DOI:
10.1039/D3NA01145C
(Communication)
Nanoscale Adv., 2024,
6, 1374-1379
The structure–activity relationship of copper hydride nanoclusters in hydrogenation and reduction reactions†
Received
24th December 2023
, Accepted 1st February 2024
First published on 1st February 2024
Abstract
Copper hydrides are highly active catalysts in hydrogenation reactions and reduction processes. Three Stryker-type copper hydride nanoclusters (NCs), [(TPP)CuH]6, [(TCP)CuH]6 and [(TOP)CuH]6 (TPP = triphenylphosphine, TCP = tricyclohexylphosphine and TOP = tri-n-octylphosphine), were synthesized in this study. Due to variations in the electron-donating properties of the phosphine ligands, the UV-visible absorption spectra of the three NCs exhibited notable distinctions. The influence of the phosphine ligands on the effectiveness of the NCs as hydride sources in hydrogenation processes, as well as on the applicability as homogeneous catalysts for reduction reactions, was systematically studied. Due to the highest electron-donating properties of the TOP ligand, [(TOP)CuH]6 was found to exhibit superior performance in both hydrogenation reactions and catalytic reduction reactions. Moreover, these hydrophobic NCs worked well as heterogeneous catalysts in the reduction of 4-nitrophenol.
Introduction
Metal nanoclusters (NCs) have emerged as a noteworthy class of nanomaterials, showcasing distinct properties attributable to the quantum confinement effect when compared to their nanoparticle counterparts.1–4 Wet chemistry approaches enable the preparation of metal NCs under ambient pressure on a gram scale.5,6 Typically, this synthesis involves the reduction of metal salts or precursors in the presence of protecting ligands.7 Due to the strong interaction between metal ions and ligands, strong reducing agents like sodium borohydride are commonly employed.8 The structures of ligand-protected metal NCs can be solved by single crystal X-ray diffraction (XRD).9,10 Following up on the breakthrough of Au102, an increasing number of gold11–15 and silver NCs16–19 have been isolated and structurally characterized. These NCs exhibit a characteristic structure comprising a metal core shielded by a monolayer of metal–ligand complexes.20,21 The overall oxidation state of gold and silver falls between zero and plus one, tending closer to zero.22 With a growing number of gold and silver NCs being reported, it is now feasible to explore structure–property relationships at the atomic level.23 In the case of copper systems, the scenario is somewhat different. In addition to introduced organic ligands, hydrides are often identified in copper NCs.24 Locating these hydride ligands using XRD poses challenges due to the weak diffraction of hydrogen.25 The advancement of neutron diffraction has facilitated the identification and location of hydrides in copper NCs.26 Consequently, it was discovered that many copper NCs are in fact copper hydride NCs.27 The overall oxidation state of copper remains between zero and plus one, albeit leaning closer to plus one.28,29
Copper hydrides have become widely used in organic synthesis and catalysis. They are a good source of hydrides in stoichiometric reactions.30 Alternatively, catalytic reductions and hydrogenation reactions can be performed in the presence of an additional reducing agent, ensuring the continuous regeneration of copper hydride NCs during the reaction.31 One of the most well-known copper hydride NCs is the hexametric [(TPP)CuH]6 (TPP = triphenylphosphine), commercially available as Stryker's reagent.32 This hexametric NC exhibits notable regioselectivity and chemoselectivity, particularly in the hydrogenation of α,β-unsaturated ketones and aldehydes.33 In addition to triphenylphosphine, the hexametric NC can be synthesized using various phosphine ligands. For instance, tri(p-tolyl)phosphine and tris(4-methoxy)phenyl phosphine34 have been employed to investigate their impact on the electron transfer ability of copper hydride clusters ([CuH]6). Despite these efforts, systematic studies exploring the ligand effect on the reactivity of [CuH]6, especially considering factors such as steric hindrance33 and electronic structures,34 are scarce.35 The present study utilizes different [CuH]6 NCs protected by aliphatic and aromatic phosphines as a model system to comprehensively examine how the ligand influences the catalytic properties of the respective NC. The study reveals that the phosphine ligand with high electron-donating properties plays an important role in both stoichiometric and catalytic reactions. We anticipate that the results presented here will serve as instructive guidance for the future design of NCs with enhanced catalytic properties.
Experimental
Chemicals
Copper(II) acetate anhydrous (Cu(OAc)2, 98%) was purchased from Alfa Aesar. Triphenylphosphine (TPP, 99%), tricyclohexylphosphine (TCP, 98%), tri-n-octylphosphine (TOP, 97%), 1,1,3,3-tetramethyldisiloxane (TMDSO, 99%), benzene-d6 (C6D6, 99 atom% D), phenylacetylene (98%), sodium borohydride (NaBH4, 98%) and 4-nitrophenol (4-NP, 99%) were purchased from ABCR. O,O,O-1,3,5-trimethylresorcinol (98%) was purchased from Apollo Scientific. Toluene (99.5%), acetonitrile (99.9%), methanol (MeOH, 99.9%), and ethanol (EtOH, 99.9%) were purchased from Carl Roth.
Synthesis of [CuH]6 nanoclusters
All procedures for the synthesis of [CuH]6 were performed in an argon-filled glovebox. Cu(OAc)2 (0.186 g, 1 mmol), TPP (0.525 g, 2 mmol), and 1,1,3,3-tetramethyldisiloxane (1.2 mmol, 0.22 mL) were stirred in anhydrous toluene (2 mL) for 1 h.36 The colour of the reaction mixture changed from blue to green and then to dark red. The solution was filtered and described for [(TPP)CuH]6 (Cu6-1). The synthesis of [(TCP)CuH]6 (Cu6-2) and [(TOP)CuH]6 (Cu6-3) closely mirrored that of Cu6-1, with the only difference being the utilization of TCP or TOP in place of TPP, respectively. The hydride of Cu6-2 and Cu6-3 was obviously found in the 1H NMR spectra. More detailed information can be known in the ESI.†
Characterization methods
UV-vis spectra were recorded on 8453 UV-visible Spectroscopy systems from Agilent with argon purging. ATR-FTIR was recorded inside an Ar-filled glovebox using an Alpha spectrometer (Bruker) with an Alpha-P (Bruker) ATR unit. Nuclear magnetic resonance (NMR) spectra were collected on a Bruker Avance II 300 MHz at room temperature.
Hydrometallation of alkynes using [CuH]6
In the glovebox, phenylacetylene (4.6 × 10−3 mmol, 1 equiv.) was mixed with [CuH]6 (5.6 × 10−3 mmol, 1.2 equiv.) in C6D6 (520 μL). The solution was stirred vigorously for 1 h and the color of it changed from dark red to light yellow. The vessel was vented to air, and stirring over silica gel precipitated the Cu byproducts. The yield of styrene was determined from the 1H NMR spectrum (300 MHz, C6D6) with an internal stranded (O,O,O-1,3,5-trimethylresorcinol). Methanol (2.47 × 10−3 mmol, 0.5 equiv.) and TMDSO (2.7 × 10−2 mmol, 5.9 equiv.) were additionally added to the system separately for the control study.
Heterogeneous catalytic reduction of 4-nitrophenol
A water solution of 4-NP (1 ml, 0.1 mM) with NaBH4 (2.5 mg, 6.48 × 10−2 mmol) and [CuH]6 (1.02 × 10−3 mmol) was mixed, and the mixture was stirred for 5 min at room temperature. The intensity of the 4-NP absorption peak centered at 400 nm was monitored as a function of time to follow the reduction of 4-NP.
Computational methods
In all the calculations, the protecting ligands of all the nanoclusters were simplified as –P(CH3)3 groups, which had been proved to be an effective way to reduce the computational complexity. The geometric structure was optimized by the Perdew–Burke–Ernzerhof hybrid functional (PBE0) method with Grimme's BJ-damped variant of DFT-D3 empirical dispersion, while the all-electron def2-SVP basis set was used for all the atoms. Time-dependent density functional theory calculations were performed by setting the effective core potential LANL2DZ on all the copper atoms. Orbital composition analysis was performed by the Mulliken method using the Multiwfn 3.8 program.37 All the calculations were performed using the ORCA package.38
Results and discussion
It's reported in a Pd-catalysed system that phosphine ligands play a crucial role in determining the reactivity and selectivity.39 The stereoselectivity and regioselectivity of the catalytic hydrogenation of ketones, α,β-unsaturated ketones, and aldehydes, facilitated by soluble copper(I) hydride catalysts, have also been extensively investigated by Stryker and co-workers.31–33 However, the investigations mainly focused on the impact of extra phosphine ligands introduced additionally into the reaction. It is noteworthy that in their studies, the primary catalyst remains TPP-protected [CuH]6. Tolman et al. introduced a methodology to clarify the electron donor–acceptor characteristics of phosphine ligands, wherein a lower carbonyl stretching frequency of the ligand indicates a strong electron-donating ability.40 Their findings demonstrated that, in contrast to aromatic compounds, aliphatic compounds possess a heightened electron-donating capability. Specifically, linear compounds with aliphatic structures exhibit superior electron-donating properties, which is correlated to the increase of the aliphatic chain length. Hence, the influence of coordinating phosphine ligands on the reactivity of [CuH]6 has captivated our attention. Besides TPP, two aliphatic phosphines, namely tricyclohexylphosphine (TCP) and tri-n-octylphosphine (TOP), were chosen in this study. Fig. 1 illustrates the structural models of these phosphine ligands.
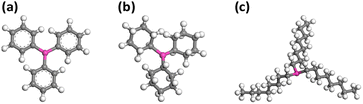 |
| Fig. 1 Schematic illustration of the (a) TPP, (b) TCP, and (c) TOP phosphorus ligands of [CuH]6. Colour legend: pink P; grey, C; white, H. | |
Cu6-1 was synthesized using 1,1,3,3-tetramethyldisiloxane, following the reported procedure in the literature. The synthesis of Cu6-2 and Cu6-3 mirrored that of Cu6-1. 31P NMR spectra in C6D6 indicate that the set of [CuH]6 NCs were of high purity with characteristic singlets at 8.66, 24.07, and −6.93 ppm, respectively (Fig. S1†). The variations in the P chemical shifts were ascribed to the different phosphine ligands and their coordination with copper.41 The attenuated total reflection-infrared (ATR-IR) experiments on [CuH]6 are depicted in Fig. S2.† Taking Cu6-1 as an example, the distinct absorption peak at 1435 cm−1 is attributed to the characteristic vibration of P–Ar. Additionally, the confirmation of aryl groups in Cu6-1 was established through absorptions located at 747 cm−1 and 3053 cm−1. These absorptions are attributed to the out-of-plane deformations of C–H and stretching vibrations of
C–H, respectively, further confirming the presence of TPP in Cu6-1.42,43 In Cu6-2 and Cu6-3 NCs, distinct vibrational bands around 1100 cm−1 were attributed to the C–P asymmetric stretching vibration.44 The above results suggest that when phosphine ligands are coordinated to copper, the ligand backbone structures underwent no apparent changes.
Fig. 2 shows the UV-vis absorption spectra illustrating various absorption characteristics of [CuH]6 NCs in toluene. Notably, Cu6-1 exhibited an distinctive absorption band centred at approximately 524 nm, consistent with previous findings.34 The spectrum of Cu6-2 was found featureless. Cu6-3 exhibits an absorption peak around 515 nm, shifted slightly compared to Cu6-1. The crystal structure of Cu6-1 is composed of a octahedron copper core with six long and six short Cu–Cu distances.45 The H ligands were assumed to be located on the six long Cu–Cu edges.46 Derived from Cu6-1, the schemes of Cu6-2 and Cu6-3 were illustrated.
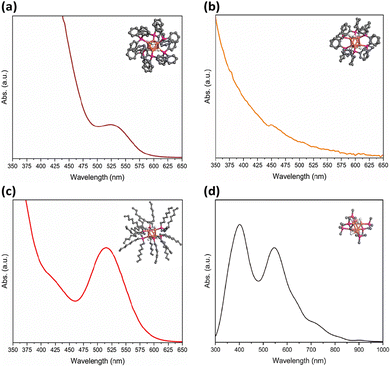 |
| Fig. 2 The experimental UV-vis absorption spectra of (a) Cu6-1, (b) Cu6-2, (c) Cu6-3 and (d) TDDFT simulated absorption spectrum of [CuH]6 (the Gaussian width was set to 60 nm). Insets show their structures. Colour legend: orange, Cu; pink, P; grey, C; white, H. Hydrogen atoms on ligands are omitted for clarity. | |
To gain a better understanding of the electronic structure of [CuH]6, time-dependent density functional theory (TDDFT) calculations were performed. The theoretical absorption spectrum successfully reproduced one of the experimental peaks at around 2.28 eV (Fig. 2d and S3†). Kohn–Sham (KS) orbital analysis indicates that this absorption predominantly corresponds to the LUMO + 5 ← HOMO − 6 interband transition (Fig. 3, Tables S1 and S2†). Atomic orbital composition analysis suggests that the contributions from P atoms remain significant regardless of the growing contribution of the Cu 3d orbital from HOMO − 6 to LUMO + 5. Since the substituents on the phosphine ligand have great impact on the P atom, their influence on the electronic structure of the NC should also be non-negligible.
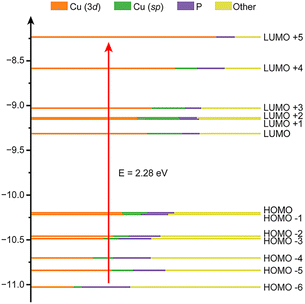 |
| Fig. 3 Kohn–Sham (KS) orbitals of the NCs obtained from time-dependent density functional theory (TDDFT) calculations. The different colours in the energy levels show the contribution of the atomic orbitals. | |
To investigate the impact of the ligands on the [CuH]6-driven hydrogenation reaction, phenylacetylene hydrogenation was considered as a model reaction (Scheme 1). To our surprise, the three NCs lead to the selective formation of styrene. Although the three NCs showed a similar selectivity, their activities were different. When Cu6-3 was used, 63.8% of phenylacetylene was converted to styrene. This value was 44.4% and 57% for Cu6-1 and Cu6-2, respectively. It is reported that MeOH can react with the hydride in NaBH4, forming alkoxyborohydride.47 To verify this, we introduced MeOH into the system. As expected, a notable inhibition effect was observed in Fig. 4. The generation of styrene decreased to 18.9%, 32.6%, and 41.3%, respectively. This reduction on activity is possibly due to the consumption of the hydride species associated with Cu by MeOH.48 Similar to MeOH, EtOH resulted in a reduction in the generation of styrene (Fig. S4†). This conclusion well agrees with the previous analysis. In addition to serving as a source of hydrides, [CuH]6 NCs can also serve as catalysts when other reducing agents are present, ensuring the continual regeneration of [CuH]6 during the reaction. When TMDSO as a source of hydrides was introduced, the selective reduction of phenylacetylene was promoted. 55.4%, 62.0%, and 90.0% of styrene were found after 1 hour in Cu6-1, Cu6-2 and Cu6-3 catalysis systems, respectively. In both hydrogenation and catalytic reduction reactions, Cu6-3 exhibited superior activity. This could be attributed to the high electron-donating properties of the TOP ligand. The stronger electrostatic force increases the Cu–H bond length, and consequently, enhanced the catalytic activity of copper NCs.49 We further investigated the changes of the residue after the hydrometallation reaction. While the ATR-IR spectrum showed no apparent change (Fig. S5†), the 31P NMR study, as displayed in Fig. S6,† revealed slight shifts that could be attributed to the altered relative positions of the phosphine ligands and their coordination with copper. Notably, new narrow P peaks at 62.74 ppm and 58.49 ppm were found in Cu6-2 and Cu6-3 systems, respectively. The formation of these catalytically active species during the hydrogenation process could also be attributed to the weaker interaction between Cu and hydrides in Cu6-2 and Cu6-3.
 |
| Scheme 1 Selective hydrogenation of phenylacetylene by [CuH]6. | |
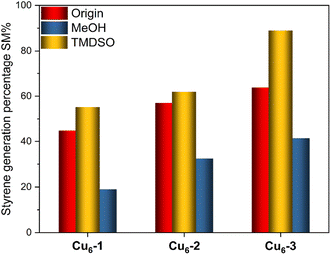 |
| Fig. 4 Hydrometallation of phenylacetylene using [CuH]6. | |
To further investigate the possibility of using [CuH]6 as a heterogeneous catalyst, we studied the reduction of 4-nitrophenol (4-NP) to 4-aminophenol (4-AP) by NaBH4 as a model reaction. The NCs were dispersed into the solution of 4-NP and NaBH4. In the Cu6-3 system, 4-NP was completely reduced to 4-AP after 4 minutes. The conversion rate is 49% and 62% for Cu6-1 and Cu6-2 systems, respectively (Table S3†). Utilizing UV-vis absorption spectroscopy, the reduction process was monitored by determining the intensity variation of the 400 nm peak associated with 4-NP. As the catalytic reaction progressed in the presence of Cu6-3, the intensity of the 400 nm peak exhibited a rapid decline, becoming entirely reduced after 4 minutes (Fig. 5a). This observation implies the complete conversion of 4-NP to 4-AP (λmax = 295 nm in water). The reaction rate constant was determined to be 0.84 min−1 (Fig. 5b), which is much higher than that of other reported coper hydride NCs (0.5 min−1 for Cu11(Tf-dpf)6(OAc)2H3 and 0.007 min−1 for Cu12(Tf-dpf)6(OAc)3 H3).26 Reusability tests were also carried out on conversion of 4-NP, as shown in Fig. S7 and Table S4.†Cu6-3 showed remarkably high stability and recyclability over four cycles of 4-NP reduction. Cu6-3 exhibits promise as a catalyst in a variety of applications.
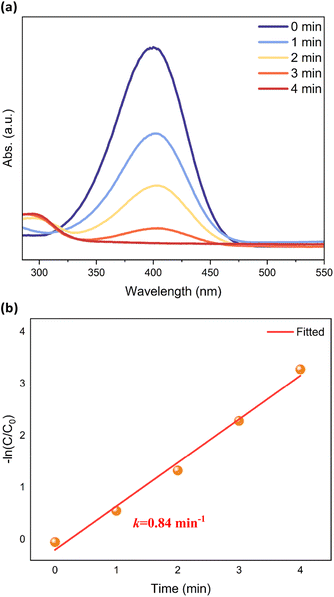 |
| Fig. 5 (a) UV-vis spectra showing the gradual reduction of 4-NP in the presence of Cu6-3 and (b) plot of −ln(C/C0) versus time. | |
Conclusions
In summary, three different phosphine ligands were successfully employed in the synthesis of copper hydride NCs, [CuH]6. The distinct electron-donating properties of the three ligands resulted in the different reactivities of the three NCs. Due to the highest electron-donating properties of the TOP ligand, [(TOP)CuH]6 was found to exhibit superior performance in both stoichiometric hydrogenation and catalytic reduction of phenylacetylene. Moreover, the NCs served as an effective heterogeneous catalyst in the reduction of 4-nitrophenol by NaBH4. These findings contribute to a deep understanding of the ligand effect on the performance of copper hydride NCs. We hope it could serve as instructive guidance for the future design of NCs with enhanced catalytic properties.
Author contributions
Y. W.: conceptualization. X. Z. synthesized all the [CuH]6 materials and performed all the physiochemical characterization and hydrogenation experiments. Y. L. and Y. S. conducted DFT calculations. W. M. provided UV-vis tests. Data plotting and analysis were performed by X. Z., W. M., and Y. L. The manuscript was drafted by X. Z. and Y. W. and then revised by all the co-authors.
Conflicts of interest
There are no conflicts to declare.
Acknowledgements
The authors would like to acknowledge Prof. Nicola Pinna's support and help. We are grateful to the German Research Foundation (DFG, Project 456075917) for the financial support of this work. We appreciate Prof. Kallol Ray and Wanli Ma for UV-vis support and helpful discussion. X. Z. acknowledges the fellowship from the China Scholarship Council (CSC, No. 202206420020). Y. L. acknowledges the fellowship from the China Scholarship Council (CSC, No. 201908420279). Y. S. acknowledges the National Natural Science Foundation of China (No. 12174045) for financial support.
References
- X. Zou, X. Kang and M. Zhu, Chem. Soc. Rev., 2023, 52, 5892–5967 RSC.
- Y. Liu, Z. Li, X. Liu, N. Pinna and Y. Wang, Nanoscale Horiz., 2023, 8, 1435–1439 RSC.
- Y. Wang, Z. Liu, A. Mazumder, C. G. Gianopoulos, K. Kirschbaum, L. A. Peteanu and R. Jin, J. Am. Chem. Soc., 2023, 145, 26328–26338 CrossRef CAS PubMed.
- N. Zhang and Y. Xiong, Advanced Sensor and Energy Materials, 2023, 100047 CrossRef.
- Y. Lu and W. Chen, Chem. Soc. Rev., 2012, 41, 3594–3623 RSC.
- Y. Wang, X. Liu, R. Wang, B. Cula, Z.-N. Chen, Q. Chen, N. Koch and N. Pinna, J. Am. Chem. Soc., 2021, 143, 9595–9600 CrossRef CAS PubMed.
- M. R. Friedfeld, J. L. Stein, A. Ritchhart and B. M. Cossairt, Acc. Chem. Res., 2018, 51, 2803–2810 CrossRef CAS PubMed.
- Y. Wang, X. Liu, Q. Wang, M. Quick, S. A. Kovalenko, Q. Chen, N. Koch and N. Pinna, Angew. Chem., Int. Ed., 2020, 132, 7822–7828 CrossRef.
- F. Hu, R. He, Z. Guan, C. Liu and Q. Wang, Angew. Chem., Int. Ed., 2023, 62, e202304134 CrossRef CAS PubMed.
- H. Shen, Q. Wu, M. S. A. Hazer, X. Tang, Y. Han, R. Qin, C. Ma, S. Malola, B. K. Teo, H. Häkkinen and N. Zheng, Chem, 2022, 8, 2380–2392 CAS.
- P. D. Jadzinsky, G. Calero, C. J. Ackerson, D. A. Bushnell and R. D. Kornberg, Science, 2007, 318, 430–433 CrossRef CAS PubMed.
- X. Liu, E. Wang, M. Zhou, Y. Wan, Y. Zhang, H. Liu, Y. Zhao, J. Li, Y. Gao and Y. Zhu, Angew. Chem., Int. Ed., 2022, 61, e202207685 CrossRef CAS PubMed.
- F. Hu, Z. Guan, G. Yang, J. Wang, J. Li, S. Yuan, G. Liang and Q. Wang, J. Am. Chem. Soc., 2021, 143, 17059–17067 CrossRef CAS PubMed.
- X. Yu, Y. Sun, W.-w. Xu, J. Fan, J. Gao, X. Jiang, Y. Su and J. Zhao, Nanoscale Horiz., 2022, 7, 1192–1200 RSC.
- X. Yu, Y. Su, W.-w. Xu and J. Zhao, J. Phys. Chem. Lett., 2021, 12, 2312–2319 CrossRef CAS PubMed.
- A. Ghosh, O. F. Mohammed and O. M. Bakr, Acc. Chem. Res., 2018, 51, 3094–3103 CrossRef CAS PubMed.
- Y. Horita, S. Hossain, M. Ishimi, P. Zhao, M. Sera, T. Kawawaki, S. Takano, Y. Niihori, T. Nakamura, T. Tsukuda, M. Ehara and Y. Negishi, J. Am. Chem. Soc., 2023, 154, 23533–23540 CrossRef PubMed.
- C. Zhu, J. Xin, J. Li, H. Li, X. Kang, Y. Pei and M. Zhu, Angew. Chem., Int. Ed., 2022, 134, e202205947 CrossRef.
- Z. Wang, R. K. Gupta, F. Alkan, B.-L. Han, L. Feng, X.-Q. Huang, Z.-Y. Gao, C.-H. Tung and D. Sun, J. Am. Chem. Soc., 2023, 145, 19523–19532 CrossRef CAS PubMed.
- Y. Liu, E. Wierzbicka, A. Springer, N. Pinna and Y. Wang, J. Phys. Chem. C, 2022, 126, 1778–1784 CrossRef CAS.
- Y. Wang, X. H. Liu, S. A. Kovalenko, Q. Y. Chen and N. Pinna, Chem. – Eur. J., 2019, 25, 4814–4820 CrossRef CAS PubMed.
- W.-Q. Shi, L. Zeng, R.-L. He, X.-S. Han, Z.-J. Guan, M. Zhou and Q.-M. Wang, Science, 2024, 383, 326–330 CrossRef CAS PubMed.
- F. Yu, Y. Zhu, Y. Gao, R. Wang, W. Huang, Y. Gao and Z. Wang, Nano Res., 2022, 15, 8665–8672 CrossRef CAS.
- Q. Tang, Y. Lee, D. Li, W. Choi, C. Liu, D. Lee and D. Jiang, J. Am. Chem. Soc., 2017, 139, 9728–9736 CrossRef CAS PubMed.
- J. Liao, R. P. Brocha Silalahi, T. Chiu and C. Liu, ACS Omega, 2023, 8, 31541–31547 CrossRef CAS PubMed.
- C. Liu, S. Yuan, S. Wang, Z. Guan, D. Jiang and Q. Wang, Nat. Commun., 2022, 13, 2082 CrossRef CAS PubMed.
- S. Yuan, H. Luyang, Z. Lei, X. Wan, J. Li and Q. Wang, Chem. Commun., 2021, 57, 4315–4318 RSC.
- L. Liu, Z. Wang, Z. Wang, R. Wang, S. Zang and T. C. W. Mak, Angew. Chem., Int. Ed., 2022, 61, e202205626 CrossRef CAS PubMed.
- K. K. Chakrahari, J. Liao, R. P. B. Silalahi, T. Chiu, J. Liao, X. Wang, S. Kahlal, J. Saillard and C. Liu, Small, 2021, 17, 2002544 CrossRef CAS PubMed.
- P. Chiu and S. K. Leung, Chem. Commun., 2004, 2308–2309 RSC.
- W. S. Mahoney, D. M. Brestensky and J. M. Stryker, J. Am. Chem. Soc., 1988, 110, 291–293 CrossRef CAS.
- J. F. Daeuble, C. McGettigan and J. M. Stryker, Tetrahedron Lett., 1990, 31, 2397–2400 CrossRef CAS.
- J. Chen, J. F. Daeuble, D. M. Brestensky and J. M. Stryker, Tetrahedron, 2000, 56, 2153–2166 CrossRef CAS.
- M. S. Eberhart, J. R. Norton, A. Zuzek, W. Sattler and S. Ruccolo, J. Am. Chem. Soc., 2013, 135, 17262–17265 CrossRef CAS PubMed.
- X. Duan, Y. Han, B. Zhu and Y. Gao, Mater. Today Catal., 2023, 3, 100032 CrossRef.
- D. Lee and J. Yun, Tetrahedron Lett., 2005, 46, 2037–2039 CrossRef CAS.
- T. Lu and F. Chen, J. Comput. Chem., 2012, 33, 580–592 CrossRef CAS PubMed.
- F. Neese, Wiley Interdiscip. Rev. Comput. Mol. Sci., 2012, 2, 73–78 CrossRef CAS.
- I. del Río, N. Ruiz, C. Claver, L. A. van der Veen and P. W. van Leeuwen, J. Mol. Catal. A: Chem., 2000, 161, 39–48 CrossRef.
- C. A. Tolman, J. Am. Chem. Soc., 1970, 92, 2953–2956 CrossRef CAS.
- J. H. Letcher and J. R. Van Wazer, J. Chem. Phys., 1966, 44, 815–829 CrossRef CAS.
- L. Shi, B. Li, S. Lu, D. Zhu and W. Li, Appl. Organomet. Chem., 2009, 23, 379–384 CrossRef CAS.
- X. Zheng, T. Xu, X. Kang, Y. Xing, Y. Cao and X. Gui, J. Chem. Eng., 2022, 431, 134088 CrossRef CAS.
- P. Astolfi, M. Pisani, E. Giorgini, B. Rossi, A. Damin, F. Vita, O. Francescangeli, L. Luciani and R. Galassi, Nanomater, 2020, 10, 1851 CrossRef CAS PubMed.
- R. C. Stevens, M. R. McLean, R. Bau and T. F. Koetzle, J. Am. Chem. Soc., 1989, 111, 3472–3473 CrossRef CAS.
- E. L. Bennett, P. J. Murphy, S. Imberti and S. F. Parker, Inorg. Chem., 2014, 53, 2963–2967 CrossRef CAS PubMed.
- C. P. Prasanth, E. Joseph, A. Abhijith, D. S. Nair, I. Ibnusaud, J. Raskatov and B. Singaram, J. Org. Chem., 2018, 83, 1431–1440 CrossRef CAS PubMed.
- V. Dalla, J. Catteau and P. Pale, Tetrahedron Lett., 1999, 40, 5193–5196 CrossRef CAS.
- F. Bertini, M. Glatz, N. Gorgas, B. Stöger, M. Peruzzini, L. F. Veiros, K. Kirchner and L. Gonsalvi, Chem. Sci., 2017, 8, 5024–5029 RSC.
|
This journal is © The Royal Society of Chemistry 2024 |
Click here to see how this site uses Cookies. View our privacy policy here.