DOI:
10.1039/D4RA03711A
(Review Article)
RSC Adv., 2024,
14, 21832-21858
Nanotechnology in food packaging materials: role and application of nanoparticles
Received
20th May 2024
, Accepted 27th June 2024
First published on 9th July 2024
Abstract
Global concerns about food security, driven by rising demand, have prompted the exploration of nanotechnology as a solution to enhance food supply. This shift comes in response to the limitations of conventional technologies in meeting the ever-increasing demand for food products. Consequently, nanoparticles play a crucial role in enhancing food production, preservation, and extending shelf life by imparting exceptional properties to materials. Nanoparticles and nanostructures with attributes like expansive surface area and antimicrobial efficacy, are versatile in both traditional packaging and integration into biopolymer matrices. These distinctive qualities contribute to their extensive use in various food sector applications. Hence, this review explores the physicochemical properties, functions, and biological aspects of nanoparticles in the context of food packaging. Furthermore, the synergistic effect of nanoparticles with different biopolymers, alongside its different potential applications such as food shelf-life extenders, antimicrobial agents and as nanomaterials for developing smart packaging systems were summarily explored. While the ongoing exploration of this research area is evident, our review highlights the substantial potential of nanomaterials to emerge as a viable choice for food packaging if the challenges regarding toxicity are carefully and effectively modulated.
Introduction
In recent years, nanotechnology has significantly impacted food packaging materials, addressing challenges in both food preservation and environmental considerations.1 Packaging materials are widely acknowledged for their crucial role in protecting food products, preventing degradation from physical, chemical, or biological factors, and ensuring overall quality during storage and handling.2 In this context, the integration of nanotechnology with encapsulation techniques has led to advanced methods for creating innovative food sector devices with potential benefits across various aspects of food science.3
Nanomaterials are attracting attention from researchers for their versatile applications in the food industry. Their morphology, size, and distribution contribute to novel and enhanced properties compared to their bulk counterparts from which they originate.4,5 In this sense, a wide variety of nanoparticles (NPs) derived from metal or inorganic metallic oxide-based nanomaterials, including silver, copper, zinc oxide, and titanium dioxide, along with nanostructured materials such as starch, cellulose, chitosan, and montmorillonite (MMT)6,7 have been widely employed in food packaging materials as they demonstrate enhancements in water and gas resistance, mechanical strength and thermal properties.8 However, it should be noted that this will also depend on the concentration in which they are used. Besides, nanomaterials enhance the properties of packaging materials including durability, flexibility, barrier properties, and optical properties.9 For instance, some nanostructures such as cellulose nanocrystal (CNC) and cellulose nanofiber (CNF) were found to greatly improve tensile strength and water vapor permeability of chitosan and whey protein isolate.9,10 Additionally, nanomaterials are extensively employed as antimicrobials to reduce microbial spoilage of packaged foods. In this sense, nanoparticles including copper nanoparticles and silver nanoparticles improved the antibacterial properties, thermal properties, and antioxidant activity when added into agar-based films and CNC, respectively.11,12 Similarly, nanocellulose and nisin based-hydrogel microparticles reduced the growth of L. monocytogenes.13 Nanomaterials are additionally used in intelligent packaging systems to detect microbial contamination, chemical contaminants, or gases indicative of spoilage or quality deterioration in packaged food products.14 In this context, silver nanoparticles (Ag NPs) were reported to effectively detect the lactic acid in fresh milk when incorporated into cysteine and histidine.15 Likewise, titanium dioxide nanoparticles (TiO2 NPs) were employed to check the freshness of meat, showing promising results in the evaluation of the oxidation activity of xanthine oxidase.16
As previously evidenced, nanotechnology possesses a great potential to improve innovative products and processes in the food sector which could offer an excellent opportunity to replace non-degradable plastic packaging materials. However, although there are many review papers aiming to discuss the application of nanotechnology in food packaging context, review papers particularly centered on the utilization of nanoparticles such as silver, zinc oxide, titanium dioxide, copper oxide, graphene oxide, and carbon nanotubes are hard to find. Due to the previously mentioned necessity, the present paper comprehensively reviews the applications of the previously mentioned nanomaterials as antimicrobial packaging systems and sensors to guarantee food safety as well as extend their shelf life. An attempt has also been made to acknowledge the positive impacts of nanomaterials on the properties of biofilms when incorporated into them.
Food packaging roles and contribution of nanomaterials
Packaging is an important component across diverse industries, finding applications in medicine, pharmaceuticals, food, electronics, etc.17 In food-related applications, packaging plays a vital role in slowing down food deterioration, enhancing food quality and safety and extending shelf life, thus reducing food losses and waste.18 In this regard, packaging acts as a barrier against three main types of external influences. Firstly, it addresses chemical influences by minimizing changes caused by environmental factors like gases, moisture, or light. Secondly, it deals with biological contaminants by creating a barrier against microorganisms (pathogens and spoilage agents) and maintaining conditions that control senescence. Lastly, it addresses physical threats by protecting food from mechanical damage.19
A wide range of materials are employed in packaging preparation and manufacturing. Commonly, polymer-based composite materials, including films and nanofibers,20,21 incorporate a variety of organic, inorganic, and composite nanomaterials to enhance their fundamental properties (Fig. 1).22 For example, it has been recognized that integrating nanomaterials into biopolymers enhances their mechanical and barrier properties as well as their stability under different temperature and moisture conditions.23 Furthermore, this has led to intelligent packaging that can help identify when the food has been exposed to adverse conditions, such as inadequate temperatures or elevated oxygen levels, this helps with information about food packaged quality products.24 Lastly, owing to their remarkably antimicrobial properties, nanomaterials are well-suited for antimicrobial active packaging, therefore prolonging the shelf life of food products and minimizing food loss.25 Metal-based materials like silver nanoparticles, nanoforms of certain metal oxides such as copper oxide nanoparticles, titanium oxide nanoparticles, zinc oxide nanoparticles, and carbon-based materials including carbon nanotubes and graphene oxide are among the nanomaterials that have been added to food packaging as functional additions.26
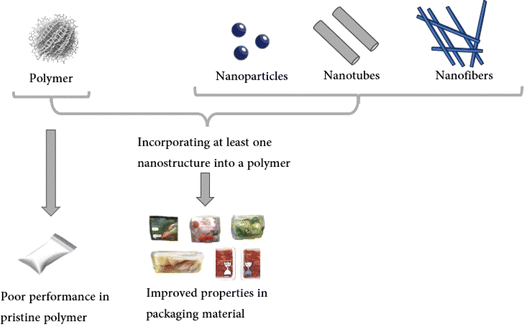 |
| Fig. 1 Schematic representation of the overall procedure for the manufacturing of composites containing nanomaterials and enhancement of the functional properties. | |
Processing techniques
Polymer composites incorporating nanomaterials can be manufactured using processes similar to those employed for conventional polymer composites. This aspect makes them particularly appealing from a production point of view.27 The fabrication method dictates both the concentration and dispersion of the nanofillers and nano reinforcements into the polymer.28
Solution mixing or solvent casting implies vigorously stirring or nanoparticle ultrasonication in a polymer solution before casting it into a mold and subsequently evaporating the solvent. This technique allows for obtaining a homogeneous distribution of nanoparticles within the polymer while facilitating the formation of filler-rich layers.29
At the industrial scale, composite materials on nanoscale are commonly produced through extrusion or melt processing, where polymer and nanofillers are compounded in a single or twin-screw extruder. This process involves blending the components into a molten state, aided by shear and elongational stresses within the mixer. The resulting blend can then be utilized to manufacture a wide range of products such as films through processes like profile injection molding, extrusion, and blow molding.30
Electrospinning and electrospraying involve expelling (electrospinning) or spraying (electrospraying) polymer solutions by applying high electric potential at standard temperature and pressure conditions. Electrospinning provides micro and nanostructured fiber-based materials with diverse morphologies and particles in the range of nanometers to a few micrometers for electrospraying31
Sol–gel technique is widely utilized for producing nano-based coatings. A dense colloidal sol is applied to a surface via dipping, spraying, or spin coating methods. The resulting layers possess a thickness ranging from a few nanometres to 0.5 and 3 μm.32
Effect of the most used nanoparticles in food packaging
Food packaging materials are vital for preserving product quality during distribution and storage. They ideally exhibit appropriate mechanical, optical, and thermal characteristics, acting as barriers against contaminants, water vapor, oxygen, carbon dioxide, flavors, and microorganisms.33 Artificial polymers like polyethylene (PE), polypropylene (PP), polystyrene (PS), polyvinyl chloride (PVC), polyethylene terephthalate (PET), polyvinyl alcohol (PVA), and polyglycolide (PGA) are commonly utilized in food packaging owing to their thermal stability, mechanical properties, lightness, and cost-effectiveness. However, sourced from non-renewable petroleum, these polymers present potential health and environmental hazards.34 Recent research focuses on developing biopolymers for food packaging. Various biodegradable polymers, sourced from plant and animal origins, include polysaccharides (agar, carrageenan, starch, pectin, inulin, chitin, and chitosan), microbial polysaccharides (xanthan, gellan, curdlan, pullulan, dextran, and bacterial cellulose), polyesters, proteins, and synthetic variants (poly(L-lactide) or poly (butylene succinate)). Some are produced through microbial fermentation, such as poly(β-hydroxybutyrate) (PHB).
Unfortunately, natural polymers possess challenges due to their high gas and vapor permeability, coupled with poor resistance, limiting their broad applications.34,35 One potential solution to this issue is the enhancement of their properties such as mechanical strength and gas barrier capabilities. This approach enables their effective application in food packaging while addressing environmental concerns.36 Achieving this objective involves integrating nanomaterials, such as nano-fillers, into biodegradable polymers to form biocomposites. These innovative materials can provide enhanced mechanical strength and act as barriers against microorganisms, moisture, and gases.37
On the other hand, nanoparticles are gaining interest due to their microbial and sensor properties. These particles show promise by directly interacting with bacterial cells, offering potential solutions.38 Furthermore, the integration of nanotechnology with appropriate analytical methodologies and detection techniques proves highly valuable for evaluating the quality and safety of food.39 As an example, nanosensors, made from metals and metal oxides, are integrated into ‘smart packaging’ for food. Enabling real-time monitoring of storage conditions, assessing freshness, providing insights into product quality, and enhancing overall safety. These nanosensors can also be used in measurement devices to evaluate factors influencing food quality and safety.40
Silver nanoparticles (Ag NPs)
To enhance barrier and functional properties of polymers. Several studies suggest that the addition of silver nanoparticles into biopolymers holds promise in food packaging technology by markedly enhancing packaging attributes, such as durability, barrier properties, mechanical strength and extending shelf life by reducing pathogen as summarized in Table 1.41,49 For example, Singh and Sahareen (2017)42 reinforced a cellulosic material with silver nanoparticles, the resulting composite extended shelf life of vegetables by retaining its nutritional content, improving antimicrobial effectiveness, and prolonging freshness by maintaining moisture. In another study, three cellulose-based active papers—chitosan (P–CH), chitosan composite (P–CH–TiO2), and chitosan–Ag/TiO2 composite (P–CH–Ag/TiO2)—showed significant yeast and mold inhibition, with P–CH–Ag/TiO2 being the most effective, maintaining 79.25% inhibition after a 6 months storage period and extending kernel shelf life.43 Similarly, Shankar et al. (2021)44 found that composite films containing chitosan, essential oils, and silver nanoparticles, incorporated with gamma irradiation, significantly extended the shelf life of strawberries stored at 4 °C. This improvement was evident in reduced weight loss and decay levels compared to control treatments. Likewise, in a study by Kavakebi et al. (2021),45 a polyvinyl alcohol coated film with silver nanoparticles significantly extended the shelf life of rainbow trout fillet, eliminating S. aureus and prolonging shelf life up to the 7th day in fish trials. Similarly, Nguyen et al. (2021)46 developed a coating with polyvinyl alcohol/agar/maltodextrin and silver nanoparticles for extending the shelf life of harvested bananas. The applied coating reduced weight loss, acidity loss, pH variations, total soluble solids, and softening during storage. Furthermore, Lee et al. (2019)47 found that adding silver nanoparticles to pullulan/pectin films improved various properties and enhanced antimicrobial activity against foodborne pathogens. Additionally, mango peel-extract and silver nanoparticles enhanced a polylactic acid (PLA) film, improving water and oxygen transmission rates without compromising strength and stability. The film also achieved exceptional anti-oxidation and anti-ultraviolet properties.48
Table 1 Packaging materials with silver nanoparticles in food packaging technologies
Food packaging material |
Main finding |
Ref. |
Cellulosic paper |
The composite film had antimicrobial properties against A. hydrophila. Moreover, the films enhanced shelf life of tomatoes and cabbages |
42 |
Cellulose-based papers coated with chitosan |
The addition of Ag NPs and TiO2 NPs led to an improvement of the water and oxygen barrier properties |
43 |
Chitosan incorporated with essential oils |
The composite film displayed potent antimicrobial activity against E. coli, L. monocytogenes, S. typhimurium and A. niger. Additionally, it extended the shelf life of strawberries by 4 days while maintaining their quality |
44 |
Polyvinyl alcohol |
The silver/PVA composite prolonged the shelf life of salmon fillets up to the 7th day and exhibited antimicrobial properties against S. aureus |
45 |
Polyvinyl alcohol coated with agar and maltodextrin |
The incorporation of Ag NPs improved the antibacterial effectiveness against E. coli and S. aureus while also enhancing the flexibility of the film. Moreover, the composite film extended the shelf life of bananas by 5 days |
46 |
Pullulan blended with pectin |
The composite film exhibited superior physical, mechanical, optical, and barrier characteristics. The incorporation of Ag NPs improved the antimicrobial efficacy against S. typhimurium, E. coli and L. monocytogenes of the film |
47 |
Polylactic acid |
The integration of Ag NPs enhanced the mechanical strength of the film and its ability to act as a barrier against water vapor and oxygen. Additionally, the composite film exhibited potent antibacterial properties, contributing to the extension of the shelf life of strawberries |
48 |
As antibacterial agents and food spoilage detection. Silver nanoparticles also possess a wide spectrum of antimicrobial activity to a large variety of microorganisms.50 Additionally, Ag NPs also have the potential to function as detectors, identifying and responding to changes in environmental conditions, including detecting contaminants, assessing microbial quality, and monitoring gas changes.51,52 Multiple research articles have evaluated the efficacy of Ag NPs integrated into food packaging to inhibit foodborne pathogens as summarized in Table 2. For example, films based on chitosan/silver nanoparticles/gold nanoparticles effectively inhibited the growth of S. aureus, P. aeruginosa, A. niger and C. albicans.53 Furthermore, Patri and co-workers (2018)58 developed a sensor combining silver nanoparticles with a chitosan matrix to modify graphite screen-printed electrodes. The sensor successfully measured nitrite levels in commercial milk powder samples, showing satisfactory agreement with a standard protocol. Another study reinforced pullulan films with Ag NPs and essential oils, showing strong antimicrobial activities against Staphylococcus aureus and Listeria monocytogenes in meat and poultry products.54 Ghaffarlou et al. (2022)55 compared the antimicrobial activity of Ag NPs/pullulan and Au NPs/pullulan composites. The authors observed that Ag NPs/pullulan-based films exhibited superior antimicrobial activity against P. aeruginosa, E. coli, and S. aureus. A starch/polyvinyl alcohol film inhibited the growth of Listeria inocua, Escherichia coli, Aspergillus niger and Penicillium expansum when added silver nanoparticles. Further, Mathew et al. (2019)56 developed composite films using polyvinyl alcohol/clay/silver nanoparticles (Ag NPs) for meat packaging. The films exhibited potent antibacterial activity against S. Typhimurium and S. aureus, along with improved mechanical strength, barrier properties, and water resistance. An activated biochar, polylactic acid and silver nanoparticles-based biosensor were developed using solvent casting and in situ oxidative synthesis. The resulting biosensor demonstrated enhanced sensing capabilities for detecting ammonia (NH3) in a 5–60 ppm range, suggesting its potential applications in the food and agriculture sectors.59 Sodium alginate, polyvinyl alcohol, and silver nanoparticles-based sensor film showed strong reactivity to Hg2+ ions in a pH range of 2.0 to 10. The sensor demonstrated a linear response to Hg2+ ion concentrations from 0.9 ppb to 1200 ppb.60 A biodegradable film based on tragacanth, hydroxypropyl methylcellulose, and beeswax, fortified with silver nanoparticles. exhibited antibacterial properties against B. cereus, S. aureus, S. pneumoniae, S. Typhimurium, E. coli and L. monocytogenes.57 The authors highlighted that the resulting films exhibited a stronger antibacterial effect against Gram-negative bacteria in comparison to Gram-positive bacteria. The differing antimicrobial efficacy of Ag NPs against Gram-positive and Gram-negative bacteria can be explained through the perspective of the structure and thickness of the bacterial cell wall.61 Gram-positive bacteria possess several layers of peptidoglycan, which are thicker, usually ranging from 20 to 80 nm, in contrast to the thinner peptidoglycan layer of Gram-negative bacteria (ranging from 7 to 8 nm). This structural difference makes it challenging for Ag NPs to penetrate the cytoplasmic membrane of Gram-positive bacteria. However, due to the thinner peptidoglycan layer in Gram-negative bacteria, Ag NPs can easily penetrate and cause cell death.62
Table 2 Packaging materials with silver nanoparticles in food packaging technologies
Polymer matrices |
Nanomaterial |
Size of nanomaterial (nm) |
Active against bacterial species |
Possible mechanism of action |
Ref. |
Chitosan |
(a) Ag NPs |
(a) 70 to 100 |
(a), (b) S. aureus, P. aureginosa, A. niger, C. albicans |
Electrostatic attraction between the negatively charged cell membrane of the microorganisms and the positively charged Ag NPs or Au NPs |
53 |
(b) Au NPs |
(b) 20 to 30 |
Pullulan reinforced with essential oils |
(a) Ag NPs |
(a) 100 |
(a), (b) S. aureus, L. monocytogenes, E. coli O157:H7 |
Interference with vital cellular processes |
54 |
(b) ZnO NPs |
(b) 110 |
Disruption of DNA replication |
Oxidative stress through the catalysis of ROS. |
Pullulan |
(a) Ag NPs |
(a), (b) 20 to 50 |
(a), (b) P. aureginosa, E. coli, S. aureus |
— |
55 |
(b) Au NPs |
Polyvinyl alcohol reinforced with MMT |
Ag NPs |
— |
S. typhimurium, S. aureus |
Interaction between positively charged silver ions (Ag+) and negatively charged bacterial cell wall |
56 |
Tragacanth blended with hydroxypropyl methylcellulose and beeswax |
Ag NPs |
— |
B. cereus, S. aureus, S. pneumonia, L. monocytogenes, E. coli, S. typhimorum |
Interaction between positively charged silver ions (Ag+) and negatively charged phosphorous or sulfur groups of macromolecules in cell walls |
57 |
The mechanism of Ag NPs has been well established.50,63–65 It is widely accepted that the positive charge of Ag NPs interacts with the negatively charged phosphorus or sulfur groups found in proteins and nucleic acids through electrostatic forces.66 This interaction has the potential to deform bacterial cell walls and membranes, potentially leading to cell death. On the other hand, Ag NPs can also induce the production of reactive oxygen species (ROS) and free-radical species, such as hydrogen peroxide, superoxide anion, hydroxyl radical, hypochlorous acid, and singlet oxygen. These species can elevate oxidative stress within bacterial cells. Furthermore, they can improve the permeability characteristics of bacterial membranes, leading ultimately to cell death.67 An illustration of reported antimicrobial mechanisms induced by nanoparticles is shown in Fig. 2.
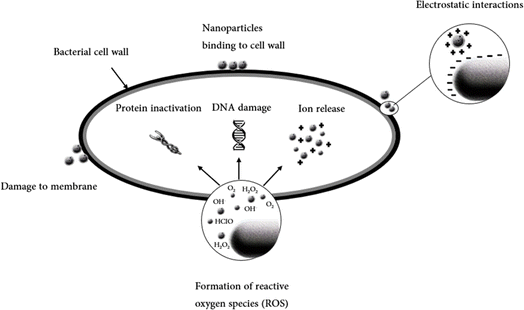 |
| Fig. 2 Schematic of the antimicrobial mechanisms of nanoparticles in food-related applications. | |
Toxicity and safety concerns. According to the regulations set by the European Food Safety Authority (EFSA), the migration of silver from packaging material into foodstuffs should not exceed 0.05 mg kg−1 in food and 0.05 mg L−1 in water.68 As outlined by the United States Environmental Protection Agency, the permissible level of silver in drinking water should not exceed 0.10 mg L−1.69 Although silver nanoparticles can prolong the shelf life of food products, a drawback associated with them is the potential migration of these nanoparticles into the food product, resulting in risks of toxicity.70 Silver nanoparticles may accumulate in various organs of the human body, such as the brain, kidneys, liver, and testicles.71 Moreover, elevated doses of silver nanoparticles can induce neurotoxic, hepatotoxic, and genotoxic effects. However, the possibility of silver migration from packaging systems to food reaching such levels is minimal, although the potential toxicological consequences of silver nanoparticles in food due to migration have not been extensively evaluated.72 For example, in a study conducted by Echegoyen and Nerín (2013),73 the migration of silver was assessed in three types of packaging materials: polypropylene, plastic bags, and polyolefin. The results indicated that the migration of silver fell within the limits set by European regulatory standards. Moreover, Gallocchio et al. (2016)74 conducted a study to assess the migration of silver from a food packaging containing silver nanoparticles (Ag NPs) into chicken meatballs under typical domestic storage conditions. The findings revealed that the migration was gradual. Similarly, Li et al. (2018)75 conducted research on polylactic acid film integrated with titanium dioxide and silver nanoparticles in cottage cheese. Their findings revealed that as storage time increased, the migration of silver also increased, reaching a concentration of 0.02 mg kg−1, which remained well below the standard limits. Cushen et al. (2012)76 noted that the migration rate of silver nanoparticles is influenced by the viscosity of the packaging polymer and the size of the nanoparticles. Typically, migration increases with decreasing particle size and viscosity.
Zinc oxide nanoparticles (ZnO NPs)
To enhance barrier and functional properties of polymers. In the past years, zinc oxide nanoparticles have attracted attention owing to their feasible properties, cost-effectiveness, and essential role in biological systems.77 ZnO nanoparticles possess the potential to improve the properties of packaging materials, like mechanical and barrier properties, and stability.78 Recently, polyvinyl alcohol and gelatin-based films incorporating ZnO and TiO2 NPs doped on 4A zeolite were developed for food packaging applications. The resulting composite doubled the shelf life of shrimp from 6 to 12 days by inhibiting bacterial growth.79 Furthermore, by incorporating ZnO NPs, a PVA and arginine chitosan blend exhibited superior mechanical properties, and water and oxygen barriers.80 Jayakumar et al. (2019)81 developed starch/PVA-based composite films reinforced with ZnO NPs, nutmeg oil, and jamun extract. The inclusion of ZnO NPs improved the antimicrobial features of the film as well as its water and ultraviolet barrier, and mechanical strength. Chitosan blends reinforced with ZnO NPs demonstrated superior properties such as an improvement in its antioxidant properties and bactericidal efficacy against E. coli, S. aureus, B.subtilis, P. aeruginosa82 and L. monocytogenes.83 Further, Priyadarshi et al. (2017)84 enhanced the mechanical properties of chitosan films (77% rise in tensile modulus and 67% increase in tensile strength) by incorporating ZnO NPs into the chitosan matrix. Additionally, polylactic acid reinforced with zinc oxide demonstrated an improvement in the firmness, phenolic content, color retention, and sensory quality of apples.85 Another study incorporated 5% of ZnO NPs into polylactic acid, resulting in a reduced gas permeability as well as an improvement of its tensile properties and antimicrobial activity against E. coli of polylactic acid.86 Examples of films and coatings containing zinc oxide nanoparticles from the available literature are documented in Table 3.
Table 3 Packaging materials with zinc oxide nanoparticles in food packaging technologies
Food packaging material |
Main finding |
Ref. |
Polyvinyl alcohol blended with gelatin |
The addition of ZnO NPs and TiO2 NPs extended the shelf life of shrimp up to 12 days and preserved the sensory characteristics of the films during storage |
79 |
Polyvinyl alcohol |
The incorporation of ZnO NPs improved the antibacterial efficacy against S. aureus and E. coli of the film and extended the shelf life of cherry tomatoes |
80 |
Starch blended with polyvinyl alcohol |
The water and UV barrier and mechanical and antimicrobial properties of starch/PVA film can be improved owing to the incorporation of ZnO NPs |
81 |
Chitosan and cellulose acetate phthalate |
Incorporating ZnO NPs enhanced the thermal stability and barrier properties of the film, extending the shelf life of black grape fruits up to 9 days |
82 |
Pullulan blended with chitosan |
The blend of ZnO NPs and propolis enhanced the mechanical strength of the film by 25%. Additionally, there was a slight increase in the film's water vapor barrier and hydrophobicity |
83 |
Chitosan |
Chitosan film reinforced with ZnO NPs showed an enhancement of 77% and 67% in tensile modulus and tensile strength respectively. Moreover, the enhanced films exhibited a twofold increase in antimicrobial activity against B. subtilis and a 1.5-fold increase against E. coli |
84 |
Polylactic acid |
An improvement in water vapor permeability, elongation at break and elastic modulus of the films was observed. Additionally, the film prolonged the shelf life of fresh-cut apples for 14 days |
85 |
Polylactic acid |
Adding 5 wt% of ZnO NPs enhanced the tensile properties and lowered the permeability to O2 and CO2 of the films. The film extended the shelf life of cherries |
86 |
As antibacterial agents and food spoilage detection. ZnO NPs enhance the antibacterial properties of active coatings, seamlessly integrated into polymer matrices, further, they can also be used in food spoilage detection. For instance, their incorporation into chitosan-based edible films effectively inhibited E. coli growth while improving thermal stability.87 Another report developed a film based on ZnO NPs, chitosan carboxylated multiwalled carbon nanotubes and polyaniline Another report developed a film based on ZnO NPs, chitosan carboxylated multiwalled carbon nanotubes and polyaniline for detecting xanthine in fish meat during storage. The biosensor demonstrated a linear response of 0.1–100 μM.16 Hooda et al. (2018),88 developed a composite strip combining chitosan, coconut fiber, and zinc oxide nanoparticles designed for polyamine sensing. The resulting composite measured polyamine levels in fruits and vegetables within a temperature range of 25 to 33 °C and an incubation time of 6 minutes, it possessed a linearity of 5.0 mM. Furthermore, Hezma et al. (2019),89 compared the effects of incorporating various concentrations of ZnO NPs in polyvinyl alcohol/chitosan blend films for food packaging applications. The authors concluded that the sample containing 10 and 15 wt% ZnO displayed the greatest thermal stability, mechanical strength, and antibacterial activity. Another report revealed that the incorporation of zinc oxide nanoparticles on polylactic acid films enhanced the antibacterial efficacy against E. coli and L. monocytogenes.87 In a parallel report, the antimicrobial potential of trinary bio-composite composed of black cumin cake extract/gelatin/PVA film with ZnO NPs was tested. The resulting composite effectively inhibited the growth of three Gram-positive bacterial strains.90 A smart packaging integrated of chitosan, polyvinyl alcohol and ZnO NPs with Carissa carandas anthocyanin demonstrated improved mechanical strength, reduced moisture, and lowered water vapor permeability. Additionally, the films displayed distinct colour changes which allows the effective monitoring of fish fillet freshness.91 Furthermore, Bajpai et al. (2010)92 investigated the antibacterial activity of zinc oxide nanoparticles loaded on a chitosan-based edible film. The authors found that ZnO nanoparticles demonstrated fair antibacterial action against E. coli. Table 4 summarizes the antibacterial activity of different biopolymer based-materials including ZnO NPs.
Table 4 Antibacterial activity of different biopolymer based-materials containing ZnO NPs
Polymer matrices |
Nanomaterial |
Size of nanomaterial (nm) |
Active against bacterial species |
Possible mechanism of action |
Ref. |
Chitosan blended with PVA |
ZnO NPs |
24.5 |
S. aureus, E. coli, C. albicans, A. niger |
The ROS (OH, O2−, H2O2) and Zn2+ released from ZnO react with bacterial cell wall and intracellular contents of the cell causing damage to nucleic acids and ultimately leading to bacterial death |
89 |
Polylactic acid |
ZnO NPs |
50 to 100 |
E. coli, L. monocytogenes |
Formation of reactive oxygen species |
87 |
Gelatin blended with PVA and reinforced with black cumin cake extract |
ZnO NPs |
<100 |
M. luteus, L. monocytogenes, S. aureus |
Permeation of Zn2+ particles into the cell membrane, causing oxidative stress ultimately disrupting the cellular functions |
90 |
Chitosan |
ZnO NPs |
— |
E. coli |
ZnO releases ROS species which interact with cell membrane causing severe damage and ultimately kill the bacteria |
92 |
The mechanism of action of ZnO NPs can be explained through the inhibition of bacteria by allowing Zn2+ particles to penetrate the cell membrane, inducing oxidative stress. This stress leads to damage to lipids, carbohydrates, proteins, and DNA, ultimately disrupting cellular functions.93
Toxicity and safety concerns. ZnO nanoparticles are used as active materials in food packaging, posing potential risks to consumers upon contact. In vivo studies have shown that these nanoparticles can access organs through various pathways, including ingestion, inhalation, and parenteral routes.94 Hence, the main concern is to corroborate whether there is migration of nanoparticles from the packaging to the food or not. Should such migration occur, the subsequent focus is to investigate the impact of ingesting these nanoparticles within the body, traversing from the mouth to the gastrointestinal tract, through both in vitro and in vivo exposure tests.95 In this context, Emamifar et al. (2010)96 investigated the migration of silver and zinc oxide nanoparticles from low density polyethylene (LDPE) films into orange juice. They found that the silver ion content (0.1 ± 0.003 μg L−1) in orange juice remained below the allowable concentration threshold (10 ppm) even after 112 days. Although zinc ion migration was higher than silver ions (0.68 ± 0.002 μg L−1), the levels remained within acceptable limits since ZnO is recognized as Generally Recognized as Safe (GRAS) for food applications. While these findings offer promise, further studies are imperative. These should include toxicological investigations based on data derived from migration tests to elucidate the potential actions of nanoparticles as well as released ions within the body.
Titanium dioxide nanoparticles (TiO2 NPs)
To enhance barrier and functional properties of polymers. Titanium dioxide nanoparticles are accepted as safe materials by the Food and Drug Administration (FDA), making them significant for applications in the biomedical, food, and cosmetics sectors.97,98 Specifically, in the food packaging sector, the interaction of TiO2 NPs with film matrices enhances mechanical and gas barrier properties, and in some cases, provides a secondary function of ethylene decomposition, contributing to prolonged fruit shelf life after harvest.99–101 For example, in a study, a chitosan-based film demonstrated superior strength and enhanced barrier properties by the incorporation of TiO2 NPs, additionally, it increased the shelf life of tomatoes by delaying ripening.102 Tian et al. (2019)103 developed a chitosan/TiO2 coating film, the resulting film demonstrated its potential to preserve Gingko biloba seeds by inhibiting mildew, delaying senescence, and preventing declines in firmness. In another report, pullulan/carboxymethyl cellulose/TiO2-NPs composites demonstrated superior antimicrobial activities against E. coli and S. aureus. Besides, it effectively prolonged the shelf life of strawberries by reducing weight loss, and maintaining the firmness, titratable acidity, and skin color.104 Polyvinyl alcohol/TiO2 NPs composite film's potential in food packaging was evidenced by extending the shelf life of Macrobrachium rosenbergii by 1–2 days105 and showing an improvement in its tensile strength and Young's modulus.106 Alberton et al. (2014)107 reported the fabrication of PLA/TiO2 produced through melt mixing. Authors concluded that the incorporation of 1 wt% TiO2 increased PLA's tensile strength and Young's modulus, nevertheless, a decrease in the elongation at break was observed (from 3.56% to 3.00%). Similar results were observed by Qu et al. (2019)108 who studied the properties of chitosan–TiO2 and zein–chitosan–TiO2 films. The authors observed that TiO2 could induce a modification of the film matrix, particularly in chitosan–TiO2 films, forming a dense structure that acts as an obstacle to water vapor diffusion through the film.109,110 Examples of packaging materials incorporating titanium dioxide nanoparticles are summarized in Table 5.
Table 5 Packaging materials with titanium dioxide nanoparticles in food packaging technologies
Food packaging material |
Main finding |
Ref. |
Chitosan |
The reinforced film delayed the ripening process of tomatoes and exhibited improved tensile strength, barrier properties and ethylene photodegradation ability |
102 |
Chitosan |
The film with TiO2 and SiO2 NPs improved fruit health, texture firmness, and moisture content while decreasing decay and shrinkage rates |
103 |
Pullulan blended with carboxymethyl cellulose |
Incorporation of TiO2 NPs improved water vapor and UV-visible light barrier properties in the composite films. Furthermore, the films displayed strong activity against E. coli and S. aureus |
104 |
Polyvinyl alcohol |
PVA reinforced with TiO2 NPs exhibited strong antimicrobial activity against Shewanella spp., Pseudomonas putida and Aeromonas hydrophilia and extended the shelf life for 1–2 days of macrobrachium rosenbergii |
105 |
Polyvinyl alcohol |
The incorporation of TiO2 NPs improved the mechanical properties of the film, although elongation at break slightly decreased |
106 |
Polylactic acid |
An improvement in the Young modulus and tensile strength of the films was observed |
107 |
Zein blended with chitosan |
The addition of 0.15%wt TiO2 NPs increased the antibacterial properties of the film. Additionally, composite films showed better mechanical properties, thermal stability and hydrophobic property |
108 |
As antibacterial agents and food spoilage detection. TiO2 nanoparticles are commonly used as additives in food for their non-toxic, photostable, and antibacterial properties. They are extensively studied for their ability to enhance functional properties and inhibit microbial growth in food, making them cost-effective and highly demanded in the field, further, they are integrated into biosensors due to their remarkable stability, photocatalytic performance, and biocompatibility.111,112 Table 6 summarizes the application of TiO2 NPs in improving the antimicrobial properties of different biopolymers. For example, Li et al. (2016)113 observed that chitosan–TiO2 composites exhibited increased antibacterial efficacy against Xanthomonas oryzae pv. Another report found that chitosan/TiO2/Ag NPs composites reduced Escherichia coli by 6 logs after 24 hours and showed a minimum inhibitory concentration (MIC) value of 0.38 mg ml−1 during refrigerated fruit storage.114 A dual-layer antibacterial chromogenic material based on chitosan, hydroxyethyl cellulose, and mulberry anthocyanins, and TiO2 NPs was designed to measure litchi fruit freshness. The resulting material showed antibacterial properties against E. coli and S. aureus and changed colors as the quality of the litchi decreased.118 Another study documented that incorporating TiO2 significantly enhances the antimicrobial properties of PLA films, as seen in inhibition zone results, measuring 4.86 ± 0.50 mm for E. coli and 4.63 ± 0.45 mm for S. aureus.115 Tajdari et al. (2020)116 developed a film based on ZnO NPs/TiO2 NPs/polylactic acid. In comparison to neat PLA, the resulting composite exhibited greater antimicrobial action against L. monocytogenes and E. coli. In another study, the antimicrobial potential of cellulose/PVA/TiO2 NPs-based composite films was tested. The films effectively suppressed the growth of pathogens including Bacillus cereus and Escherichia coli.117 Likewise, Jiang et al. (2023),119 developed a pH-responsive smart film using alginate, polyvinyl alcohol, purple garlic peel extract, and titanium dioxide nanoparticles to visually monitor beef freshness. During the storage of beef, the developed film exhibited noticeable color changes, effectively indicating its freshness (blue) and deterioration (yellow-green). Similarly, a composite film based on polylactic acid/TiO2 NPs/lycopene effectively monitored the oxidative changes of packaged margarine during storage, changing color from dark orange to pale yellow.120 Additionally, the antimicrobial effectiveness of binary bio-composite film based on chitosan and TiO2 NPs was tested. The biocomposite inhibited the growth of different pathogens including E. coli and S. aureus, as well as fungi (Aspergillus and Penicillium). The authors emphasized that the films demonstrated greater efficacy against Gram-positive bacteria compared to Gram-negative bacteria, possibly due to variances in cell wall structure, physiology, metabolism, or surface charge of bacterial cells.102
Table 6 Antibacterial activity of different biopolymer based-materials containing TiO2 NPs
Polymer matrices |
Nanomaterial |
Size of nanomaterial (nm) |
Active against bacterial species |
Possible mechanism of action |
Ref. |
Chitosan |
TiO2 NPs |
21 |
S. aureus, E. coli, S. Typhimurium, Pseudomonas aeruginosa, Aspergillus, Penicillium |
Photocatalytic reaction and generation of ROS under exposure of UV light |
102 |
Chitosan |
TiO2 NPs |
5 |
Xanthomonas oryzae strains |
Release of toxic metal ions |
113 |
Generation of hydroxyl radicals and other ROS under radiation with UV light |
Chitosan |
(a) Ag NPs |
(a), (b) <100 |
E. coli |
— |
114 |
(b) TiO2 NPs |
Polylactic acid |
TiO2 NPs |
10 to 20 |
E. coli, S. aureus |
Generation of hydrogen peroxide, hydroxyl radicals and superoxide anions under UV light that ultimately contacts and kill the microbes |
115 |
Polylactic acid |
(a) ZnO NPs |
(a) 10 to 30 |
(a), (b) L. monocytogenes, E. coli |
Zn2+ release into the medium leads to direct physical attack on bacterial cell walls via electrostatic interactions |
116 |
(b) TiO2 NPs |
(b) 18 |
Cellulose blended with PVA |
TiO2 NPs |
0.32 to 20 |
Bacillus cereus, E. coli |
Electromagnetic attraction between microorganism and metal oxides |
117 |
The possible action mode of TiO2 has been established. It mainly includes the release of toxic metal ions causing damage to cell membranes upon direct contact with nanoparticles. This leads to the inactivation and death of bacteria by oxidizing the polyunsaturated phospholipids within the cell membrane. Additionally, it facilitates the degradation of toxic compounds expelled by bacteria through the generation of hydroxyl radicals (OH) and other reactive oxygen species (ROS) when exposed to UV light. The antimicrobial effectiveness of TiO2 NPs is related to their size as well as their high surface-to-volume ratio.121
Safety and toxicity concerns. Since 2002, the United States Food and Drug Administration (FDA) has formally approved the utilization of titanium dioxide in food additives, with a restriction that the added quantity should not surpass 1% of the total food mass. Similarly, in the European Union (EU), titanium dioxide is authorized as a food additive (listed as E 171) at quantum satis, indicating that no maximum level is specified.122 Nevertheless, it is relevant to ascertain both the occurrence and extent of nanoparticle migration from packaging materials when in contact with food, as well as the repercussions of their ingestion on the gastrointestinal tract and other organs. In this scenario, Lin and colleagues (2014)123 examined the migration of titanium from LDPE–TiO2 nanocomposites into food simulants (acetic acid and ethanol). Their findings revealed that the highest amount of migrated titanium was 12.1 μg kg−1, observed after exposure to 3% acetic acid at 100 °C for 8 hours. In a separate investigation, migration tests of polyvinyl alcohol–chitosan–TiO2 composites revealed that only a minimum quantity of titanium migrated (3.87 × 10−3% for film treated at 200 MPa) into olive oil after 11 hours, with none detected in water, ethanol, or acetic acid.124 Moreover, Li et al. (2018)125 recently assessed the migration of titanium from PLA-based composites, demonstrating that similar to synthetic composites, the migrated nanomaterial amounted to a small quantity within the safe range.
Copper oxide nanoparticles (CuO NPs)
To enhance barrier and functional properties of polymers. Copper oxide nanoparticles are in high demand due to their biological potential. Research on the application of CuO nanoparticles in food packaging is limited but often emphasizes its capacity to modify oxygen and water barrier properties, optical characteristics, and confer antimicrobial effects, including bactericidal activity against both Gram-positive and Gram-negative bacteria, particularly in nonbiodegradable plastics and a few biopolymers.126 Previously, the fabrication of chitosan films together with CuO nanoparticles was reported to effectively enhance the polymer's properties. For example, Kalia et al. (2021)127 found that ZnO and CuO NPs supplementation improved chitosan characteristics by decreasing moisture content, water holding capacity, and solubility of the films. Besides, it extended the shelf life of guava fruits by one week. Another report reinforced chitosan films with montmorillonite/CuO NPs, the resulting composites demonstrated superior mechanical strength and antibacterial properties without affecting film transparency or water solubility.128 Additionally, CuO NPs could be integrated into polyvinyl alcohol-based blends to improve their properties. For example, Youssef et al. (2020),129 developed carboxymethyl cellulose, polyvinyl alcohol and CuO-based composites. The resulting composites effectively extended the shelf life of cheese by reducing bacterial count, slowing moisture loss, and increasing hardness during storage. Likewise, PVA/CuO NPs-based films showed an improvement in their tensile modulus (4.5 GPa) in comparison to neat PVA (0.9 GPa).69 Furthermore, Francis et al. (2022)130 improved water barrier properties of PVA/starch/glycerol (PSG) composites for food packaging by adding CuO and ZnO. The resulting films showed increased hydrophobicity, reducing water absorptivity by 51.49% and solubility by 60% compared to PSG film. Recently, a polylactic acid/zinc oxide/copper oxide composite film was developed to study its potential in the food packaging sector. The resulting composite showed improved antioxidant and antimicrobial properties and could be employed to extend the shelf life of orange juice.131 Table 7 summarizes the main applications of CuO nanoparticles in different biopolymers.
Table 7 Packaging materials with copper oxide nanoparticles in food packaging technologies
Food packaging material |
Main finding |
Ref. |
Chitosan |
Incorporation of CuO and TiO2 NPs improved the antimicrobial potential of composite films. The films exhibited great transparency and better physical characteristics |
127 |
Chitosan |
The incorporation of MMT–CuO NPs increased the tensile strength and elongation at break, but reduced the water vapor permeability and oxygen permeability of the films |
128 |
Carboxymethyl cellulose blended with polyvinyl alcohol |
Incorporating 0.9 wt% CuO NPs improved the water vapor permeability, mechanical properties, and antimicrobial efficacy of the coating material |
129 |
Polyvinyl alcohol blended with starch and glycerol |
The mechanical properties, thermal stability, water resistance, and UV barrier properties of the films were enhanced by incorporating CuO and ZnO nanoparticles |
130 |
Polylactic acid modified with polyaniline |
The antioxidant and antimicrobial characteristics of the film were enhanced due to the addition of CuO and ZnO NPs |
131 |
Polyvinyl alcohol |
The composite film exhibited reinforced mechanical, dielectric properties and antibacterial properties |
132 |
As antibacterial agents and food spoilage detection. CuO NPs are also widely employed in food packaging due to their potent antimicrobial properties, effectively inhibiting the growth of bacteria, viruses, and fungi. For example, Revathi et al. (2019)133 indicated that chitosan/CuO NPs/neem seed biocomposite exhibited remarkably antimicrobial activity against S. aureus. Further, a CuO NPs/cellulose/chitosan nanofibers composite showed remarkably antimicrobial activity against E. coli, P. aeruginosa, and L. monocytogenes.21 Fathi et al. (2022)134 developed biobased films with carboxymethyl chitosan, saffron petal anthocyanin, and copper oxide nanoparticles. The films exhibited potent antimicrobial activity, changed color in different pH environments, and were effective for real-time monitoring of lamb meat freshness. Table 8 summarizes the antimicrobial activity of biopolymer-based materials incorporating CuO NPs.
Table 8 Antimicrobial efficacy of diverse biopolymer-based materials incorporating CuO nanoparticles
Polymer matrices |
Nanomaterial |
Size of nanomaterial (nm) |
Active against bacterial species |
Possible mechanism of action |
Ref. |
Bacterial cellulose and chitosan nanofibers |
CuO NPs |
48 |
E. coli, P. aeruginosa, L. monocytogenes |
Formation of reactive oxygen species |
21 |
Chitosan and neem |
CuO NPs |
22 |
S. aureus, E. coli, K. aerogenes, S. pyogenes |
Adsorption of Cu2+ ions to the surface of the bacterial and damage the cell membrane |
133 |
Carboxymethyl chitosan blended with petal anthocyanin |
CuO NPs |
— |
Staphylococcus aureus, E. coli |
— |
134 |
The antimicrobial activity of CuO NPs is commonly attributed to various mechanisms. Firstly, CuO NPs attach to the cell membrane, interacting with bacterial lipid membranes. This interaction alters membrane permeability, obstructing nutrient intake and ultimately affecting bacterial viability.135 Also, the release of copper ions and the subsequent formation of copper-peptide complexes disrupt the integrity of the bacterial membrane is another mechanism widely accepted.136 Finally, CuO NPs could catalyze the generation of reactive oxygen species (ROS), significantly enhancing their production, and ultimately resulting in cell death.137
Safety and toxicity concerns. The toxicological effects of employing CuO nanoparticles on human health and the environment are primarily dependent on their physicochemical characteristics.138 Until today, there's scarce information related to the toxicity of copper oxide nanoparticles in food-based applications, therefore, given the repercussions of excessive CuO NP utilization, it is imperative to intensify efforts to comprehend their adverse effects, particularly their migration into food products, and to establish regulatory measures aimed at mitigating human exposure to these nanoparticles.
Carbon nanotubes (CNTs)
To enhance barrier and functional properties of polymers. Single-walled and multi-walled carbon nanotubes have been thoroughly examined for their physicochemical, mechanical, and antimicrobial properties, making them potential additives in packaging. Blending them with synthetic or biopolymers enhances flexibility, thermal stability, mechanical strength, and provides effective barriers against gases and moisture. This modification improves food preservation, shielding it from physical, chemical, and biological deterioration and facilitating transportation and storage.139,140 In this context, Wen et al. (2022),141 observed that PVA films with MWCNTs/ZnO had the potential to suppress natural microorganism growth in chicken meat for at least 36 hours under refrigerated storage. Similarly, Khachatryan et al. (2023)142 improved a chitosan/alginate blend by adding carbon nanostructures. The resulting composite demonstrated enhanced mechanical strength, solubility, water absorption, and UV radiation protection with a 14% reduction in solubility. Another report found that polyvinyl alcohol/multiwall carbon nanotubes/ZnO NPs composite films effectively extended the shelf life of stored vegetables by reducing water loss.143 Likewise, the association of carbon nanotubes and PLA blends have demonstrated potential for food packaging applications. For example, Yakduomi et al. (2022)144 enhanced PLA film's barrier properties, mechanical strength (increment of Young's Modulus by 815%), antibacterial and antifungal activities by incorporating polydopamine-wrapped carbon nanotubes and TiO2 NPs. Another study reinforced PLA films with functionalized carbon nanotubes (fMWCNT), revealing a remarkable improvement in Young's Modulus (up to 50%) and a 20% increase in crystallinity, compared to neat PLA.145 Table 9 summarizes the effects of carbon nanotubes on food packaging materials.
Table 9 Packaging materials with carbon nanotubes in food packaging technologies
Food packaging material |
Main finding |
Ref. |
Polyvinyl alcohol |
PVA reinforced with MWCNTs–ZnO exhibited enhanced thermal stability, water vapor transmission rate, hydrophobicity and antibacterial activity |
141 |
Sodium alginate and chitosan |
Mechanical properties of the composite films were improved by the addition of CNTs and GO. |
142 |
PHBV |
Crystallization temperature, crystallinity, storage modulus and dielectric properties of the films were enhanced by the incorporation of CNTs |
143 |
Polylactic acid |
Crystallization rate, barrier, mechanical, antibacterial and antifungal properties of the films were improved by the addition of MWCNTs |
144 |
Polylactic acid |
PLA reinforced with fMWCNTs exhibited better mechanical properties and Young modulus |
145 |
As antibacterial agents and food spoilage detection. Furthermore, CNTs can be integrated into biopolymers improving its antimicrobial properties as illustrated in Table 10.150 For example, Cui et al. (2020)146 demonstrated that a film containing polylactic acid/poly(ε-caprolactone)/carbon nanotubes/cinnamaldehyde exhibited a sustained cinnamaldehyde release and prolonged its antibacterial effects on S. aureus and E. coli for 21 days, surpassing the film without CNTs by 7 days. Further, the antibacterial properties and thermo-mechanical features of polylactic acid are significantly enhanced by the incorporation of Ag NPs and CNTs.147 Additionally, Goodwin et al. (2015)148 explored the influence of CNTs on polyvinyl alcohol. The bacterial viability against P. aureginosa demonstrated a gradual decrease with escalating concentrations of CNTs. In another study, a lignin/polyvinyl alcohol/lignin-decorated thin-walled carbon nanotubes composite films exhibited increased breaking stress and modulus, along with enhanced antimicrobial properties against S. aureus, compared to neat lignin/PVA films.149 Further, Rezaei et al. (2018),151 immobilized amino-linked lysozyme aptamers in a reduced graphene oxide/multiwalled carbon nanotubes/chitosan/synthesized carbon quantum dot-based composite using glutaraldehyde linker. The resulting composite successfully detected lysozyme protein demonstrating high sensitivity, reproducibility, specificity, and rapid response.
Table 10 Antimicrobial effectiveness of diverse biopolymer-based materials containing carbon nanotubes
Polymer matrices |
Nanomaterial |
Size of nanomaterial (nm) |
Active against bacterial species |
Possible mechanism of action |
Ref. |
Polylactic acid reinforced with PCL and CIN |
CNTs |
Diameter: 3–5 |
S. aureus, E. coli |
— |
146 |
Outer diameter: 8–15 |
Length: 0.05 |
Polylactic acid |
(a) Ag NPs |
(a), (b) Diameter: 60 to 100, length: 0.015 |
S. haemolyticus |
— |
147 |
(b) CNTs |
Polyvinyl alcohol |
CNTs |
Diameter: 15 to 5 |
P. aureginosa |
Membrane lipid disruption or protein binding due to CNT-microorganism contact |
148 |
Length: 0.005 to 20 |
Polyvinyl alcohol |
MWCNTs |
Diameter: 7 to 10 |
S. aureus |
Harmonious effect of MWCNTs through penetration cell |
149 |
Length: 0.100 to 0.300 |
Length: 0.100 to 0.300 |
The mechanism of action of CNTs relies on their interaction with microorganisms, which disrupts cellular membranes, metabolic processes, and morphology.152 Research has revealed that the bacteriostatic properties of CNTs arises from their direct contact with microorganisms, causing damage to their cell membranes and subsequent bacterial cell death. Scanning electron microscopy (SEM) observations have revealed morphological alterations in microorganisms following incubation with CNTs, indicative of compromised cellular integrity. The bacteriostatic attributes of CNTs are increasingly acknowledged and attributed to their high surface-to-volume ratio and substantial inner volume.153 Fig. 3 shows the schematic mechanism of antimicrobial activity of some carbon-based nanomaterials such as carbon nanotubes and graphene oxide sheets.
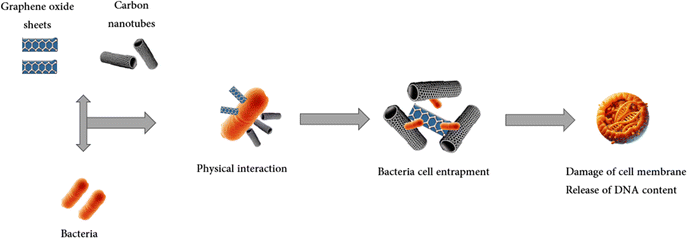 |
| Fig. 3 Mechanism of action of some carbon-based materials. | |
Safety and toxicity concerns. To date, despite the promising properties of CNTs-based composites, their practical use in food packaging remains severely restricted due to significant health concerns. These concerns are mainly related to both the inherent toxicity of CNTs and their potential migration from composite materials.154 Primarily, owing to their unique nanoscale dimensions, CNTs possess the capability to breach biological barriers within the human body, engaging with cells at a molecular level. This interaction can lead to detrimental impacts on various bodily systems, including the lungs, skin, kidneys, and nervous system.155 In this context, Bott et al. (2014)156,157 developed an investigation into the migration behavior of two variants of carbon nanotubes. The assessment involved incorporating these nanomaterials into low-density polyethylene (LDPE) and polystyrene (PS), followed by exposure to various food simulants. These simulants included a 3% acetic acid solution and 95% ethanol, maintained at 60 °C for extended contact periods, as well as an isooctane solution at 40 °C under rapid extraction conditions. Based on both experimental findings and theoretical analysis, it can be concluded that carbon black particles, upon integration into LDPE or PS, do not exhibit migration out of the matrix into food. Once these nanoparticles are fully immobilized, their diffusion remains consistently below the detection limit of any currently available sensitive method. The authors suggest that this conclusion can be extrapolated to other food-contact plastics where carbon black particles are fully embedded. Furthermore, effective reductions in CNT release from their composites have been documented through CNT functionalization158 or irradiation treatment.159 Nevertheless, a comprehensive understanding of the migration characteristics of CNTs is imperative and necessitates extensive studies. Such information is essential to guide their practical applications.
Graphene oxide (GO)
To enhance barrier and functional properties of polymers. Graphene oxide stands out as a noteworthy graphene-based material (GBM), garnering considerable attention owing to its expansive surface area, biocompatibility, as well as its antimicrobial and antioxidant properties.157 Earlier research has demonstrated the effectiveness of graphene oxide in improving preservation capabilities of composites and overall barrier properties, enhancing their physicochemical and biological properties as exemplified in Table 11.166 In this context, F. Han Lyn et al. (2021)157 reinforced chitosan with 2.0% GO and the resulting composite possessed reduced water vapor and oxygen permeability by around 43% and 55%, respectively, demonstrating its potential as an antioxidant material for food packaging. Further, the incorporation of graphene oxide and TiO2 NPs in chitosan films showed a reduction in moisture loss, inhibition of polyphenol oxidase (PPO) activity, additionally, it enhanced antioxidant enzyme activity of the film, especially superoxide dismutase (SOD).160 Vilvert et al. (2022)161 evaluated chitosan/graphene oxide composites on the postharvest preservation of ‘Palmer’ mangoes during cold storage. The resulting composites delayed mango ripening, preserved appearance, firmness and nutritional attributes. Furthermore, the composites decreased weight loss, reduced respiration rate, and minimized both the incidence and severity of anthracnose in mangoes during storage. Other reports evidenced the effects on polyvinyl alcohol-based films upon the addition of graphene oxide materials. For example, Loryuenyong et al. (2015)162 enhanced mechanical and barrier properties of polyvinyl alcohol by incorporating 2.0 wt% GO. Further, bananas wrapped in the resulting composite showed a slower ripening process than those without packaging or packaged in neat PVA. Furthermore, a PVA/Ag NPs/GO/tragacanth gum composite exhibited improved strength, thermal stability, barrier properties and effectively inhibited bacteria growth in raw beef for at least 7 days in refrigerator.163 Additionally, Arfat et al. (2018)164 developed a clove essential oil/graphene oxide nanosheets/polylactide composites for food packaging applications. The study revealed improved antibacterial activity against Staphylococcus aureus and Escherichia coli. Similarly, Kim et al. (2020)165 used graphene oxide and carbon nanotubes (GOCNT), as a co-filler in PLA composite films. A 75% improvement in tensile strength, 140% rise in Young's Modulus and 67% reduction in oxygen transmission was observed in comparison to neat PLA films.
Table 11 Packaging materials with graphene oxide in food packaging technologies
Food packaging material |
Main finding |
Ref. |
Chitosan |
The resulting film exhibited significantly lower vapor permeability oxygen permeability, water solubility and light transmittance |
157 |
Chitosan |
Antimicrobial effects against B. subtilis and A. niger of the film were enhanced by the incorporation of GO and TiO2 NPs |
160 |
Chitosan |
The packaging material slowed down the ripening process of mangoes, resulting in reduced respiration rate, weight loss, and incidence and severity of diseases |
161 |
Polyvinyl alcohol |
The tensile strength, elastic modulus, and failure stain of the films were markedly enhanced by incorporating 0.3 and 2.0 wt% of graphene oxide |
162 |
PVA blended with tragacanth gum |
The resulting films effectively preserved raw beef samples by inhibiting the growth of natural beef bacteria for a minimum of 7 days |
163 |
Polylactide doped with clove EO |
The addition of GO enhanced the optical and anti-UV properties of the films |
164 |
Polylactic acid |
Polylactic acid reinforced with GO–CNTs exhibited a 75% increase in tensile strength and a 130% increase in Young's modulus |
165 |
As antimicrobial agents and food spoilage detection. Additionally, graphene oxide possesses remarkably antimicrobial properties attributed to its extensive surface area and unique thermal, electrical, and physicomechanical properties.167 In this context, several research suggests that the introduction of GO in biopolymers can remarkably enhance its antimicrobial properties as shown in Table 12. As an example, a report suggested that antibacterial activity against E. coli and B. subtilis of chitosan films were higher when graphene oxide was incorporated.168 Further, Khawaja et al. (2018)169 compared the antimicrobial efficacy of different composites containing graphene oxide, chitosan and/or AgNPs. The resulting composites demonstrated notable antibacterial activity against several bacterial strains and the authors concluded that the order of antibacterial effectiveness was GO/CS/Ag > GO/Ag > GO/CS > GO. A highly sensitive humidity-sensing material based on GO/folic acid/chitosan/polyvinyl alcohol was developed by Moustafa et al. (2023).170 The sensor showed rapid response and recovery times (2.6 s/3.5 s), making it promising for quick and accurate humidity detection in intelligent food packaging. Also, the inhibition rate of polylactic acid films against E. coli, B. cereus, S. cerevisiae171 and S. aureus172 have been reported to be increased by the incorporation of GO and ZnO NPs. Dhanasekar et al. (2018)173 highlighted the remarkable bacteriostatic properties of a polyvinyl alcohol film infused with graphene oxide, coupled with either Cu2O or TiO2. This innovative film exhibited significant effectiveness against bacterial strains such as S. aureus, Streptococcus oralis, E. coli, and P. aeruginosa when exposed to UV light. Another report stated that the addition of GO and Ag NPs conferred antibacterial activity against S. aureus and E. coli to polyvinyl alcohol films.174 Usman et al. (2016),175 compared the antimicrobial effectiveness of different composites based on polyvinyl alcohol, graphene oxide, starch and Ag NPs. The authors observed that composite films showed antibacterial activity, with rankings in ascending order: PVA/GO, PVA/Ag, PVA/GO/Ag, and PVA–GO/Ag/Starch. In another report, a dual-purpose sensor based on polylactic acid and graphene oxide successfully detected biogenic amines in food spoilage, providing increased color intensity with analyte concentration and sensitive quantification at low limits of detection (LOD), specifically 0.07 pM for putrescine and 0.02 pM for cadaverine.176
Table 12 Antimicrobial effectiveness of diverse biopolymer-based materials containing graphene oxide
Polymer matrices |
Nanomaterial |
Size of nanomaterial (nm) |
Active against bacterial species |
Possible mechanism of action |
Ref. |
Chitosan |
(a) Graphene oxide |
— |
S. aureus, E. coli |
Active superoxide ions generated on the surface of the oxide reacts with the peptide linkages in the cell wall of bacteria and thus disrupt |
168 |
(b) ZnO NPs |
Chitosan |
(a) ZnO NPs |
— |
E. coli, K. pneumonia, S. aureus, S. mutans, Salmonella, P. aureginosa |
Ions of nanomaterials deactivate cellular enzymes, DNA and proteins by reacting with electron-donating groups interfering with respiratory sequence and leading to cell death |
169 |
(b) Graphene oxide |
Polylactic acid |
(a) ZnO NPs |
(a), (b) 1–5 nm thickness, 4–8 number of layers |
E. coli, B. cereus, S. cerevisiae |
— |
170 |
(b) Graphene oxide |
Polylactic acid |
(a) ZnO NPs |
— |
S. aureus, E. coli |
Inactivation of cell due to direct contact between ZnO and bacteria. Adhesion of GO sheets to the surface cells, covering them and inhibiting cell proliferation |
171 |
(b) Graphene oxide |
Polyvinyl alcohol |
(a) Cu2O NPs |
— |
P. aureginosa, S. aureus, S. oralis, E. coli |
Generation of reactive oxygen species due to light irradiaton |
172 |
(b) Reduced graphene oxide |
(a) TiO2 NPs |
Polyvinyl alcohol |
(a) Ag NPs |
— |
S. aureus, E. coli |
Chemical oxidation based on the oxidation of cellular components by GO sheets |
173 |
(b) Graphene oxide |
Polyvinyl alcohol blended with starch |
(a) Ag NPs |
— |
S. aureus, E. coli |
— |
174 |
(b) Graphene oxide |
(b) Graphene oxide |
The mechanism by which GO nanoparticles inhibit bacterial growth can be explained through (a) physical interactions between the bacterial cell and the nanoparticles. This interaction manifests through direct contact with the basal planes or sharp edges of the graphene material, or through the envelopment of bacteria with nanosheet; (b) physical demolition and chemical oxidation of the cell membrane and cell wall of microorganisms, achieved by the generation of reactive oxygen species (ROS), culminating in microbial death and a subsequent reduction in microbial resistance.173
Safety and toxicity concerns. Graphene and its derivatives exhibit remarkable properties, including excellent dispersibility and stability in human physiological environments, rendering them highly promising for diverse applications. Nevertheless, investigations into the toxicity of graphene derivatives in human biological organisms have yielded inconclusive results. For example, in a study by Yang et al. (2013),177 biodistribution and toxicological analyses of nanographene oxide were conducted in mice through oral and intraperitoneal administration routes. The researchers observed that the graphene derivatives were not adsorbed by the organs but were readily excreted. However, they emphasized that toxicity would be dependent upon factors such as surface coating, size, and routes of administration. Furthermore, in a study by Liu et al. (2012),178 the impact of size and dose on the biodistribution of graphene oxide (GO) in mice was investigated. Their findings indicated that GO particles ranging between 1 and 5 μm accumulated in the lungs, while those sized between 110 and 500 nm accumulated in the liver. Consequently, they concluded that graphene oxide may not be suitable for human use. In contrast, Nguyen et al. (2015)179 demonstrated the low toxicity of graphene oxide against specific intestinal bacteria. Their study revealed that cells remained unaffected across all tested concentrations of graphene oxide for 24 hours, with no observed dose-dependent cytotoxicity. Additionally, Manikandan et al. (2020)180 concluded that PHB/graphene nanocomposites exhibit a negligible cytotoxic effect. Considering these findings collectively, the safety assessment of graphene derivatives remains in its early stages, highlighting the need for further research efforts.
Cellulose nanocrystals
To enhance barrier and functional properties of polymers. Cellulose stands as the most extensively distributed and prevalent polysaccharide in nature181. Nanocellulose refers to cellulose structured at nanoscale dimension.182 This can include cellulose nanofibers (CNF), cellulose nanocrystals (CNC), or bacterial nanocellulose.183 Recently, CNCs have been utilized to reinforce packaging materials, the notable improvements are mainly attributed to the interactions between CNCs and the polymer matrix as summarized in Table 13.190 Different kind of polymers have been used as the matrixes. For example, A. Anžlovar et al. (2018)184 demonstrated that acetic anhydride-modified CNCs can act as reinforcement in linear low-density polyethylene (LLDPE) composites. The authors found an improvement in the compatibility between CNCs and the matrix, resulting in a 90% increase in the breaking strain of the composite. However, the introduction of CNCs influenced the crystallinity of LLDPE, which consequently restricted the reinforcing effect. Similarly, Perumal et al. (2018)185 produced CNCs from rice straw via acid hydrolysis and incorporated them into PVA/chitosan composite films for reinforcement. FTIR analysis indicated significant electrostatic interactions and hydrogen bonding between CNCs and PVA/chitosan, leading to a 130% enhancement in tensile strength. Additionally, the resulting composites also exhibited superior barrier properties. Furthermore, El Achaby et al. (2017)186 formulated a composite film using CNCs, PVA, and carboxymethyl cellulose (CMC) through solvent casting. They observed an 82% increase in tensile strength for the optimized product, coupled with an 81% reduction in water vapor permeability. Remarkably, the film retained approximately 90% optical transparency. Moreover, Yadav et al. (2019)187 demonstrated that CNC into κ-carrageenan/glycerol films reduced water vapor permeability by 52%. Another study found that the addition of 5% of CNC into chitosan-based films enhanced by 26% and 27% in mechanical properties and water vapor permeability, respectively.188 Additionally, a chitosan-CNC composite film was fabricated through covalent linkage, using CNC isolated from eucalyptus wood pulp. The resulting film exhibited a substantial enhancement in mechanical properties (150%).189
Table 13 Packaging materials containing cellulose nanocrystals in food packaging technologies
Food packaging material |
Main finding |
Ref. |
Low density poly(ethylene) |
The addition of acetic anhydride-modified CNCs increased composites' stiffness and strain at break by 20% and up to 90%, respectively. Further, Young modulus increased by ∼45% |
184 |
Polyvinyl alcohol blended with chitosan |
Composite films increased tensile strength (98.16 MPa) and thermal stability |
185 |
Polyvinyl alcohol blended with carboxymethyl cellulose |
The tensile modulus and strength were markedly enhanced (141% and 83%, respectively) by the addition of 5 wt% CNC. Additionally, water vapor permeability was reduced by 87% while composites maintained the same transparency level |
186 |
κ-carrageenan |
Incorporation of CNCs increased the tensile strength and elongation at break of films (from 38.33 ± 3.79 MPa to 52.73 ± 0.70 MPa and from 21.50 ± 3.72% to 28.27 ± 2.39%, respectively). Additionally, water vapor permeability of the films decreased |
187 |
Chitosan |
Improvement of 26% in films' tensile strength was achieved by the addition of 5% (w/w) CNCs. In addition, water vapor permeability of the composite decreased by 27% |
188 |
Chitosan |
Chitosan films reinforced with functionalized cellulose nanocrystals demonstrated a remarkably improvement in tensile strength (up to 150%), while achieving a considerable decrease in hydrophilicity |
189 |
As antimicrobial agents. To date, several semicrystalline polymers, including polycaprolactone, polyamide, and polylactic acid, have been reinforced through the addition of CNC. However, CNC lacks intrinsic antibacterial properties, restricting its application in functional packaging. Furthermore, most studies have predominantly investigated the mechanical behavior of CNC in polymer matrices, with limited research on modifying the functional properties of CNC composites. In this context, Meng et al. (2023)191 enhanced the antibacterial properties of polyvinyl alcohol by incorporating quaternized cellulose nanocrystals as nanofillers. The composite film achieved 100% bactericidal efficiency against E. coli and S. aureus. Moreover, Huang et al. (2023)192 used methacrylamide, cetyltrimethylammonium bromide, and zinc oxide to modify CNCs, and investigated their effect in polylactic acid films. S. aureus and E. coli were effectively inhibited, and the shelf life of packaged pork samples was effectively extended from 3 days to 10 days. Likewise, Leite et al. (2020)193 explored the antimicrobial activity of gelatin films incorporating rosin-grafted cellulose nanocrystals. Authors highlighted that E. coli and S. aureus growth were effectively inhibited by using this composite. Additionally, Costa et al. (2021)194 developed chitosan/cellulose nanocrystals films via solvent casting method. The films were designed as active pads in order to extend the shelf life and maintain the quality of meat. Overall results showed and effective supress effect on the growth of Pseudomonas and Enterobacteriaceae bacteria during the initial storage period. In another study, a remarkably inhibitory effect against L. monocytogenes was observed in corn starch/chitosan-based films when a concentration of 0.5% CNCs was added.195 The various applications of cellulose nanocrystals as antimicrobial agents in different polymers are summarized in Table 14.
Table 14 Antimicrobial effectiveness of diverse biopolymer-based materials containing cellulose nanocrystals
Polymer matrices |
Nanomaterial |
Size of nanomaterial (nm) |
Active against bacterial species |
Possible mechanism of action |
Ref. |
Polyvinyl alcohol |
Quaternized cellulose nanocrystals |
150 ± 20 nm length |
S. aureus, E. coli |
— |
191 |
6 ± 1 nm width |
Polylactic acid |
Modified CNCs |
— |
S. aureus, E. coli |
— |
192 |
Gelatin |
Rosing-grafted CNCs |
— |
S. aureus, E. coli |
Increased permeability and leakage of cytoplasm due to the interaction of rosing grafted CNCs with the phospholipid cell membrane |
193 |
Chitosan |
CNCs |
∼75 nm diameter |
S. aureus, E. coli, C. albicans |
Cell membrane damage due to direct contact with rodlike CNCs particles making microbial cells susceptible to protonated chitosan |
194 |
Corn starch mixed with chitosan |
CNCs |
— |
L. monocytogenes, S. aureus |
Damage to the structural integrity of the cell membrane due to the interaction of nanoparticles and bacteria via electrostatic forces |
195 |
Safety and toxicity concerns. Manufacturing of CNCs offers excellent properties that make them promise for food-related applications. However, being a nanoscale derivative, CNCs still possess unknown properties that could potentially expose humans and the environment to unforeseen risks.190 Recent evaluations have focused on the health and safety aspects of CNCs. It was suggested that studying the gastrointestinal processing of CNCs was important to assess the associated safety concerns. In this context, Koshani and Madadlou (2018),196 highlighted that CNCs are indigestible and could interact with the gut microbiome in the distal ileum and colon. These interactions might influence microbiome metabolism, though the implications of these effects remain uncertain in terms of whether they are beneficial or harmful. Likewise, Ni et al. (2012)197 evaluated the cytotoxicity of cellulose nanowhiskers using L929, while Dong et al. (2012)198 assessed cytotoxicity with nine different cell lines of CNC. Both studies concluded that CNCs demonstrated low cytotoxicity potential. In recent years, cellulose has been classified as a GRAS substance by the FDA. However, as of now, there is no common regulation for nanocellulose, particularly for CNC, in the EU. In 2018, the European Food Safety Authority (EFSA) published guidance on assessing the safety of nanoscience and nanotechnology applications.199 This guidance outlines strategies for characterizing risks and analysing uncertainties, aiming to offer suggestions for future CNC research.
Lipid nanoparticles
To enhance barrier and functional properties of polymers. The concept of lipid nanoparticles is related to utilizing lipids derived from various sources such as animal and vegetable fats, waxes, fatty acids, and acylglycerols, engineered into nanoscale dimensions.200 Recent studies have demonstrated that lipid nanostructured materials possess several beneficial properties that can be effectively used to enhance the properties of food packaging. In this context, research findings indicate that the incorporation of solid-lipid nanoparticles (SLNs) into xanthan gum remarkably improves several properties such as mechanical strength, thermal stability and water vapor permeability of the films.201 Similarly, M. L. Zambrano-Zaragoza et al. (2013)202 developed an edible coating by incorporating SLNs into xanthan gum in order to extend shelf life of guava fruits. Overall results showed a reduced weight loss, lower respiration rates and minimal change in greenish color of guava fruits when stored under refrigeration conditions for 30 days. Moreover, addition of candeuba wax SLNs into xanthan gum enhanced the mechanical strength of films by improving tensile strength, elongation and elastic modulus. The resulting system also extended the shelf life of tomatoes (12 °C for 26 days) by maintaining its firmness and controlling parameters such as pH, acidity, soluble solids, colour changes and antioxidant properties.203 Moreover, incorporating solid-lipid nanoparticles (SLNs) into protein (β-lactoglobulin)-based edible films demonstrated a significant reduction in water vapor permeability while enabling control over mass transfer.204 Similar results were obtained when beeswax-based solid-lipid nanoparticles were incorporated into xanthan gum for food packaging applications. The developed coating effectively reduced weight loss and decaying rate, preserved firmness and minimized colour changes, which in overall aided in enhancing the shelf life of strawberries during refrigerated storage at 4 °C for up to 21 days.205 The diverse applications of nanostructured lipid in food packaging systems are summarized in Table 15.
Table 15 Packaging materials containing lipid nanoparticles in food packaging technologies
Food packaging material |
Main finding |
Ref. |
Xanthan gum |
Improvement of flexibility and strength of the films was achieved by the addition of 60–75 g L−1 solid lipid nanoparticles |
201 |
Xanthan gum |
Addition of solid lipid nanoparticles improved shelf life of guava fruit by showing lowest range of weight loss and delaying the maturation process |
202 |
Xanthan gum |
Composite films exhibited improved barrier properties |
203 |
Proteins |
Incorporation of solid lipid nanoparticles led to a remarkably decrease in water vapor permeability of the film |
204 |
Xanthan gum mixed with propylene glycol |
The composite films exhibited appropriated properties (decreased decay rates, less fungal growth and weight loss) in order to enhance strawberries' shelf life |
205 |
As antimicrobial agents. Various lipid-based nanostructures including liposomes, solid lipid nanoparticles, nanostructured lipid carriers, and nanoemulsions have been acknowledged for their role in enhancing the preservation of food packaging by improving the antimicrobial activity and acting as effective carriers for releasing natural antimicrobial agents.111 The various applications of lipid nanoparticles to different food packaging systems are summarized in Table 16. For example, an agar-based edible film incorporating nanoliposomes loaded with medicinal plant extract (Artemisia annua oil) and chitosan can effectively preserve cherry tomatoes by inhibiting E. coli O157:H7.206 Furthermore, nanoemulsion-based edible coating loaded with citrus essential oil and chitosan nanoparticles was developed for seafood packaging purposes. The resulting system effectively extended the shelf life of silvery pomfret from 12 to 16 days by preventing lipid oxidation and inhibiting microorganisms' growth.207 Similarly, L. Salvia-Trujillo et al. (2015)208 developed a sodium alginate-based antimicrobial edible coating incorporating lemongrass essential oil nanoemulsion in order to preserve fresh-cut apples. The system effectively inhibits the growth of Escherichia coli, while maintaining firmness, reducing respiration rate and browning, and improving overall quality attributes. Similarly, the incorporation of oleic acid nanoemulsion (OAN) loaded with natural antimicrobials (lactic acid, nisin and lauric alginate) into starch-based coatings significantly inhibited Brochothrix thermosphacta, Listeria monocytogenes Scott A, and Micrococcus luteus. Additionally, the OAN offered stable suspension and high optical clarity in the coating.209
Table 16 Applications of lipid-based nanostructures in food packaging systems
Types of nanolipid |
Desing of packaging material content |
Types of packaging |
Food products |
Properties |
Ref. |
Nanoliposome |
Agar/Artemisia annua oil/chitosan |
Edible film |
Cherry tomato |
Cellular leakage and alterations in cell membrane permeability resulting from the release of large molecular substances |
206 |
Nanoemulsion |
Citrus essential oil/chitosan nanoparticles |
Edible coating |
Silvery pomfret |
Inhibited microbial proliferation and decreased oxidation of proteins and lipids |
207 |
Nanoemuslion |
Sodium alginate/lemongrass essential oil/tween 80 |
Edible coating |
Fresh-cut apple |
Extended the shelf life of apples by exhibiting antimicrobial properties, lowering respiration rates, and decreasing ethylene production |
208 |
Nanoemulsion |
Starch/oleic acid/lactic acid/nisin/lauric alginate |
Edible coating |
Fresh-cut pineapple |
Suppresses microbial growth while reducing the respiration rate and mitigating changes in color |
209 |
Safety and toxicity concerns. The widespread adoption of lipid nanoparticles for food-related applications faces limitations due to insufficient understanding and undisclosed properties regarding safety. There are concerns about potential toxicity related to prolonged storage times, which could adversely impact human health. Furthermore, the potential toxicity of these nanoparticles depends on factors such as particle size, bioavailability, biocompatibility, and the amount used. Therefore, the incorporation of lipid nanoparticles into food systems should adhere to specified limits as described by standard bodies or regulatory agencies. Metal and inorganic nanoparticles are non-digestible and can potentially be absorbed directly into epithelial cells, where they may accumulate, be metabolized, or transported through the bloodstream. If these particles are transported from epithelial cells, they can circulate throughout the body, leading to metabolic reactions, excretion, and potential accumulation in specific tissues. However, unlike metal and inorganic NPs, lipid nanoparticles have not been observed to undergo direct absorption in the gastrointestinal tract to date.210 However, there is a possibility of encountering similar occurrences when non-digestible oils are employed for encapsulating bioactives in the production of lipid nanoparticles. Additionally, particle aggregation may occur due to factors such as size, shape, charge, composition, and interfacial chemistry. The presence of lipid nanoparticles in the mouth, stomach, and small intestine could disrupt normal gastrointestinal tract functions due to their smaller size, extensive surface area, and elevated surface energy. This could potentially lead to toxicity concerns, as their behaviour differs significantly from that of microlipids.211,212 Furthermore, the inclusion of organic solvents and certain components such as surfactants could pose potential toxicity risks, as these substances are necessary during the production of nanostructured lipids. However, most of the organic solvent typically evaporates during drying, although minimum amounts may remain in the final product. Therefore, it is important to understand the potential toxic effects of the chemicals employed in nanostructured lipids fabrication before their application. Nevertheless, based on the aforementioned discussion, further research on different forms of nanolipids is required to comprehensively explore all aspects of these nanomaterials and their potential impact on human health when consumed through food and beverages.
Regulations of nanomaterials in food packaging
Guidelines from regulatory bodies offer secure routes for manufacturers, importers, and consumers to safeguard food products' safety in the market.213 However, globally, the absence of established regulations for nanotechnology applications persists due to the scarcity of comprehensive and dependable fundamental research concerning the safety assessment and migration properties of nanomaterials from packaging into the food system.24 In certain countries, nanomaterials are permitted for use in Food Contact Materials (FCMs), whereas in others, their usage is prohibited due to concerns regarding potential toxicity as illustrated in Table 17.214 The Food and Drug Administration (FDA) oversees the use of nanomaterials in food packaging within the United States. Manufacturers of nanomaterials must secure pre-market approval from the FDA, either through a Food Additive Petition (FAP) or the Food Contact Notification (FCN) system. However, substances deemed Generally Recognized as Safe (GRAS) are exempt from this premarket authorization requirement. Additionally, if an organization publishes a scientific risk assessment of its product in a peer-reviewed scientific journal, it may market the product without prior FDA approval.219 The regulation of packaging materials including nanomaterials in Europe falls under the review of the European Union, governed by regulation EC 1935/2004. This regulation permits their use in food packaging as long as they do not pose a risk to human health, as outlined in Article 3. Furthermore, according to Article 23 of regulation EC 10/2011, packages containing nanoparticles must undergo evaluation before entering the market, in accordance with the Novel Food Regulation EC 258/97. Additionally, the regulation of nanomaterials, even if previously authorized in bulk form, is necessary under EEC 89/109. In cases where non-authorized substances are utilized, a maximum migration limit of 0.01 mg kg−1 must be ensured through a functional barrier, as specified in Article 14, EC 450/2009.220
Table 17 Applications and regulations of selected nanomaterials used in food packaging
Nanomaterial |
Applications |
Current status |
Used in |
Commercially available products |
Note |
Ref. |
FCS: Effective Food Contact Substance (FCS) Notifications. GRAS: Generally Recognized as Safe. |
Ag |
Utilized as preservatives, antimicrobials, antibiotics, and antistatic agents |
FCS inventorya |
Reusable food containers |
Nano-silver baby milk bottle |
FCN no. 1235. (<4 ppm by weight of silver as an antimicrobial agent blended into polymers) |
214 and 215 |
Silver-nano noble one-touch mug cup |
Fresh food containers (OSO Fresh®) |
Nano-silver NS-315 water bottle |
Nano-silver salad bowl |
FresherLonger™ Miracle Food Storage |
FresherLonger™ Plastic Storage Bags |
TiO2 |
It is utilized as a color additive |
Not subject to certification |
|
|
Less than 1% by weight of the food |
216 |
ZnO |
Utilized as an additive for UV filtering, antimicrobial purposes, and as a fungistatic agent |
GRAb |
Plastic glasses, plastic films |
Nano Plastic Wrap (SongSing Nano Technology Co., Ltd. Taiwan) |
Authorized under EC regulation 10/2011 (based on conventional particle size) |
217 |
CuO |
Used as a nutritional dietary supplement |
|
|
|
Approved for use in animal feed |
CNT and GO sheets |
Food packaging |
|
Films, bottles for packaging |
|
There is no conclusive information available for the U.S at this time |
218 |
Limitations and challenges of nanomaterials in food packaging
Numerous challenges must be addressed before nanotechnology can revolutionize product development and processes in food-related applications. The main challenge is the creation of edible delivery systems through cost-effective processing techniques, ensuring formulations that are both effective and safe for human consumption.221 Ensuring food safety involves addressing concerns about nanoparticles leaching and migrating from packaging materials into food products. Nanomaterials, whether added directly or indirectly, can sometimes be isolated due to migration from other sources.222 At the nanoscale, materials exhibit distinct behaviors, and our current understanding of analyzing these phenomena remains limited. A comprehensive understanding of the functional aspects and toxicity of nanomaterials at the nanoscale will significantly enhance the practical applications and safety standards of nanotechnology. The potential risks, toxicity issues, and environmental concerns associated with nanoparticles must be acknowledged. Nanoparticles can pass the biological barriers and permeate various tissues and organs. Moreover, the synthesis of nanoparticles through diverse chemical methods can produce harmful non-eco-friendly by-products that lead to significant environmental pollution.223 Hence, it is relevant to establish a comprehensive risk assessment program, regulatory frameworks, and biosecurity measures, and address public concerns before manufacturing, packaging, and consuming nano-based food products. Additionally, both in vitro and in vivo studies on nanoparticle interactions with living organisms are essential before commercial application, especially in the production of antibacterial nanoparticles that are environmentally friendly.224
The trend of research in food packaging
The evolution of food packaging has been driven by continual adaptations to meet the evolving demands of the food industry. Today, the significance of food packaging rivals that of the contents it safeguards, primarily by extending shelf life and reducing waste during transportation, storage, and distribution. However, the growing demand for packaging, predominantly produced from materials that pose potential environmental hazards, underscores a critical opportunity for research into eco-friendly alternatives.
Transitioning to environmentally sustainable packaging materials presents challenges, foremost among them being the need to meet current industry standards and demands. Key considerations include economic viability, ecological sustainability, and the ability to uphold essential physical and chemical properties that prolong product freshness and quality. Moreover, modern packaging should incorporate interactive and intelligent features to inform consumers about product freshness and quality, while also being aesthetically pleasing and potentially offering added consumer benefits through active ingredients.
In response to these demands, recent studies in nanotechnology have focused on developing composite materials based on biopolymers. These materials are enhanced with nanoparticles and micro/nanostructures in precise combinations to impart the requisite physicochemical and biological properties demanded by the food industry. Such research represents a fraction of ongoing efforts aimed at transitioning towards advanced, environmentally friendly packaging solutions.
Conclusions
The increasing focus on health, nutrition, and food safety, along with growing global food demand, has fueled interest in exploring nanotechnology for enhancing food quantity and quality. Metal nanoparticles like silver (Ag), zinc oxide (ZnO), titanium dioxide (TiO2), copper oxide (CuO), and nanomaterials such as carbon nanotubes and graphene oxide are widely used in food preservation. Integrating them into various polymer matrices, including biopolymers, enhances properties and functionality, making nanotechnology invaluable in the food industry. Hence, incorporating metal-based nanoparticles into functional food packaging enhances biological properties, including antioxidant and antimicrobial activities. It also improves the mechanical, physical, barrier, and optical characteristics of the material, ensuring food safety, preserving nutritional value, and extending shelf life. Furthermore, nanotechnology enables real-time monitoring during production, allowing for the development of smart packaging systems for continuous monitoring of food quality and safety throughout the supply chain. Indeed, while nanomaterials hold promise in the food sector, it's essential to assess both their benefits and risks. Addressing biosafety concerns and establishing regulatory frameworks present challenges that require thorough research. Ensuring safety and regulatory compliance is important for the successful integration of these materials into the food packaging industry.
Data availability
This study constitutes a scientific review and does not include original experimental data or other unpublished information. The images included in this manuscript are original creations, while the tables were compiled from cited references. Consequently, this manuscript does not contain supplementary electronic materials.
Author contributions
Conceptualization: R. Herrera-Rivera; S. P. Torres-Arellanes; L. Hernández-Sánchez; R. Román-Doval. Formal analysis: L. Hernández-Sánchez; F. Solis-Pomar; E. Pérez-Tijerina, Diana C. Navarro-Ibarra. Project administration: R. Herrera-Rivera; L. Hernández-Sánchez; F. Solis-Pomar; E. Pérez-Tijerina. Writing – original draft: R. Herrera-Rivera; S. P. Torres-Arellanes; L. Hernández-Sánchez; F. Solis-Pomar; E. Pérez-Tijerina. Writing – review & editing: C. I. Cortés-Martínez; Diana C. Navarro-Ibarra, R. Román-Doval.
Conflicts of interest
The authors declare no competing interests.
References
- R. Singh, S. Dutt, P. Sharma, A. K. Sundramoorthy, A. Dubey, A. Singh and S. Arya, Future of Nanotechnology in Food Industry: Challenges in Processing, Packaging, and Food Safety, Global chall., 2023, 7, 2200209 CrossRef PubMed
. - A. M. Youssef, S. M. El-Sayed, H. H. Salama, H. S. El-Sayed and A. Dufresne, Evaluation of bionanocomposites as packaging material on properties of soft white cheese during storage period, Carbohydr. Polym., 2015, 132, 274–285 CrossRef CAS PubMed
. - A. V. Singh, Z. Hosseinidoust, B.-W. Park, O. Yasa and M. Sitti, Microemulsion-Based Soft Bacteria-Driven Microswimmers for Active Cargo Delivery, ACS Nano, 2017, 11(10), 9759–9769 CrossRef CAS PubMed
. - M. A. Abdel-Wahhab and F. Márquez, Nanomaterials in Biomedicine, Soft Nanosci. Lett., 2015, 05(03), 53–54 CrossRef
. - S. Hassan and A. V. Singh, Biophysicochemical Perspective of Nanoparticle Compatibility: A Critically Ignored Parameter in Nanomedicine, J. Nanosci. Nanotechnol., 2014, 14(1), 402–414 CrossRef CAS PubMed
. - K. Pathakoti, M. Manubolu and H.-M. Hwang, Nanostructures: Current uses and future applications in food science, J. Food Drug Anal., 2017, 25(2), 245–253 CrossRef CAS PubMed
. - A. Orsuwan and R. Sothornvit, Polysaccharide Nanobased Packaging Materials for Food Application, in Food Packaging and Preservation, Elsevier, 2018, pp. 239–270 Search PubMed
. - S. Shankar and J.-W. Rhim, Bionanocomposite Films for Food Packaging Applications, in Reference Module in Food Science, Elsevier, 2018 Search PubMed
. - Y. Xu, S. Willis, K. Jordan and E. Sismour, Chitosan nanocomposite films incorporating cellulose nanocrystals and grape pomace extracts, Packag. Technol. Sci., 2018, 31(9), 631–638 CrossRef CAS
. - R. A. Carvalho, T. A. Santos, V. M. de Azevedo, P. H. C. Felix, M. V. Dias and S. V. Borges, Bio-nanocomposites for food packaging applications: effect of cellulose nanofibers on morphological, mechanical, optical and barrier properties, Polym. Int., 2018, 67(4), 386–392 CrossRef CAS
. - S. Shankar, X. Teng and J.-W. Rhim, Properties and characterization of agar/CuNP bionanocomposite films prepared with different copper salts and reducing agents, Carbohydr. Polym., 2014, 114, 484–492 CrossRef CAS PubMed
. - M. Salari, M. Sowti Khiabani, R. Rezaei Mokarram, B. Ghanbarzadeh and H. Samadi Kafil, Development and evaluation of chitosan based active nanocomposite films containing bacterial cellulose nanocrystals and silver nanoparticles, Food Hydrocolloids, 2018, 84, 414–423 CrossRef CAS
. - P. Lu, et al., Nanocellulose/Nisin Hydrogel Microparticles as Sustained Antimicrobial Coatings for Paper Packaging, ACS Appl. Polym. Mater., 2022, 4(4), 2664–2673 CrossRef CAS
. - K. D. Grieger, J. Harrington and N. Mortensen, Prioritizing research needs for analytical techniques suited for engineered nanomaterials in food, Trends Food Sci. Technol., 2016, 50, 219–229 CrossRef CAS
. - A. A. Madhavan, R. Qotainy and R. Nair, Synthesis of functionalized silver nanoparticles and its application as chemical sensor, in 2019 Advances in Science and Engineering Technology International Conferences (ASET), IEEE, 2019, pp. 1–4 Search PubMed
. - R. Devi, S. Yadav and C. S. Pundir, Amperometric determination of xanthine in fish meat by zinc oxide nanoparticle/chitosan/multiwalled carbon nanotube/polyaniline composite film bound xanthine oxidase, Analyst, 2012, 137(3), 754–759 RSC
. - B. Tajeddin and M. Arabkhedri, Polymers and food packaging, in Polymer Science and Innovative Applications, Elsevier, 2020, pp. 525–543 Search PubMed
. - J. Han, L. Ruiz-Garcia, J. Qian and X. Yang, Food Packaging: A Comprehensive Review and Future Trends, Compr. Rev. Food Sci. Food Saf., 2018, 17(4), 860–877 CrossRef PubMed
. - K. Marsh and B. Bugusu, Food Packaging—Roles, Materials, and Environmental Issues, J. Food Sci., 2007, 72(3), 39–55 CrossRef PubMed
. - M. Hoseinnejad, S. M. Jafari and I. Katouzian, Inorganic and metal nanoparticles and their antimicrobial activity in food packaging applications, Crit. Rev. Microbiol., 2018, 44(2), 161–181 CrossRef CAS PubMed
. - H. Almasi, P. Jafarzadeh and L. Mehryar, Fabrication of novel nanohybrids by impregnation of CuO nanoparticles into bacterial cellulose and chitosan nanofibers: Characterization, antimicrobial and release properties, Carbohydr. Polym., 2018, 186, 273–281 CrossRef CAS PubMed
. - J. O. Adeyemi and O. A. Fawole, Metal-Based Nanoparticles in Food Packaging and Coating Technologies: A Review, Biomolecules, 2023, 13(7), 1092 CrossRef CAS PubMed
. - R. Becerril, C. Nerín and F. Silva, Encapsulation Systems for Antimicrobial Food Packaging Components: An Update, Molecules, 2020, 25(5), 1134 CrossRef CAS PubMed
. - Y. Huang, L. Mei, X. Chen and Q. Wang, Recent Developments in Food Packaging Based on Nanomaterials, Nanomaterials, 2018, 8(10), 830 CrossRef PubMed
. - S. Barage, et al., Nanomaterial in Food Packaging: A Comprehensive Review, J. Nanomater., 2022, 2022, 1–12 CrossRef
. - S. Jafarzadeh, A. Salehabadi and S. M. Jafari, Metal nanoparticles as antimicrobial agents in food packaging, in Handbook of Food Nanotechnology, Elsevier, 2020, pp. 379–414 Search PubMed
. - M. Oliveira and A. V Machado, Preparation of Polymer-Based Nanocomposites by Different Routes, Nova Science Publishers, 2013 Search PubMed
. - S. Lal, U. Jana, P. K. Manna, G. P. Mohanta, R. Manavalan and S. L. Pal, Nanoparticle: An overview of preparation and characterization, J. Appl. Pharm. Sci., 2011,(06), 228–234 Search PubMed
. - P. Wang, Y. Li, C. Zhang, F. Que, J. Weiss and H. Zhang, Characterization and antioxidant activity of trilayer gelatin/dextran-propyl gallate/gelatin films: Electrospinning versus solvent casting, Lebensm. Wiss. Technol., 2020, 128, 109536 CrossRef CAS
. - C. Vasile, Polymeric Nanocomposites and Nanocoatings for Food Packaging: A Review, Materials, 2018, 11(10), 1834 CrossRef PubMed
. - C. Bavatharani, et al., Electrospinning technique for production of polyaniline nanocomposites/nanofibres for multi-functional applications: A review, Synth. Met., 2021, 271, 116609 CrossRef CAS
. - R. A. Caruso and M. Antonietti, Sol−Gel Nanocoating: An Approach to the Preparation of Structured Materials, Chem. Mater., 2001, 13(10), 3272–3282 CrossRef CAS
. - P. Sarkar, R. Choudhary, S. Panigrahi, I. Syed, S. Sivapratha and C. V. Dhumal, Nano-inspired systems in food technology and packaging, Environ. Chem. Lett., 2017, 15(4), 607–622 CrossRef CAS
. - B. Ghanbarzadeh, S. A. Oleyaei and H. Almasi, Nanostructured Materials Utilized in Biopolymer-based Plastics for Food Packaging Applications, Crit. Rev. Food Sci. Nutr., 2015, 55(12), 1699–1723 CrossRef CAS PubMed
. - J.-W. Rhim, H.-M. Park and C.-S. Ha, Bio-nanocomposites for food packaging applications, Prog. Polym. Sci., 2013, 38(10–11), 1629–1652 CrossRef CAS
. - F. Mustafa and S. Andreescu, Nanotechnology-based approaches for food sensing and packaging applications, RSC Adv., 2020, 10(33), 19309–19336 RSC
. - C. Sharma, R. Dhiman, N. Rokana and H. Panwar, Nanotechnology: An Untapped Resource for Food Packaging, Front. Microbiol., 2017, 8, 1735 CrossRef PubMed
. - L. Wang, C. Hu and L. Shao, The antimicrobial activity of nanoparticles: present situation and prospects for the future, Int. J. Nanomed., 2017, 12, 1227–1249 CrossRef CAS PubMed
. - J.-M. Liu, et al., Emerging functional nanomaterials for the detection of food contaminants, Trends Food Sci. Technol., 2018, 71, 94–106 CrossRef CAS
. - G. Fuertes, I. Soto, R. Carrasco, M. Vargas, J. Sabattin and C. Lagos, Intelligent Packaging Systems: Sensors and Nanosensors to Monitor Food Quality and Safety, J. Sens., 2016, 2016, 1–8 Search PubMed
. - M. Carbone, D. T. Donia, G. Sabbatella and R. Antiochia, Silver nanoparticles in polymeric matrices for fresh food packaging, J. King Saud Univ. Sci., 2016, 28(4), 273–279 CrossRef
. - M. Singh and T. Sahareen, Investigation of cellulosic packets impregnated with silver nanoparticles for enhancing shelf-life of vegetables, Lebensm. Wiss. Technol., 2017, 86, 116–122 CrossRef CAS
. - A. Mihaly Cozmuta, et al., Active papers coated with chitosan and containing TiO2 and Ag/TiO2 nanoparticles for increasing the shelf-life of walnut kernels, Cellulose, 2018, 25(9), 5205–5225 CrossRef CAS
. - S. Shankar, D. Khodaei and M. Lacroix, Effect of chitosan/essential oils/silver nanoparticles composite films packaging and gamma irradiation on shelf life of strawberries, Food Hydrocolloids, 2021, 117, 106750 CrossRef CAS
. - E. Kavakebi, A. Anvar, H. Ahari and A. Motalebi, The effect of PVA coated film containing silver nanoparticles synthesized from aqueous Satureja rechingeri extract on shelf life of rainbow trout (Oncorhynchus mykiss) fillet, J. Food BioProcess Eng., 2020, 135–145 Search PubMed
. - T. T. Nguyen, et al., Development of poly (vinyl alcohol)/agar/maltodextrin coating containing silver nanoparticles for banana (Musa acuminate) preservation, Food Packag. Shelf Life, 2021, 29, 100740 CrossRef CAS
. - J. H. Lee, D. Jeong and P. Kanmani, Study on physical and mechanical properties of the biopolymer/silver based active nanocomposite films with antimicrobial activity, Carbohydr. Polym., 2019, 224, 115159 CrossRef CAS PubMed
. - J. Cheng, X. Lin, X. Wu, Q. Liu, S. Wan and Y. Zhang, Preparation of a multifunctional silver nanoparticles polylactic acid food packaging film using mango peel extract, Int. J. Biol. Macromol., 2021, 188, 678–688 CrossRef CAS PubMed
. - S. D. F. Mihindukulasuriya and L.-T. Lim, Nanotechnology development in food packaging: A review, Trends Food Sci. Technol., 2014, 40(2), 149–167 CrossRef CAS
. - E. O. Simbine, L. d C. Rodrigues, J. Lapa-Guimarães, E. S. Kamimura, C. H. Corassin and C. A. F. de Oliveira, Application of silver nanoparticles in food packages: a review, Food Sci. Technol., 2019, 39(4), 793–802 CrossRef
. - Silver Micro-nanoparticles - Properties, Synthesis, Characterization, and Applications, ed. S. Kumar, P. Kumar and C. Shakher Pathak, IntechOpen, 2021 Search PubMed
. - K. Zheng, M. I. Setyawati, D. T. Leong and J. Xie, Antimicrobial silver nanomaterials, Coord. Chem. Rev., 2018, 357, 1–17 CrossRef CAS
. - A. M. Youssef, M. S. Abdel-Aziz and S. M. El-Sayed, Chitosan nanocomposite films based on Ag-NP and Au-NP biosynthesis by Bacillus Subtilis as packaging materials, Int. J. Biol. Macromol., 2014, 69, 185–191 CrossRef CAS PubMed
. - M. K. Morsy, H. H. Khalaf, A. M. Sharoba, H. H. El-Tanahi and C. N. Cutter, Incorporation of Essential Oils and Nanoparticles in Pullulan Films to Control Foodborne Pathogens on Meat and Poultry Products, J. Food Sci., 2014, 79(4) DOI:10.1111/1750-3841.12400
. - M. Ghaffarlou, et al., Green and Facile Synthesis of Pullulan-Stabilized Silver and Gold Nanoparticles for the Inhibition of Quorum Sensing, ACS Appl. Bio Mater., 2022, 5(2), 517–527 CrossRef CAS PubMed
. - S. Mathew, J. Mathew, R. Krishnankutty and S. Babu, Biodegradable and active nanocomposite pouches reinforced with silver nanoparticles for improved packaging of chicken sausages, Food Packag. Shelf Life, 2019, 19, 155–166 CrossRef
. - A. Bahrami, R. Rezaei Mokarram, M. Sowti Khiabani, B. Ghanbarzadeh and R. Salehi, Physico-mechanical and antimicrobial properties of tragacanth/hydroxypropyl methylcellulose/beeswax edible films reinforced with silver nanoparticles, Int. J. Biol. Macromol., 2019, 129, 1103–1112 CrossRef CAS PubMed
. - S. B. Patri, P. S. Adarakatti and P. Malingappa, Silver Nanoparticles-Chitosan Composite Embedded Graphite Screen-Printed Electrodes as a Novel Electrochemical Platform in the Measurement of Trace Level Nitrite: Application to Milk Powder Samples, Curr. Anal. Chem., 2018, 15(1), 56–65 CrossRef
. - A. Sobhan, K. Muthukumarappan, L. Wei, R. Zhou and N. Ghimire, Development of a Biosensor with Electrically Conductive
and Biodegradable Composite by Combinatory Use of Silver Nanoparticles, Novel Activated Biochar, and Polylactic Acid, J. Electrochem. Soc., 2021, 168(10), 107501 CrossRef CAS
. - D. Sahu, N. Sarkar, G. Sahoo, P. Mohapatra and S. K. Swain, Nano silver imprinted polyvinyl alcohol nanocomposite thin films for Hg2+ sensor, Sens. Actuators, B, 2017, 246, 96–107 CrossRef CAS
. - A. Orsuwan, S. Shankar, L.-F. Wang, R. Sothornvit and J.-W. Rhim, Preparation of antimicrobial agar/banana powder blend films reinforced with silver nanoparticles, Food Hydrocolloids, 2016, 60, 476–485 CrossRef CAS
. - P. Kanmani and J.-W. Rhim, Physicochemical properties of gelatin/silver nanoparticle antimicrobial composite films, Food Chem., 2014, 148, 162–169 CrossRef CAS PubMed
. - M. Pinaki and G. Samaresh, Green Approach to the Synthesis of Poly(Vinyl Alcohol)-Silver Nanoparticles Hybrid Using Rice Husk Extract and Study of its Antibacterial Activity, Biointerface Res. Appl. Chem., 2020, 10(5), 6474–6480 Search PubMed
. - O. Gherasim, R. A. Puiu, A. C. Bîrcă, A.-C. Burduşel and A. M. Grumezescu, An Updated Review on Silver Nanoparticles in Biomedicine, Nanomaterials, 2020, 10(11), 2318 CrossRef CAS PubMed
. - A. Sharma, A. Sagar, J. Rana and R. Rani, Green synthesis of silver nanoparticles and its antibacterial activity using fungus Talaromyces purpureogenus isolated from Taxus baccata Linn, Micro Nano Syst. Lett., 2022, 10(1), 2 CrossRef
. - B. Chopade, et al., Synthesis of silver nanoparticles using Dioscorea bulbifera tuber extract and evaluation of its synergistic potential in combination with antimicrobial agents, Int. J. Nanomed., 2012, 483 CrossRef PubMed
. - A. Istiqola and A. Syafiuddin, A review of silver nanoparticles in food packaging technologies: Regulation, methods, properties, migration, and future challenges, J. Chin. Chem. Soc., 2020, 67(11), 1942–1956 CrossRef CAS
. - EFSA Panel on Food Contact Materials, Enzymes and Processing Aids (CEP), C. Lambré, J. M. Barat Baviera, C. Bolognesi, A. Chesson, P. S. Cocconcelli and G. Rivière, et al., Safety assessment of the substance silver nanoparticles for use in food contact materials, EFSA J., 2021, 19(8), e06790 Search PubMed
. - United States Environmental Protection Agency, Drinking Water Regulations and Contaminants, 2024 Search PubMed
. - B. Panea, G. Ripoll, J. González, Á. Fernández-Cuello and P. Albertí, Effect of nanocomposite packaging containing different proportions of ZnO and Ag on chicken breast meat quality, J. Food Eng., 2014, 123, 104–112 CrossRef CAS
. - F. Bagheri-Abassi, H. Alavi, A. Mohammadipour, F. Motejaded and A. Ebrahimzadeh-Bideskan, The effect of silver nanoparticles on apoptosis and dark neuron production in rat hippocampus, Iran. J. Basic Med. Sci., 2015, 18(7), 644–648 Search PubMed
. - S. S. Ahmad, O. Yousuf, R. U. Islam and K. Younis, Silver nanoparticles as an active packaging ingredient and its toxicity, Packag. Technol. Sci., 2021, 34(11–12), 653–663 CrossRef CAS
. - Y. Echegoyen and C. Nerín, Nanoparticle release from nano-silver antimicrobial food containers, Food Chem. Toxicol., 2013, 62, 16–22 CrossRef CAS PubMed
. - F. Gallocchio, et al., Testing nano-silver food packaging to evaluate silver migration and food spoilage bacteria on chicken meat, Food Addit. Contam.: Part A, 2016, 33(6), 1063–1071 CrossRef CAS PubMed
. - W. Li, L. Li, H. Zhang, M. Yuan and Y. Qin, Evaluation of PLA nanocomposite films on physicochemical and microbiological properties of refrigerated cottage cheese, J. Food Process. Preserv., 2018, 42(1), e13362 CrossRef
. - M. Cushen, J. Kerry, M. Morris, M. Cruz-Romero and E. Cummins, Nanotechnologies in the food industry – Recent developments, risks and regulation, Trends Food Sci. Technol., 2012, 24(1), 30–46 CrossRef CAS
. - H. Sharifan, A. Noori, M. Bagheri and J. M. Moore, Postharvest spraying of zinc oxide nanoparticles enhances shelf life qualities and zinc concentration of tomato fruits, Crop Pasture Sci., 2021, 73(2), 22–31 CrossRef
. - P. J. P. Espitia, C. G. Otoni and N. F. F. Soares, Zinc Oxide Nanoparticles for Food Packaging Applications, in Antimicrobial Food Packaging, Elsevier, 2016, pp. 425–431 Search PubMed
. - M. Azizi-Lalabadi, A. Ehsani, B. Ghanbarzadeh and B. Divband, Polyvinyl alcohol/gelatin nanocomposite containing ZnO, TiO2 or ZnO/TiO2 nanoparticles doped on 4A zeolite: Microbial and sensory qualities of packaged white shrimp during refrigeration, Int. J. Food Microbiol., 2020, 312, 108375 CrossRef CAS PubMed
. - L. Yang, et al., Preparation and characterization of PVA/arginine chitosan/ZnO NPs composite films, Int. J. Biol. Macromol., 2023, 226, 184–193 CrossRef CAS PubMed
. - A. Jayakumar, et al., Starch-PVA composite films with zinc-oxide nanoparticles and phytochemicals as intelligent pH sensing wraps for food packaging application, Int. J. Biol. Macromol., 2019, 136, 395–403 CrossRef CAS PubMed
. - M. P. Indumathi, K. Saral Sarojini and G. R. Rajarajeswari, Antimicrobial and biodegradable chitosan/cellulose acetate phthalate/ZnO nano composite films with optimal oxygen permeability and hydrophobicity for extending the shelf life of black grape fruits, Int. J. Biol. Macromol., 2019, 132, 1112–1120 CrossRef CAS PubMed
. - S. Roy, R. Priyadarshi and J.-W. Rhim, Development of Multifunctional Pullulan/Chitosan-Based Composite Films Reinforced with ZnO Nanoparticles and Propolis for Meat Packaging Applications, Foods, 2021, 10(11), 2789 CrossRef CAS PubMed
. - R. Priyadarshi and Y. S. Negi, Effect of Varying Filler Concentration on Zinc Oxide Nanoparticle Embedded Chitosan Films as Potential Food Packaging Material, J. Polym. Environ., 2017, 25(4), 1087–1098 CrossRef CAS
. - W. Li, L. Li, Y. Cao, T. Lan, H. Chen and Y. Qin, Effects of PLA Film Incorporated with ZnO Nanoparticle on the Quality Attributes of Fresh-Cut Apple, Nanomaterials, 2017, 7(8), 207 CrossRef PubMed
. - A. Marra, C. Silvestre, D. Duraccio and S. Cimmino, Polylactic acid/zinc oxide biocomposite films for food packaging application, Int. J. Biol. Macromol., 2016, 88, 254–262 CrossRef CAS PubMed
. - S. Shankar, L.-F. Wang and J.-W. Rhim, Incorporation of zinc oxide nanoparticles improved the mechanical, water vapor barrier, UV-light barrier, and antibacterial properties of PLA-based nanocomposite films, Mater. Sci. Eng. C, 2018, 93, 289–298 CrossRef CAS PubMed
. - V. Hooda and Archita, Enzymes loaded chitosan/coconut fibre/zinc oxide nanoparticles strip for polyamine determination, Food Chem., 2018, 239, 1100–1109 CrossRef CAS PubMed
. - A. M. Hezma, A. Rajeh and M. A. Mannaa, An insight into the effect of zinc oxide nanoparticles on the structural, thermal, mechanical properties and antimicrobial activity of Cs/PVA composite, Colloids Surf., A, 2019, 581, 123821 CrossRef CAS
. - A. Tymczewska, B. U. Furtado, J. Nowaczyk, K. Hrynkiewicz and A. Szydłowska-Czerniak, Functional Properties of Gelatin/Polyvinyl Alcohol Films Containing Black Cumin Cake Extract and Zinc Oxide Nanoparticles Produced via Casting Technique, Int. J. Mol. Sci., 2022, 23(5), 2734 CrossRef CAS PubMed
. - Y. Amaregouda, K. Kamanna and A. Kamath, Multifunctional Bionanocomposite Films Based on Chitosan/Polyvinyl Alcohol with ZnO NPs and Carissa carandas Extract Anthocyanin for Smart Packaging Materials, ACS Food Sci. Technol., 2023, 3(9), 1411–1422 CrossRef CAS
. - S. K. Bajpai, N. Chand and V. Chaurasia, Investigation of water vapor permeability and antimicrobial property of zinc oxide nanoparticles-loaded chitosan-based edible film, J. Appl. Polym. Sci., 2010, 115(2), 674–683 CrossRef CAS
. - B. Lallo da Silva, et al., Relationship Between Structure And Antimicrobial Activity Of Zinc Oxide Nanoparticles: An Overview, Int. J. Nanomed., 2019, 14, 9395–9410 CrossRef PubMed
. - A. Król, P. Pomastowski, K. Rafińska, V. Railean-Plugaru and B. Buszewski, Zinc oxide nanoparticles: Synthesis, antiseptic activity and toxicity mechanism, Adv. Colloid Interface Sci., 2017, 249, 37–52 CrossRef PubMed
. - C. Silvestre, D. Duraccio and S. Cimmino, Food packaging based on polymer nanomaterials, Prog. Polym. Sci., 2011, 36(12), 1766–1782 CrossRef CAS
. - A. Emamifar, M. Kadivar, M. Shahedi and S. Soleimanian-Zad, Evaluation of nanocomposite packaging containing Ag and ZnO on shelf life of fresh orange juice, Innovat. Food Sci. Emerg. Technol., 2010, 11(4), 742–748 CrossRef CAS
. - W. Zhang and J.-W. Rhim, Titanium dioxide (TiO2) for the manufacture of multifunctional active food packaging films, Food Packag. Shelf Life, 2022, 31, 100806 CrossRef CAS
. - P. N. Navya, A. Kaphle, S. P. Srinivas, S. K. Bhargava, V. M. Rotello and H. K. Daima, Current trends and challenges in cancer management and therapy using designer nanomaterials, Nano Convergence, 2019, 6(1), 23 CrossRef CAS PubMed
. - A. Maldonado, et al., Study of Ethylene-Removing Materials Based on Eco-Friendly Composites with Nano-TiO2, Polymers, 2023, 15(16), 3369 CrossRef CAS PubMed
. - M. Alizadeh Sani, et al., Titanium dioxide nanoparticles as multifunctional surface-active materials for smart/active nanocomposite packaging films, Adv. Colloid Interface Sci., 2022, 300, 102593 CrossRef CAS PubMed
. - M. Alizadeh-Sani, E. Mohammadian and D. J. McClements, Eco-friendly active packaging consisting of nanostructured biopolymer matrix reinforced with TiO2 and essential oil: Application for preservation of refrigerated meat, Food Chem., 2020, 322, 126782 CrossRef CAS PubMed
. - U. Siripatrawan and P. Kaewklin, Fabrication and characterization of chitosan-titanium dioxide nanocomposite film as ethylene scavenging and antimicrobial active food packaging, Food Hydrocolloids, 2018, 84, 125–134 CrossRef CAS
. - F. Tian, et al., Preservation of Ginkgo biloba seeds by coating with chitosan/nano-TiO2 and chitosan/nano-SiO2 films, Int. J. Biol. Macromol., 2019, 126, 917–925 CrossRef CAS PubMed
. - X. Zhang, Z. Li, R. Ji, K. Li and W. Zhang, Preparation and Characterization of Pullulan/Carboxymethyl Cellulose/Nano-TiO2 Composite Films for Strawberry Preservation, Food Biophys., 2021, 16(4), 460–473 CrossRef
. - Z.-P. Tang, C.-W. Chen and J. Xie, Development of antimicrobial active films based on poly(vinyl alcohol) containing nano-TiO 2 and its application in macrobrachium rosenbergii packaging, J. Food Process. Preserv., 2018, 42(8), e13702 CrossRef
. - R. Chougale, D. Kasai, S. Nayak, S. Masti, A. Nasalapure and A. V Raghu, Design of eco-friendly PVA/TiO 2 -based nanocomposites and their antifungal activity study, Green Mater., 2020, 8(1), 40–48 CrossRef
. - J. Alberton, S. M. Martelli, F. M. Fakhouri and V. Soldi, Mechanical and moisture barrier properties of titanium dioxide nanoparticles and halloysite nanotubes reinforced polylactic acid (PLA), IOP Conf. Ser. Mater. Sci. Eng., 2014, 64, 012010 CrossRef CAS
. - L. Qu, et al., Improved mechanical and antimicrobial properties of zein/chitosan films by adding highly dispersed nano-TiO2, Ind. Crops Prod., 2019, 130, 450–458 Search PubMed
. - W. Li, K. Zheng, H. Chen, S. Feng, W. Wang and C. Qin, Influence of Nano Titanium Dioxide and Clove Oil on Chitosan–Starch Film Characteristics, Polymers, 2019, 11(9), 1418 CrossRef CAS PubMed
. - L. Zhang, W. Xia, X. Liu and W. Zhang, Synthesis of titanium cross-linked chitosan composite for efficient adsorption and detoxification of hexavalent chromium from water, J. Mater. Chem. A, 2015, 3(1), 331–340 Search PubMed
. - V. Katiyar and T. Ghosh, Nanotechnology in Edible Food Packaging, Springer Singapore, Singapore, 2021 Search PubMed
. - X. Zhang, Y. Liu, H. Yong, Y. Qin, J. Liu and J. Liu, Development of multifunctional food packaging films based on chitosan, TiO2 nanoparticles and anthocyanin-rich black plum peel extract, Food Hydrocolloids, 2019, 94, 80–92 Search PubMed
. - B. Li, et al., Synthesis, characterization, and antibacterial activity of chitosan/TiO 2 nanocomposite against Xanthomonas oryzae pv. oryzae, Carbohydr. Polym., 2016, 152, 825–831 CrossRef CAS PubMed
. - B. Lin, Y. Luo, Z. Teng, B. Zhang, B. Zhou and Q. Wang, Development of silver/titanium dioxide/chitosan adipate nanocomposite as an antibacterial coating for fruit storage, LWT–Food Sci. Technol., 2015, 63(2), 1206–1213 CrossRef CAS
. - S. Feng, F. Zhang, S. Ahmed and Y. Liu, Physico-Mechanical and Antibacterial Properties of PLA/TiO2 Composite Materials
Synthesized via Electrospinning and Solution Casting Processes, Coatings, 2019, 9(8), 525 CrossRef CAS
. - A. Tajdari, A. Babaei, A. Goudarzi and R. Partovi, Preparation and study on the optical, mechanical, and antibacterial properties of polylactic acid/ZnO/TiO 2 shared nanocomposites, J. Plast. Film Sheeting, 2020, 36(3), 285–311 CrossRef CAS
. - S. Ramesh, H. S. Kim and J.-H. Kim, Cellulose–Polyvinyl Alcohol–Nano-TiO 2 Hybrid Nanocomposite: Thermal, Optical, and Antimicrobial Properties against Pathogenic Bacteria, Polym. Plast. Technol. Eng., 2018, 57(7), 669–681 CrossRef CAS
. - J. Luo, G. Xia, L. Liu, A. Ji and Q. Luo, Fabrication of Chitosan/Hydroxyethyl Cellulose/TiO2 Incorporated Mulberry Anthocyanin 3D-Printed Bilayer Films for Quality of Litchis, Foods, 2022, 11(20), 3286 CrossRef CAS PubMed
. - K. Jiang, et al., Smart Indicator Film Based on Sodium Alginate/Polyvinyl Alcohol/TiO2 Containing Purple Garlic Peel Extract for Visual Monitoring of Beef Freshness, Polymers, 2023, 15(21), 4308 CrossRef CAS PubMed
. - S. Pirsa and S. Asadi, Innovative smart and biodegradable packaging for margarine based on a nano composite polylactic acid/lycopene film, Food Addit. Contam.: Part A, 2021, 38(5), 856–869 Search PubMed
. - P. A. Christensen, T. P. Curtis, T. A. Egerton, S. A. M. Kosa and J. R. Tinlin, Photoelectrocatalytic and photocatalytic disinfection of E. coli suspensions by titanium dioxide, Appl. Catal., B, 2003, 41(4), 371–386 Search PubMed
. - Z. Chen, S. Han, S. Zhou, H. Feng, Y. Liu and G. Jia, Review of health safety aspects of titanium dioxide nanoparticles in food application, NanoImpact, 2020, 18, 100224 Search PubMed
. - Q.-B. Lin, H. Li, H.-N. Zhong, Q. Zhao, D.-H. Xiao and Z.-W. Wang, Migration of Ti from nano-TiO 2 -polyethylene composite packaging into food simulants, Food Addit. Contam.: Part A, 2014, 1–7 Search PubMed
. - Z. Lian, Y. Zhang and Y. Zhao, Nano-TiO 2 particles and high hydrostatic pressure treatment for improving functionality of polyvinyl alcohol and chitosan composite films and nano-TiO 2 migration from film matrix in food simulants, Innovat. Food Sci. Emerg. Technol., 2016, 33, 145–153 Search PubMed
. - W. Li, L. Li, H. Zhang, M. Yuan and Y. Qin, Evaluation of PLA nanocomposite films on physicochemical and microbiological properties of refrigerated cottage cheese, J. Food Process. Preserv., 2018, 42(1), e13362 Search PubMed
. - W. Zhang, S. Roy and J. Rhim, Copper-based nanoparticles for biopolymer-based functional films in food packaging applications, Compr. Rev. Food Sci. Food Saf., 2023, 22(3), 1933–1952 Search PubMed
. - A. Kalia, et al., Nettle-Leaf Extract Derived ZnO/CuO Nanoparticle-Biopolymer-Based Antioxidant and Antimicrobial Nanocomposite Packaging Films and Their Impact on Extending the Post-Harvest Shelf Life of Guava Fruit, Biomolecules, 2021, 11(2), 224 Search PubMed
. - A. Nouri, M. T. Yaraki, M. Ghorbanpour, S. Agarwal and V. K. Gupta, Enhanced Antibacterial effect of chitosan film using Montmorillonite/CuO nanocomposite, Int. J. Biol. Macromol., 2018, 109, 1219–1231 Search PubMed
. - A. M. Youssef, F. M. Assem, H. S. El-Sayed, S. M. El-Sayed, M. Elaaser and M. H. Abd El-Salam, Synthesis and evaluation of eco-friendly carboxymethyl cellulose/polyvinyl alcohol/CuO bionanocomposites and their use in coating processed cheese, RSC Adv., 2020, 10(62), 37857–37870 Search PubMed
. - D. V. Francis, S. Thaliyakattil, L. Cherian, N. Sood and T. Gokhale, Metallic Nanoparticle Integrated Ternary Polymer Blend of PVA/Starch/Glycerol: A Promising Antimicrobial Food Packaging Material, Polymers, 2022, 14(7), 1379 CrossRef CAS PubMed
. - P. Abdolsattari, M. Rezazadeh-Bari and S. Pirsa, Smart Film Based on Polylactic Acid, Modified with Polyaniline/ZnO/CuO: Investigation of Physicochemical Properties and Its Use of Intelligent Packaging of Orange Juice, Food Bioproc. Tech., 2022, 15(12), 2803–2825 Search PubMed
. - H. Tang, et al., Effects and Mechanism of Nano-Copper Exposure on Hepatic Cytochrome P450 Enzymes in Rats, Int. J. Mol. Sci., 2018, 19(7), 2140 CrossRef PubMed
. - T. Revathi and S. Thambidurai, Cytotoxic, antioxidant and antibacterial activities of copper oxide incorporated chitosan-neem seed biocomposites, Int. J. Biol. Macromol., 2019, 139, 867–878 CrossRef CAS PubMed
. - M. Fathi, M. Samadi, S. Abbaszadeh and M. R. Nourani, Fabrication and characterization of multifunctional bio-safe films based on Carboxymethyl Chitosan and Saffron Petal Anthocyanin Reinforced with Copper Oxide Nanoparticles for sensing the meat freshness, J. Polym. Environ., 2022, 30(11), 4538–4549 Search PubMed
. - Gh. D. Khalili, S. Alipour, M. R. Akbarpour and S. Moniri Javadhesari, Fabrication, Characterization and Investigating Properties of PVA Based Nanocomposite Films Reinforced with Mechano-chemically Synthesized CuO Nanoparticles, Met. Mater. Int., 2023, 29(8), 2374–2384 CrossRef CAS
. - Y. Haldorai and J.-J. Shim, Novel chitosan-TiO 2 nanohybrid: Preparation, characterization, antibacterial, and photocatalytic properties, Polym. Compos., 2014, 35(2), 327–333 CrossRef CAS
. - A. Errokh, et al., Controlled growth of Cu 2 O nanoparticles bound to cotton fibres, Carbohydr. Polym., 2016, 141, 229–237 Search PubMed
. - C. Gunawan, W. Y. Teoh, C. P. Marquis and R. Amal, Cytotoxic Origin of Copper(II) Oxide Nanoparticles: Comparative Studies with Micron-Sized Particles, Leachate, and Metal Salts, ACS Nano, 2011, 5(9), 7214–7225 CrossRef CAS PubMed
. - P. Asgari, O. Moradi and B. Tajeddin, The effect of nanocomposite packaging carbon nanotube base on organoleptic and fungal growth of Mazafati brand dates, Int. Nano Lett., 2014, 4(1), 98 CrossRef
. - H. Hosseini and S. M. Jafari, Introducing nano/microencapsulated bioactive ingredients for extending the shelf-life of food products, Adv. Colloid Interface Sci., 2020, 282, 102210 Search PubMed
. - Y.-H. Wen, et al., Antibacterial nanocomposite films of poly(vinyl alcohol) modified with zinc oxide-doped multiwalled carbon nanotubes as food packaging, Polym. Bull., 2022, 79(6), 3847–3866 CrossRef CAS
. - G. Khachatryan, K. Khachatryan, J. Szczepankowska, M. Krzan and M. Krystyjan, Design of Carbon Nanocomposites Based on Sodium Alginate/Chitosan Reinforced with Graphene Oxide and Carbon Nanotubes, Polymers, 2023, 15(4), 925, DOI:10.3390/polym15040925
. - J. Luo, W. Sun, H. Zhou, Y. Zhang, B. Wen and C. Xin, Bioderived and Biodegradable Poly(3-hydroxybutyrate- co -3-hydroxyvalerate) Nanocomposites Based on Carbon Nanotubes: Microstructure Observation and EMI Shielding Property Improvement, ACS Sustain. Chem. Eng., 2021, 9(32), 10785–10798 CrossRef CAS
. - F. Z. Yakdoumi, A. S. Hadj-Hamou, N. Rahoui, M. M. Rahman and V. Abetz, Polylactic acid nanocomposites containing functionalized multiwalled carbon nanotubes as antimicrobial packaging materials, Int. J. Biol. Macromol., 2022, 213, 55–69 CrossRef CAS PubMed
. - P. G. Seligra, M. Lamanna and L. Famá, PLA-fMWCNT Bionanofilms with High Modulus and Great Properties to Apply in Packaging and Biomedicine, Procedia Food Sci., 2015, 8, 383–390 CAS
. - R. Cui, et al., Antimicrobial film based on polylactic acid and carbon nanotube for controlled cinnamaldehyde release, J. Mater. Res. Technol., 2020, 9(5), 10130–10138 CrossRef CAS
. - L. Gan, et al., Antibacterial nanocomposite based on carbon nanotubes–silver nanoparticles-co-doped polylactic acid, Polym. Bull., 2020, 77(2), 793–804 CrossRef CAS
. - D. G. Goodwin, et al., Interactions of Microorganisms with Polymer Nanocomposite Surfaces Containing Oxidized Carbon Nanotubes, Environ. Sci. Technol., 2015, 49(9), 5484–5492 CrossRef CAS PubMed
. - E.-S. Lee, et al., Antimicrobial properties of lignin-decorated thin multi-walled carbon nanotubes in poly(vinyl alcohol) nanocomposites, Eur. Polym. J., 2018, 105, 79–84 Search PubMed
. - S. Hui and N. C. Das, Surface Modified Carbon Nanotubes in Food Packaging, 2022, pp. 199–233 Search PubMed
. - B. Rezaei, H. R. Jamei and A. A. Ensafi, An ultrasensitive and selective electrochemical aptasensor based on rGO-MWCNTs/Chitosan/carbon quantum dot for the detection of lysozyme, Biosens. Bioelectron., 2018, 115, 37–44 CrossRef CAS PubMed
. - A. A. P. Khan, A. Khan, M. M. Rahman, A. M. Asiri and M. Oves, Lead sensors development and antimicrobial activities
based on graphene oxide/carbon nanotube/poly(O-toluidine) nanocomposite, Int. J. Biol. Macromol., 2016, 89, 198–205 CrossRef CAS PubMed
. - Yu. G. Maksimova, Microorganisms and Carbon Nanotubes: Interaction and Applications (Review), Appl. Biochem. Microbiol., 2019, 55(1), 1–12 CrossRef CAS
. - Composites Materials for Food Packaging, ed. G. Cirillo, M. A. Kozlowski and U. G. Spizzirri, Wiley, 2018 Search PubMed
. - V. Srivastava, D. Gusain and Y. C. Sharma, Critical Review on the Toxicity of Some Widely Used Engineered Nanoparticles, Ind. Eng. Chem. Res., 2015, 54(24), 6209–6233 Search PubMed
. - J. Bott, A. Störmer and R. Franz, Migration of nanoparticles from plastic packaging materials containing carbon black into foodstuffs, Food Addit. Contam.: Part A, 2014, 31(10), 1769–1782 CrossRef CAS PubMed
. - F. Han Lyn, C. P. Tan, R. M. Zawawi and Z. A. Nur Hanani, Physicochemical properties of chitosan/graphene oxide composite films and their effects on storage stability of palm-oil based margarine, Food Hydrocolloids, 2021, 117, 106707 Search PubMed
. - Z. Gou, D. Xu, Q. Dong and X. Wu, Comparison studies on covalently and non-covalently modified MWNTs using chitosan and their starch nanocomposites, Starch/Staerke, 2016, 68(3–4), 220–229 Search PubMed
. - T. Nguyen, et al., Impact of UV irradiation on multiwall carbon nanotubes in nanocomposites: Formation of entangled surface layer and mechanisms of release resistance, Carbon, 2017, 116, 191–200 CrossRef CAS PubMed
. - W. Xu, et al., The graphene oxide and chitosan biopolymer loads TiO2 for antibacterial and preservative research, Food Chem., 2017, 221, 267–277 CrossRef CAS PubMed
. - J. C. Vilvert, et al., Chitosan and graphene oxide-based biodegradable bags: An eco-friendly and effective packaging alternative to maintain postharvest quality of ‘Palmer’ mango, Lebensm. Wiss. Technol., 2022, 154, 112741 CrossRef CAS
. - V. Loryuenyong, C. Saewong, C. Aranchaiya and A. Buasri, The Improvement in Mechanical and Barrier Properties of Poly(Vinyl Alcohol)/Graphene Oxide Packaging Films, Packag. Technol. Sci., 2015, 28(11), 939–947 CrossRef CAS
. - S. Pourmoslemi, S. Shokouhi and R. Mahjub, Investigation of antibacterial activity of polyvinyl alcohol packaging films composed of silver oxide nanoparticles, graphene oxide and tragacanth gum using Box–Behnken design, Packag. Technol. Sci., 2021, 34(10), 613–622 CrossRef CAS
. - Y. A. Arfat, J. Ahmed, M. Ejaz and M. Mullah, Polylactide/graphene oxide nanosheets/clove essential oil composite films for potential food packaging applications, Int. J. Biol. Macromol., 2018, 107, 194–203 CrossRef CAS PubMed
. - Y. Kim, J. S. Kim, S.-Y. Lee, R. L. Mahajan and Y.-T. Kim, Exploration of hybrid nanocarbon composite with polylactic acid for packaging applications, Int. J. Biol. Macromol., 2020, 144, 135–142 CrossRef CAS PubMed
. - W. Chen, H. Weimin, D. Li, S. Chen and Z. Dai, A critical review on the development and performance of polymer/graphene nanocomposites, Sci. Eng. Compos. Mater., 2018, 25(6), 1059–1073 CrossRef CAS
. - M. Azizi-Lalabadi, H. Hashemi, J. Feng and S. M. Jafari, Carbon nanomaterials against pathogens; the antimicrobial activity of carbon nanotubes, graphene/graphene oxide, fullerenes, and their nanocomposites, Adv. Colloid Interface Sci., 2020, 284, 102250 CrossRef CAS PubMed
. - A. Ray Chowdhuri, S. Tripathy, S. Chandra, S. Roy and S. K. Sahu, A ZnO decorated chitosan–graphene oxide nanocomposite shows significantly enhanced antimicrobial activity with ROS generation, RSC Adv., 2015, 5(61), 49420–49428 RSC
. - H. Khawaja, E. Zahir, M. A. Asghar and M. A. Asghar, Graphene oxide, chitosan and silver nanocomposite as a highly effective antibacterial agent against pathogenic strains, Colloids Surf., A, 2018, 555, 246–255 CrossRef CAS
. - H. Moustafa, M. H. Hemida, M. A. Shemis, A. Dufresne and M. Morsy, Functionalized GO nanoplatelets with folic acid as a novel material for boosting humidity sensing of chitosan/PVA nanocomposites for active food packaging, Surface. Interfac., 2023, 41, 103229 CrossRef CAS
. - W. C. Liew, I. I. Muhamad, J. W. Chew and K. J. A. Karim, Synergistic effect of graphene oxide/zinc oxide nanocomposites on polylactic acid-based active packaging film: Properties, release kinetics and antimicrobial efficiency, Int. J. Biol. Macromol., 2023, 253, 127288 CrossRef CAS PubMed
. - Y. Huang, et al., Poly(lactic acid)/graphene oxide-ZnO nanocomposite films with good mechanical, dynamic mechanical, anti-UV and antibacterial properties, J. Chem. Technol. Biotechnol., 2015, 90(9), 1677–1684 CrossRef CAS
. - M. Dhanasekar, et al., Ambient light antimicrobial activity of reduced graphene oxide supported metal doped TiO2 nanoparticles and their PVA based polymer nanocomposite films, Mater. Res. Bull., 2018, 97, 238–243 CrossRef CAS
. - S. Gautam, S. Sharma, B. Sharma and P. Jain, Antibacterial efficacy of poly (vinyl alcohol) nanocomposites reinforced with graphene oxide and silver nanoparticles for packaging applications, Polym. Compos., 2021, 42(6), 2829–2837 CrossRef CAS
. - A. Usman, Z. Hussain, A. Riaz and A. N. Khan, Enhanced mechanical, thermal and antimicrobial properties of poly(vinyl alcohol)/graphene oxide/starch/silver nanocomposites films, Carbohydr. Polym., 2016, 153, 592–599 CrossRef CAS PubMed
. - T. Siripongpreda, K. Siralertmukul and N. Rodthongkum, Colorimetric sensor and LDI-MS detection of biogenic amines in food spoilage based on porous PLA and graphene oxide, Food Chem., 2020, 329, 127165 CrossRef CAS PubMed
. - K. Yang, H. Gong, X. Shi, J. Wan, Y. Zhang and Z. Liu, In vivo biodistribution and toxicology of functionalized nano-graphene oxide in mice after oral and intraperitoneal administration, Biomaterials, 2013, 34(11), 2787–2795 CrossRef CAS PubMed
. - J.-H. Liu, S.-T. Yang, H. Wang, Y. Chang, A. Cao and Y. Liu, Effect of Size and Dose on The Biodistribution of Graphene Oxide in Mice, Nanomedicine, 2012, 7(12), 1801–1812 CrossRef CAS PubMed
. - T. H. D. Nguyen, M. Lin and A. Mustapha, Toxicity of Graphene Oxide on Intestinal Bacteria and Caco-2 Cells, J. Food Prot., 2015, 78(5), 996–1002 CrossRef CAS PubMed
. - N. A. Manikandan, K. Pakshirajan and G. Pugazhenthi, Preparation and characterization of environmentally safe and highly biodegradable microbial polyhydroxybutyrate (PHB) based graphene nanocomposites for potential food packaging applications, Int. J. Biol. Macromol., 2020, 154, 866–877 CrossRef CAS PubMed
. - Y. Wang, L. Wang, L. Jin, J. Wang and D. Jin, Evolutionary Analysis of Cellulose Gene Family in Grasses, 2011, pp. 178–183 Search PubMed
. - J. I. Morán, V. A. Alvarez, V. P. Cyras and A. Vázquez, Extraction of cellulose and preparation of nanocellulose from sisal fibers, Cellulose, 2008, 15(1), 149–159 CrossRef
. - N. Lin and A. Dufresne, Nanocellulose in biomedicine: Current status and future prospect, Eur. Polym. J., 2014, 59, 302–325 CrossRef CAS
. - A. Anžlovar, M. Kunaver, A. Krajnc and E. Žagar, Nanocomposites of LLDPE and Surface-Modified Cellulose Nanocrystals Prepared by Melt Processing, Molecules, 2018, 23(7), 1782 CrossRef PubMed
. - A. B. Perumal, P. S. Sellamuthu, R. B. Nambiar and E. R. Sadiku, Development of polyvinyl alcohol/chitosan bio-nanocomposite films reinforced with cellulose nanocrystals isolated from rice straw, Appl. Surf. Sci., 2018, 449, 591–602 CrossRef CAS
. - M. El Achaby, et al., Processing and properties of eco-friendly bio-nanocomposite films filled with cellulose nanocrystals from sugarcane bagasse, Int. J. Biol. Macromol., 2017, 96, 340–352 CrossRef CAS PubMed
. - M. Yadav and F.-C. Chiu, Cellulose nanocrystals reinforced κ-carrageenan based UV resistant transparent bionanocomposite films for sustainable packaging applications, Carbohydr. Polym., 2019, 211, 181–194 CrossRef CAS PubMed
. - A. Khan, et al., Mechanical and barrier properties of nanocrystalline cellulose reinforced chitosan based nanocomposite films, Carbohydr. Polym., 2012, 90(4), 1601–1608 CrossRef CAS PubMed
. - J. P. de Mesquita, C. L. Donnici, I. F. Teixeira and F. V. Pereira, Bio-based nanocomposites obtained through covalent linkage between chitosan and cellulose nanocrystals, Carbohydr. Polym., 2012, 90(1), 210–217 CrossRef CAS PubMed
. - R. Mu, et al., Recent
trends and applications of cellulose nanocrystals in food industry, Trends Food Sci. Technol., 2019, 93, 136–144 CrossRef CAS
. - L. Meng, et al., Improved mechanical and antibacterial properties of polyvinyl alcohol composite films using quaternized cellulose nanocrystals as nanofillers, Compos. Sci. Technol., 2023, 232, 109885 CrossRef CAS
. - S. Huang, S. Zou and Y. Wang, Construction of compostable packaging with antibacterial property and improved performance using sprayed coatings of modified cellulose nanocrystals, Carbohydr. Polym., 2023, 305, 120539 CrossRef CAS PubMed
. - L. S. F. Leite, et al., Eco-friendly gelatin films with rosin-grafted cellulose nanocrystals for antimicrobial packaging, Int. J. Biol. Macromol., 2020, 165, 2974–2983 CrossRef CAS PubMed
. - S. M. Costa, D. P. Ferreira, P. Teixeira, L. F. Ballesteros, J. A. Teixeira and R. Fangueiro, Active natural-based films for food packaging applications: The combined effect of chitosan and nanocellulose, Int. J. Biol. Macromol., 2021, 177, 241–251 CrossRef CAS PubMed
. - C. A. Díaz-Cruz, C. Caicedo, E. J. Jiménez-Regalado, R. Díaz de León, R. López-González and R. Y. Aguirre-Loredo, Evaluation of the Antimicrobial, Thermal, Mechanical, and Barrier Properties of Corn Starch–Chitosan Biodegradable Films Reinforced with Cellulose Nanocrystals, Polymers, 2022, 14(11), 2166 CrossRef PubMed
. - R. Koshani and A. Madadlou, A viewpoint on the gastrointestinal fate of cellulose nanocrystals, Trends Food Sci. Technol., 2018, 71, 268–273 CrossRef CAS
. - H. Ni, et al., Cellulose nanowhiskers: Preparation, characterization and cytotoxicity evaluation, Bio-Med. Mater. Eng., 2012, 22(1–3), 121–127 Search PubMed
. - S. Dong, A. A. Hirani, K. R. Colacino, Y. W. Lee and M. Roman, Cytotoxicity and cellular uptake of cellulose nanocrystals, Nano LIFE, 2012, 02(03), 1241006 CrossRef
. - EFSA Scientific Committee, A. Hardy, D. B. T. Halldorsson, M. J. Jeger, H. K. Knutsen, S. More, H. Naegeli, H. Noteborn, C. Ockleford, A. Ricci, G. Rychen, J. R. Schlatter, V. Silano, R. Solecki, D. Turck, M. Younes, Q. Chaudhry, F. Cubadda, D. Gott, A. Oomen, S. Weigel, M. Karamitrou, R. Schoonjans and A. Mortensen, Guidance on the risk assessment of the application of nanoscience and nanotechnologies in the food and feed chain: Part 1, human and animal health, EFSA J., 2018, 16(7), e05327 Search PubMed
. - H. E. Rosa, G. N. T. Gabriela, Z. H. Elvis and A. B. Patricia, Solid Lipid Nanoparticles (SLN), Nanocomposite Materials for Biomedical and Energy Storage Applications, IntechOpen, 2022, DOI:10.5772/intechopen.102536
. - C. I. García-Betanzos, H. Hernández-Sánchez, D. Quintanar-Guerrero, A. Del Real L and M. de la Luz Zambrano-Zaragoza, The Evaluation of Mechanical, Thermal, Optical and Microstructural Properties of Edible Films with Solid Lipid Nanoparticles-Xanthan Gum Stored at Different Temperatures and Relative Humidities, Food Bioproc. Tech., 2016, 9(10), 1756–1768 CrossRef
. - M. L. Zambrano-Zaragoza, E. Mercado-Silva, P. Ramirez-Zamorano, M. A. Cornejo-Villegas, E. Gutiérrez-Cortez and D. Quintanar-Guerrero, Use of solid lipid nanoparticles (SLNs) in edible coatings to increase guava (Psidium guajava L.) shelf-life, Food Res. Int., 2013, 51(2), 946–953 CrossRef CAS
. - V. Miranda-Linares, P. Escamilla-Rendón, A. Del Real-López, R. M. González-Reza and M. L. Zambrano-Zaragoza, Solid lipid nanoparticles based edible coating for saladette tomato preservation, Acta Hortic., 2018, 1194, 305–312 CrossRef
. - V. Wiedenmann, K. Oehlke, U. van der Schaaf, H. M. Koivula, K. S. Mikkonen and H. P. Karbstein, Emulsifier Composition of Solid Lipid Nanoparticles (SLN) Affects Mechanical and Barrier Properties of SLN-Protein Composite Films, J. Food Sci., 2019, 84(12), 3642–3652 CrossRef CAS PubMed
. - M. L. Zambrano-Zaragoza, D. Quintanar-Guerrero, A. Del Real, R. M. González-Reza, M. A. Cornejo-Villegas and E. Gutiérrez-Cortez, Effect of Nano-Edible Coating Based on Beeswax Solid Lipid Nanoparticles on Strawberry's Preservation, Coatings, 2020, 10(3), 253 CrossRef CAS
. - H. Cui, L. Yuan, W. Li and L. Lin, Edible film incorporated with chitosan and Artemisia annua oil nanoliposomes for inactivation of Escherichia coli O157:H7 on cherry tomato, Int. J. Food Sci. Technol., 2017, 52(3), 687–698 CrossRef CAS
. - C. Wu, L. Wang, Y. Hu, S. Chen, D. Liu and X. Ye, Edible coating from citrus essential oil-loaded nanoemulsions: physicochemical characterization and preservation performance, RSC Adv., 2016, 6(25), 20892–20900 RSC
. - L. Salvia-Trujillo, M. A. Rojas-Graü, R. Soliva-Fortuny and O. Martín-Belloso, Use of antimicrobial nanoemulsions as edible coatings: Impact on safety and quality attributes of fresh-cut Fuji apples, Postharvest Biol. Technol., 2015, 105, 8–16 CrossRef CAS
. - I. Sánchez-Ortega, B. E. García-Almendárez, E. M. Santos-López, L. R. Reyes-González and C. Regalado, Characterization and antimicrobial effect of starch-based edible coating suspensions, Food Hydrocolloids, 2016, 52, 906–913 CrossRef
. - D. J. McClements, Edible lipid nanoparticles: Digestion, absorption, and potential toxicity, Prog. Lipid Res., 2013, 52(4), 409–423 CrossRef CAS PubMed
. - Q. Chaudhry, et al., Applications and implications of nanotechnologies for the food sector, Food Addit. Contam.: Part A, 2008, 25(3), 241–258 CrossRef CAS PubMed
. - J. Jiang, G. Oberdörster and P. Biswas, Characterization of size, surface charge, and agglomeration state of nanoparticle dispersions for toxicological studies, J. Nanopart. Res., 2009, 11(1), 77–89 CrossRef CAS
. - Ö. Tarhan, Safety and regulatory issues of nanomaterials in foods, in Handbook of Food Nanotechnology, Elsevier, 2020, pp. 655–703 Search PubMed
. - S. A. O. Adeyeye and T. J. Ashaolu, Applications of nano-materials in food packaging: A review, J. Food Process. Eng., 2021, 44(7), 22 Search PubMed
. - J. M. Lourtioz, M. Lahmani, C. Dupas Haeberlin and P. Hesto, Nanosciences and Nanotechnology Evolution or Revolution, 2021 Search PubMed
. - J. C. Hannon, J. P. Kerry, M. Cruz-Romero, S. Azlin-Hasim, M. Morris and E. Cummins, Assessment of the migration potential of nanosilver from nanoparticle-coated low-density polyethylene food packaging into food simulants, Food Addit. Contam.: Part A, 2015, 1–12 Search PubMed
. - G. Singhal, R. Bhavesh, K. Kasariya, A. R. Sharma and R. P. Singh, Biosynthesis of silver nanoparticles using Ocimum sanctum (Tulsi) leaf extract and screening its antimicrobial activity, J. Nanopart. Res., 2011, 13(7), 2981–2988 CrossRef CAS
. - S. Das, L. Jagan, R. Isiah, B. Rajesh, S. Backianathan and J. Subhashini, Nanotechnology in oncology: Characterization and in vitro release kinetics of cisplatin-loaded albumin nanoparticles: Implications in anticancer drug delivery, Indian J. Pharmacol., 2011, 43(4), 409 CrossRef CAS PubMed
. - U. FDA, Inventory of Effective Food Contact Substance (FCS) Notifications, 2018 Search PubMed
. - Part 676-unified and combined state plans under title i of the workforce innovation and opportunity act contents §676, [online]. available: https://www.ecfr.gov/cgi-bin/text-idx?SID=325e94d7fdb1ce2b1f87c574bccc8ab9%26mc=true%26node=pt20.4.676%26rgn=div5.
- FDA Food Additive Status, “Food additive status list”, 2006.
- E. Jagtiani, Advancements in nanotechnology for food science and industry, Food Front., 2022, 3(1), 56–82 CrossRef
. - V. Amenta, et al., Regulatory aspects of nanotechnology in the agri/feed/food sector in EU and non-EU countries, Regul. Toxicol. Pharmacol., 2015, 73(1), 463–476 CrossRef PubMed
. - M. Cushen, J. Kerry, M. Morris, M. Cruz-Romero and E. Cummins, Migration and exposure assessment of silver from a PVC nanocomposite, Food Chem., 2013, 139(1–4), 389–397 CrossRef CAS PubMed
.
|
This journal is © The Royal Society of Chemistry 2024 |
Click here to see how this site uses Cookies. View our privacy policy here.