cold atmospheric plasma modulates endothelial nitric oxide synthase signalling and enhances burn wound neovascularisation, J. Pathol., 2019, 249(3), 368–380 CrossRef CAS PubMed.
- Y. Nomura, et al., Investigation of blood coagulation effect of nonthermal multigas plasma jet in vitro and in vivo, J. Surg. Res., 2017, 219, 302–309 CrossRef PubMed.
- X. Dai, et al., Cold atmospheric plasmas target breast cancer stemness via modulating AQP3-19Y mediated AQP3-5K and FOXO1 K48-ubiquitination, Int. J. Biol. Sci., 2022, 18(8), 3544–3561 CrossRef CAS PubMed.
- J. Van Loenhout, et al., Cold atmospheric plasma-treated PBS eliminates immunosuppressive pancreatic stellate cells and induces immunogenic cell death of pancreatic cancer cells, Cancers, 2019, 11(10), 1597 CrossRef CAS PubMed.
- K. Panngom, et al., Preferential killing of human lung cancer cell lines with mitochondrial dysfunction by nonthermal dielectric barrier discharge plasma, Cell Death Dis., 2013, 4(5), e642 CrossRef CAS PubMed.
- R. Sensenig, et al., Retraction note to: non-thermal plasma induces apoptosis in melanoma cells via production of intracellular reactive oxygen species, Ann. Biomed. Eng., 2013, 41(3), 656 CrossRef PubMed.
- W. Li, et al., Cold atmospheric plasma and iron oxide-based magnetic nanoparticles for synergetic lung cancer therapy, Free Radic. Biol. Med., 2019, 130, 71–81 CrossRef CAS PubMed.
- J. Canady, et al., The first cold atmospheric plasma phase I clinical trial for the treatment of advanced solid tumors: a novel
treatment arm for cancer, Cancers, 2023, 15(14), 3688 CrossRef PubMed.
- M. Zivanic, et al., Current state of cold atmospheric plasma and cancer-immunity cycle: therapeutic relevance and overcoming clinical limitations using hydrogels, Adv. Sci., 2023, 10(8), e2205803 CrossRef PubMed.
- H. Yu, et al., Paclitaxel-loaded core-shell magnetic nanoparticles and cold atmospheric plasma inhibit non-small cell lung cancer growth, ACS Appl. Mater. Interfaces, 2018, 10(50), 43462–43471 CrossRef CAS PubMed.
- N. D. Almeida, et al., Cold atmospheric plasma as an adjunct to immunotherapy for glioblastoma multiforme, World Neurosurg., 2019, 130, 369–376 CrossRef PubMed.
- H. Wu, et al., Enhanced tumor synergistic therapy by injectable magnetic hydrogel mediated generation of hyperthermia and highly toxic reactive oxygen species, ACS Nano, 2019, 13(12), 14013–14023 CrossRef CAS PubMed.
- W. Ying, et al., Hollow magnetic nanocatalysts drive starvation-chemodynamic-hyperthermia synergistic therapy for tumor, ACS Nano, 2020, 14(8), 9662–9674 CrossRef CAS PubMed.
- M. Chang, et al., Single-atom Pd nanozyme for ferroptosis-boosted mild-temperature photothermal therapy, Angew. Chem., Int. Ed. Engl., 2021, 60(23), 12971–12979 CrossRef CAS PubMed.
- R. R. Khanikar, et al., Cold atmospheric plasma driven self-assembly in serum proteins: insights into the protein aggregation to biomaterials, RSC Adv., 2022, 12(40), 26211–26219 RSC.
- M. Wang, et al., Killing malignant melanoma cells with protoporphyrin IX-loaded polymersome-mediated photodynamic therapy and cold atmospheric plasma, Int. J. Nanomed., 2017, 12, 4117–4127 CrossRef CAS PubMed.
- J. Lin, et al., A review on reactive oxygen species (ROS)-inducing nanoparticles activated by uni- or multi-modal dynamic treatment for oncotherapy, Nanoscale, 2023, 15(28), 11813–11833 RSC.
- J. Tornin, et al., Evaluation of the effects of cold atmospheric plasma and plasma-treated liquids in cancer cell cultures, Nat. Protoc., 2021, 16(6), 2826–2850 CrossRef CAS PubMed.
- L. Bian, et al., Ultramicrotomy preparation of magnetic nanoparticles for transmission electron microscopy, Ultramicroscopy, 2021, 227 Search PubMed.
- M. R. Khataminejad, et al., Antimicrobial effect of imipenem-functionalized Fe2O3 nanoparticles on Pseudomonas aeruginosa producing metallo beta-lactamases, Iran. J. Biotechnol., 2015, 13(4), 43–47 CrossRef PubMed.
- D. Patino-Ruiz, et al., Synthesis of FeO@SiO2-DNA core-shell engineered nanostructures for rapid adsorption of heavy metals in aqueous solutions, RSC Adv., 2020, 10(64), 39284–39294 RSC.
- D. A. Bazylinski and R. B. Frankel, Biologically-controlled mineralization of magnetic iron minerals by magnetotactic bacteria, in Environmental Microbe-Metal Interactions, ed. D. R. Lovley, 2000 Search PubMed.
|
This journal is © The Royal Society of Chemistry 2024 |
Click here to see how this site uses Cookies. View our privacy policy here.