Release of TiO2 nanoparticles from painted surfaces in cold climates: characterization using a high sensitivity single-particle ICP-MS†
Received
21st August 2019
, Accepted 18th December 2019
First published on 19th December 2019
Abstract
Paints and coatings represent one of the major applications of TiO2 nanoparticles (NPs). While it has been previously shown that NPs are released from painted surfaces, there is still a lack of experimental data on their release rates under natural conditions and on the size distributions of the NPs following release. This study quantifies TiO2 NP release from painted surfaces under natural weathering conditions and identifies the main seasonal factors that contribute to increased NP release. First, an analytical methodology using a highly sensitive single particle inductively coupled plasma mass spectrometer (SP-ICP-MS) was developed that improved the size detection limit (SDL) of the technique down to <20 nm for TiO2 NPs. Precipitation (rain, snow) was collected after it came into contact with painted panels that were exposed to natural weathering. NPs that were released from the paint, as well as those pre-existing in the precipitation were thoroughly characterized with respect to their size distributions, particle number concentrations and total metal content. During the 10 week winter exposure, 3 × 1011 NP per m2 were released, corresponding to <0.001% of the TiO2 NP load on the panels, with most of the NPs found in the 20–60 nm range. Significantly fewer NPs were released during the summer than the winter, in spite of the fact that there was more precipitation in the summer. Controlled lab weathering experiments revealed that NP release was significantly enhanced for wet surfaces, particularly, when the samples underwent freeze–thaw cycles. The results also indicated that NP release and loss (i.e. through agglomeration, sedimentation or sorption, etc.) are dynamic processes that are a function of the physical and chemical properties of the external medium. Although NP release is a primary determinant in environmental risk, subsequent NP behavior leading to losses or re-suspension can be equally critical.
Environmental significance
With the widespread use of TiO2 NPs in paints, NP release from outdoor façade coatings into environmental systems is inevitable, necessitating their environmental risk assessment. Unfortunately, analytical data on the environmental release of NPs from painted surfaces – particularly, in cold climates – are limited. A highly sensitive sector-field inductively coupled plasma mass spectrometer was used to quantify the surface-release rates of TiO2 NPs under natural weathering conditions. In field and laboratory experiments, the main seasonal factors that contributed to increased NP release were identified. Although background concentrations of Ti containing nanoparticles were high, the study demonstrated that humid and freeze–thaw conditions relevant to the winter season increased NP release. In addition, it showed that paint-released NPs could be subsequently lost due to physicochemical processes, including agglomeration, sedimentation and sorption.
|
Introduction
Recent advances in nanotechnology have led to greater numbers of nano-enabled products and increased production volumes of engineered nanomaterials (ENMs).1 With the growing production and applications of ENMs, their release into the environment is inevitable, necessitating research into their environmental fate.2–4 TiO2 nanoparticles (NPs) are the most widely produced ENM on a mass basis.5–7 Among other applications, they are extensively used in paints and coatings, where they can provide UV protection and self-cleaning properties.8–10
While the use of TiO2 NPs in paints is on the rise and their release from aged painted surfaces is imminent, their reliable risk assessment remains a challenge in environmental systems.1,9,10 This is largely due to limited existing knowledge on their mass and number concentrations, measurements of their surface-release rates and information on their particle size distributions and persistence in the environment.5,11,12 Among the limited analytical data available, lab-controlled tests conducted in simple media have clearly shown significant NP leaching of painted surfaces, with some of the released NPs still embedded in the organic paint matrix.13,14 Olabarrieta et al.15 and Al-Kattan et al.16 demonstrated that TiO2 NPs were released from coatings weathered by flowing water, while Zhang et al.17 and Zuin et al.18 showed that static immersion in water could induce NP release.
Outdoor studies,13,19–21 while more difficult to control, are more appropriate for evaluating real-world NP release scenarios. For example, Kaegi et al.13,19 followed the release of Ti and Ag from a painted exterior façade over 1 year and concluded that much of the released metal was in nanoparticulate form, with the estimated primary particle sizes for TiO2 between 20 and 300 nm and Ag below 15 nm. To the best of our knowledge, outdoor studies investigating NP release in cold climates – where snow, freezing and freeze–thaw conditions are relevant – are not yet available.
Most of the above studies estimated release based on an increase in total metal concentrations in the waters that were in contact with the painted surface and confirmed the presence of NPs using microscopic data. In such cases, it is difficult to quantify to what extent NPs or dissolved metals were released. An additional difficulty in outdoor studies is the significant presence of the target elements in the incoming precipitation.22,23 Overall, NP analysis in complex waters is a challenging task,24–26 requiring accurate NP release determinations that can identify, size and quantify the NPs on a particle by particle basis. To that end, single-particle ICP-MS (SP-ICP-MS) is well suited to provide information on NP size distributions and number concentrations in natural waters.27,28 It is based on the ultrafast measurement and analysis of the transient ICP-MS signal. NPs typically generate high intensity signals (spikes, ca. 300–500 μs) that can be discriminated from a continuous (generally low-intensity) background, representative of the dissolved metals (and small NPs).29 While the technique is extremely sensitive for several metallic NPs (e.g. Ag, Au, Ce), the analysis of TiO2 is more challenging, due primarily to high levels of background interferences.24,25,29 For this reason, most of the reported size detection limits (SDL) for TiO2 NPs are above 50 nm,30–32 restricting characterization of the smaller NPs, which are thought to be of higher risk to both the environment and human health.33
Given this context, the goal of the study was to quantify the release of TiO2 NPs from nano-enhanced surfaces under natural weathering conditions. The study was designed to: (i) characterize release of TiO2 NPs by measuring NP concentrations and size distributions with time and (ii) explore the role of temperature fluctuations (i.e. freeze, thaw) and wet/dry conditions on NP release. Experiments were designed to provide insight into the release and persistence of these important NPs in the environment.
Materials and methods
Preparation of exposure panels
Untreated oak slats (0.64 cm thick × 6.4 cm wide) were cut to 8.4 cm long panels, which were primed and painted with a white paint advertised for its Nanoguard Technology (Behr Premium Plus Ultra Exterior Satin Enamel Ultra Pure white paint). A uniform coating was obtained by applying 15 mL per side to each panel and removing excess paint with an applicator (Bird Film Applicator, Inc, Washington, USA) in order to get a wet film thickness of 4 mm. Both sides of the panel were coated twice, and the paint was allowed to dry for at least two days before the addition of the next layer. The final dry paint loading on each panel was 400 ± 40 g m−2 (or 4.4 g per panel).
Outdoor weathering setup
For outdoor weathering experiments, replicate samples of the painted panels were placed vertically in pre-weighed, wide-mouth polypropylene containers (500 mL, Fisher Scientific). Containers were randomly ordered within plastic bins and placed outdoors (Fig. S1†) on a 4th floor roof (M. H. Wong building, McGill University, Montreal, Canada), where they were left uncovered. The base of the bin was lined with sand bags to prevent tipping. Samples were left undisturbed during the weathering period except when snowfall buried the containers. In those cases, excess snow was carefully scraped off the top of the containers to prevent overflow when the snow melted. Winter weathering experiments were conducted for 10 weeks beginning in mid-February (2018), while 7 weeks of summer experiments were initiated in early June (2018). At each experimental timepoint, four control samples (container containing no painted panel) and four samples (container containing painted panels) were sacrificed.
Indoor (controlled) weathering setup
For controlled weathering experiments, panels were placed in pre-weighed polypropylene containers (4 sample replicates), which were either filled with 380 mL of Milli-Q water (wet) or not (dry). Samples were then aged under room temperature (RT), freezing (FR), or freeze–thaw (FT) conditions. For FR and FT conditions, samples were placed in a −10 °C freezer. FT conditions consisted of repeated 48 h cycles in which samples were first allowed to freeze overnight (for 24 h), then removed from the freezer and placed in a closed box on the benchtop, where they were allowed to thaw (for 24 h). The experiment was conducted for a total of 42 d (21 FT cycles). At the end of the 42 d exposure, dry FR samples were soaked for 1 d in Milli-Q water, while wet FR samples were allowed to thaw (1 d). For the wet samples (both RT & FT), in addition to the 42 d timepoint, small samples (16–18 mL) were removed at intermediate timepoints during the exposure. The sampled volume was replaced with fresh Milli-Q water in order to maintain a constant exposure surface.
Sample preparation for SP-ICP-MS
For each timepoint, the outdoor and control containers to be tested were capped and all snow or ice was allowed to melt. The contents of the containers were then gently mixed, and the panels were carefully removed. Water was weighed, mixed, and then placed in a sonicator bath for 30 minutes (Branson Ultrasonic Cleaner, 5510R-DTH Model, 135 W, 42 kHz ± 6%). Following sonication, 8–10 mL of sample was filtered through a 0.45 μm, 33 mm diameter PVDF syringe filter that had been pre-rinsed with 12 mL Milli-Q water and 6 mL of sample. Filtration on this pore-size was performed to avoid blockage of the microflow nebulizers, without minimizing removal of the nanoparticles.
SP-ICP-MS analysis and data acquisition
All NP measurements were performed on a sector field ICP-MS (AttoM ES, Nu Instruments, UK), at low resolution (300), using single ion acquisition in fast-scan mode. Liquid samples were introduced through a micro-flow concentric glass nebulizer (free aspiration rate of 200 μL min−1 for 1 L min−1 Ar) into a quartz cyclonic spray chamber cooled at 4 °C. The enhanced sensitivity27,34 of the sector-field instrument (with respect to a quadrupole-based instrument) allowed us to analyse 49Ti, which has a lower natural abundance, but also fewer interferences. An optimized dwell time of 50 μs was used,35 with an acquisition time of 50 s, which resulted in ca. 106 datapoints per replicate. Sensitivity calibrations for 49Ti were based on ionic standards (High Purity Standards). Transport efficiency (TE) measurements were performed using a suspension of ultra-uniform 30 nm Au NPs (NanoComposix, AUXU30-1M), which were prepared daily at 50 ng L−1. TE measurements were validated with a second standard reference material (60 nm Au NPs, NIST8013).35 TE values ranged from 0.10–0.12 μL s−1 (3.4–3.8%). Standard additions were performed on ionic Ti standards (1, 5 and 10 μg L−1) and spikes of engineered TiO2 NPs (P25 Aeroxide, Evonik, Germany; nominal size 25 nm) in the rain or snow melt water, in order to assess possible matrix effects. Size calculations were performed using an assumed TiO2 density of 4.23 g cm−3 (i.e. rutile). Filtered samples were diluted 10–50× in order to ensure that the number of particle events was significant (>500 events), while minimizing the possibility for co-incident events (less than 10
000 events, or 1% of the total datapoints).
SP-ICP-MS data processing
SP-ICP-MS data was processed using NuQuant software version 2.2 (Nu Instruments, UK),27 based on the methodology described by Hadioui et al.35 and Shaw et al.34 In summary, data processing involved the identification of NP peaks, calculation of the local backgrounds and integration of the peaks. An average of the local peak backgrounds was used to calculate dissolved metal content. Values of the full-width at half-maximum (FWHM) were used to flag peak events that could potentially be co-incident NP peaks or background artifacts (i.e. very slender or very wide peaks with low intensity). Flagged events were visually inspected, and if necessary, the samples were diluted further.
Total metal analysis
Following the filtration of samples for SP-ICP-MS, aliquots of the filtered samples were added to polypropylene tubes containing 67–70% HNO3 (ultratrace grade, BDH Aristar Ultra) in order to achieve a final acid content of 20% v/v. Tubes were left for 16 h at 85 °C using a DigiPREP digestion system (SCP Science) and then diluted with Milli-Q water to obtain a final HNO3 content of 4% v/v, which was used for quantitative ICP-MS analysis. Given the well documented difficulties in digesting TiO2,36 these measurements should be operationally defined as acid-extractable Ti.
Results and discussion
Optimization of SP-ICP-MS for TiO2 NPs
In comparison to other prevalent NPs such as Ag, CeO2 and Au, detection and characterization of TiO2 NPs using SP-ICP-MS is significantly more challenging.30 This is primarily due to isobaric/polyatomic interferences that increase the background signal for 48Ti, which is of greatest natural abundance.37 To overcome these challenges, SP-ICP-MS was optimized35 using several strategies, including the use of 49Ti, the use of a magnetic sector ICP-MS and the use of short dwell times (50 μs) (for further details, refer to ESI†). The combination of high sensitivity, low background and improved NP peak resolution/recognition led to SDLs that were as low as 20 nm for TiO2 NPs in the snow and rain waters. Indeed, the particle size distribution (PSD) of TiO2 NPs (Fig. 1A) and the raw signal intensities corresponding to the smallest detected NP (Fig. 1B) are presented for NPs detected in a snow melt sample.
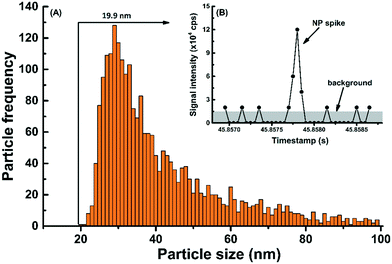 |
| Fig. 1 (A) Particle size distribution of Ti-containing NPs found in snow melt water, as measured with high-sensitivity magnetic-sector ICP-MS using a dwell time of 50 μs. (B) Raw signal intensity (cps) near the particle size detection limit. Particle diameters were calculated on the assumption that the particles were TiO2. | |
Verification of an absence of matrix effect is difficult with TiO2 NPs, given the difficulty to obtain monodisperse TiO2 standards. Therefore, several strategies were undertaken to verify and reduce matrix effects during the measurements of TiO2 NPs: (i) tests on the matrix effect were performed using ionic Ti; (ii) TiO2 NPs were measured following their spike into a natural rainwater matrix, which had a pre-existing NP background; (iii) SP-ICP-MS measurements were performed in media that were diluted at least 10×. Indeed, nearly identical recoveries (i.e. 102%) were obtained for a spike of ionic Ti measured in Milli-Q water or rainwater (Table S1†), suggesting that the matrix effect for 49Ti was minimal. When spiking TiO2 NPs (nominal size of 25 nm) into the rainwater, a recovery of 92% was attained, following correction for particle numbers in the unspiked rainwater (Fig. S2†). In that case, the slightly lower recoveries may have been caused by TiO2 losses following their agglomeration in the rainwater matrix.
Detection of Ti NPs in natural precipitation
Very early in the weathering study, it became clear that significant NP concentrations were being detected in the precipitation, prior to it coming in contact with the painted surfaces. Owing their presence to a variety of natural, urban or industrial sources, nano- and micron-sized particles are ubiquitous in the atmosphere, and they are especially important in urban and industrial areas subjected to pollution.22,23,38,39 Therefore, it was essential to accurately determine Ti NP concentrations in the natural precipitation in order to correct for their presence during the paint-release studies. Given that we have no a priori information on the full chemical composition of the Ti containing NPs, sizes have been calculated based upon the assumption that they are primarily TiO2. It is thus important to acknowledge that, for particles that are more likely to have multi-element compositions, such as natural nanominerals,22,38,40 actual particle sizes would be underestimated. On the other hand, for some particles, such as Magneli phase Ti (TixO2x−1), which are produced incidentally during coal combustion,22 sizes would be overestimated. Indeed, in this study, numerous metals, including Ti, Ce, Fe, La, Nd, U and Ag were found in particulate forms by SP-ICP-MS time-scan (Fig. S3†), with the results strongly indicating the presence of atmospheric NPs containing Ti (Fig. 2A). Micron-sized particles were also present in the precipitation; when comparing ICP-MS measurements on acidified samples measured before and after filtration, >90% of the particulate Ti was generally removed by a 0.45 μm membrane (based on total Ti mass balances). SP-ICP-MS measurements performed on the filtered samples showed that the remaining NPs had diameters with a mode around 30 nm and a size distribution extending from the SDL (20 nm) to beyond 100 nm (Fig. 2B). Snow and rain samples were analysed for both total Ti by ICP-MS and Ti NPs by SP-ICP-MS, with the results showing that significant numbers of Ti-containing NPs were found in the snow and rain samples. A subsequent experiment where Milli-Q water was left outdoors for 2 d under dry summer conditions also showed significant particle numbers (Fig. S4†), suggesting that dry deposition was an important source of the Ti NPs. This observation was also observed for a Milli-Q water sample exposed to indoor air (Fig. S5†). The ubiquitous presence of Ti NPs in the air demonstrates the importance of multiple control experiments in order to avoid false positives in the paint release experiments.
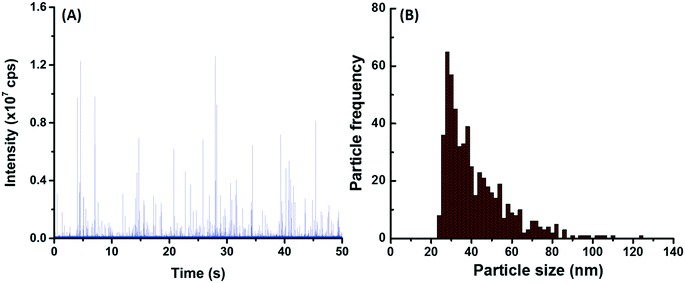 |
| Fig. 2 (A) Time-resolved SP-ICP-MS signal and the corresponding (B) particle size distribution obtained for Ti-containing NPs in snow melt (10× diluted). The snow was collected during 2 weeks in February 2018, and the measurements were performed by high-sensitivity magnetic-sector ICP-MS. Particle diameters were calculated on the assumption that particles were TiO2. | |
TiO2 NPs in paint
The water-based acrylic paint was tested for TiO2 NPs using SP-ICP-MS after dilution (2 × 107 × (w/w)) in Milli-Q water. The PSD for TiO2 extended from 20 nm (SDL) to beyond 200 nm, with most of the detected NPs around 150 ± 10 nm (mode) with a mean NP diameter of 131 ± 1 nm (Fig. S6A†). There were aprox. (6.8 ± 0.1) × 1015 NPs per kg-paint (i.e. below 250 nm) in the liquid paint, which corresponded to about 5.0% by weight. This measured NP fraction is in agreement with other reports for paints,5,8,16,41 where experimental or predicted TiO2 fractions are reported to be around 1–15% by weight.
TiO2 NP release due to weathering
Experimental containers with or without a painted panel were placed outdoors. In the control samples (i.e. without panels), NP numbers were significant and generally increased with time, in both the winter and summer sampling periods. The actual measured concentrations ranged from 8.1 × 105 NP per mL after two weeks to 1.2 × 107 NP per mL after 7 weeks in the winter and hovered around 2–4 × 106 NP per mL for 7 weeks during the summer. The observed increase in NP numbers is primarily attributed to cumulative NP deposition (directly via rain/snow or indirectly via dry deposition, see Fig. S4†), although part of the variation can be attributed to differences in the sample volumes at the time of collection (due to the timing of evaporation/precipitation events). When the data were normalized by exposure surface area of the painted panels, both total Ti and Ti NP (Fig. 3A and B, orange bars) consistently increased with time at an approximate rate of 9.2 μg-Ti per m2 per week (this corresponds to a rate of 30.9 μg-Ti per m2 per week when normalized by footprint area).
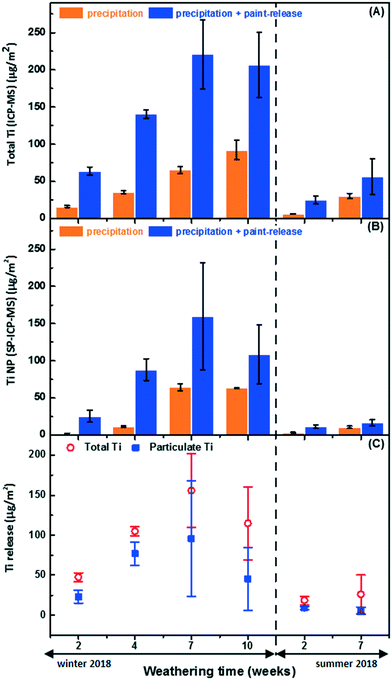 |
| Fig. 3 Quantification of Ti in the natural precipitation as well as the precipitation following its contact with painted panels: (A) total Ti measured by ICP-MS, (B) Ti NP measured by SP ICP-MS, and (C) the net release of total and nanoparticulate Ti during the winter and summer seasons. The quantities are expressed in terms of Ti metal content and are normalized by the exposure area of the painted panels. | |
In spite of this relatively large and increasing background signal, greater NP numbers (and masses) were observed in the containers containing painted surfaces (Fig. 3A and B). Raw SP-ICP-MS data comparing the precipitation (snow meltwater) (Fig. 4A) to snow meltwater that was in contact (2 weeks) with the painted panel (Fig. 4B) showed significantly more spikes, which was attributed to the release of particles from the paint. PSDs were strikingly similar (Fig. 4C and D), with most of the NPs uniformly observed for sizes below 60 nm.
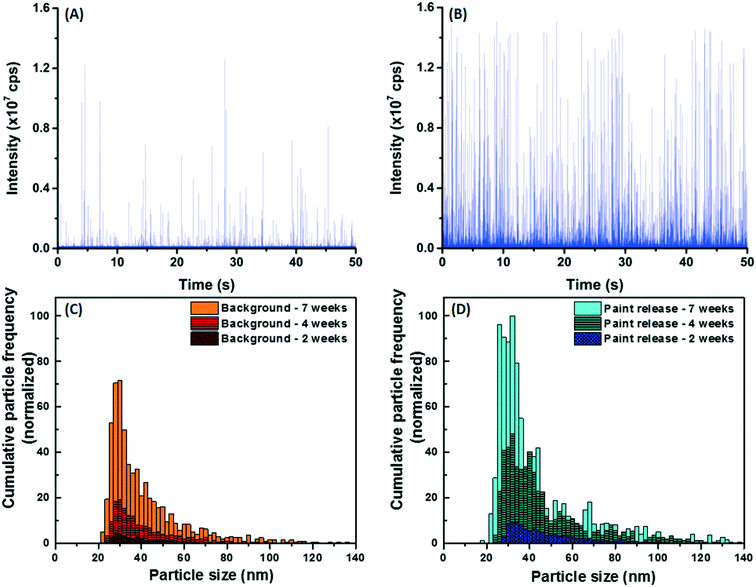 |
| Fig. 4 Time-resolved SP-ICP-MS signal (i.e. raw data) for Ti-containing NPs in (A) snow meltwater and (B) snow after 2 weeks of contact with a painted panel. Particle size distributions were obtained for (C) Ti-containing NPs in the natural precipitation background and (D) for NP released from the painted surfaces, over several weeks during the winter season. Note that paint-released NP frequencies are already corrected for background NPs occurring in the precipitation. Particle diameters were calculated on the assumption that particles were TiO2. | |
Significant release of Ti (ANOVA, p < 0.05) was observed (Fig. 3C), with a large proportion (55% on average) of it being attributed to the Ti NPs. In the winter, NP release appeared to peak at 168 ± 121 μg m−2, corresponding to (3.4 ± 2.5) × 1011 NP per m2 after 7 weeks and may have decreased after 10 weeks of exposure (Fig. 3C and Table 1), although the data are admittedly disparate due to the dynamic nature of this long-term exposure. Since sampling was conducted in a sacrificial manner in order to take into account the cumulative nature of the exposure medium, error bars increased with time, especially for the longer exposures. Certainly, NP concentrations will change as the NPs undergo physicochemical transformations (e.g. agglomeration, dissolution),42,43 as the precipitation undergoes modifications (e.g. evaporation, wind removal of snow, pH changes, new precipitation renewal), or as sorptive losses occur.11
Table 1 Quantification of the release of particulate TiO2 NPs (<450 nm) from painted panel surfaces, following their natural weathering for several weeks in Winter 2018 and Summer 2018. Quantities are reported in terms of TiO2 NP mass and number released per painted surface area or mass of dry paint load on the panel. The measurements were performed by high-resolution SP-ICP-MS
Season |
Weathering time |
Released TiO2 NPs from the painted surfaces (<450 nm fraction) after natural weathering |
NP mass per surface area |
NP mass per g-dry-paint |
NP number per surface area |
NP number per g-dry-paint |
Weeks |
μg m−2 |
ng per g-dry-paint |
NPs m−2 |
NPs per g-dry-paint |
Winter 2018 |
2
|
38.40 ± 13.62 |
118.67 ± 48.19 |
6.6 × 1010 ± 1.8 × 1010 |
2.0 × 108 ± 6.5 × 107 |
4
|
128.41 ± 24.56 |
313.83 ± 39.75 |
2.3 × 1011 ± 6.3 × 1010 |
5.4 × 108 ± 1.4 × 108 |
7
|
168.34 ± 120.55 |
369.81 ± 162.99 |
3.4 × 1011 ± 2.5 × 1011 |
8.1 × 108 ± 3.1 × 108 |
10
|
75.45 ± 65.84 |
166.37 ± 152.84 |
1.2 × 1011 ± 1.7 × 1011 |
2.3 × 108 ± 3.9 × 108 |
Summer 2018 |
2
|
14.70 ± 3.76 |
25.68 ± 7.4 |
3.2 × 1010 ± 5.6 × 109 |
5.3 × 107 ± 9.3 × 106 |
There appeared to be greater NP release in the winter as compared to the summer. While NP release during the first 2 weeks of summer weathering could be easily distinguished from background levels in the precipitation, this distinction became statistically insignificant (ANOVA, p > 0.05) after 7 weeks due to lower release-to-background ratios. Lower release in the summer did not appear to be correlated to the quantity of precipitation since rain events during the summer averaged 3.0 mm per day over the 7 weeks of exposure, whereas 2.5 mm per day (water equivalent) of snow and rain was recorded during the 10 weeks of cold weather. Whereas summer precipitation was mostly limited to a few abundant rainfall events, the winter precipitation was more uniformly distributed (Fig. S7†).
In summary, the data clearly show that weathering of the painted panels increased NP concentrations in the precipitation. The outdoor data also suggest that weathering was likely more important in the winter than in the summer and that the sizes of the released NP were generally smaller than those in the original paint. These two points are examined in more detail in the following sections.
How did weathering conditions affect TiO2 NP release?
For the experiments conducted in the summer, less release was observed when compared to measurements performed in the winter (i.e.Fig. 3C). The weather data (Fig. S7†) suggested that the summer samples underwent frequent wet-dry cycles with shorter liquid-surface interaction times, whereas the winter samples were nearly constantly exposed to snow or ice in addition to undergoing freeze–thaw cycles. Several controlled laboratory experiments were therefore designed to isolate the roles of seasonal variability, including the role of temperature, the role of moisture and the role of FT (freeze–thaw) cycles on the release of NPs from a painted surface. For a 42 day (6 week) exposure, cold conditions, wet conditions and FT conditions all contributed to increased NP release (Fig. 5). Among the different weathering conditions, exposure to water and FT cycles stood out as being important factors to boost NP release. For example, 27× more NPs were released from samples that underwent 21 FT cycles as compared to samples that were simply frozen (Fig. 5). On the other hand, wet RT samples released 12× more NPs than dry. Surprisingly, water did not appear to have an effect on the samples that were kept in the freezer in the absence of FT (Fig. 5). The data suggest that NP release is enhanced under wet conditions with high liquid-surface interaction times and increased further if the surface undergoes FT cycles. These results agree well with those for the natural weathering, consistent with the hypothesis that wet conditions along with FT cycles could indeed explain the increased NP release that was observed during the winter season.
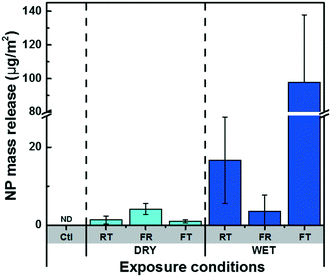 |
| Fig. 5 NP mass release under different exposure conditions, quantified by SP-ICP-MS and normalized by the exposure area of painted panels. Ctl refers to a control condition, where no panel was used. The exposure conditions consisted of dry and wet conditions, with RT, FR and FT denoting the sub-conditions of room temperature, only-freezing, and freeze–thaw, respectively. The exposure duration was 42 days or 21 cycles (in the case of FT). ND denotes “not detected” (ANOVA, p > 0.05). | |
In addition, time-resolved monitoring of the NP release was performed for the wet RT or FT (Fig. 6). During the first 18 days of weathering, no significant difference in the release quantities were observed; however, during the following 4 weeks of weathering (14 FT cycles), the mass of NPs released under FT conditions greatly surpassed those under RT conditions (Fig. 6A). Furthermore, while NP mass (and numbers) appeared to increase in the later stages of the FT experiments, they stabilized or decreased for the samples held at room temperature (Fig. 6A). As above, we hypothesize that NP loss is primarily driven by agglomeration43 leading to sedimentation44 of the NPs, as well as sorptive processes.11 This is consistent with the observation that the proportion of NP >100 nm consistently increased during the 4 weeks of weathering, prior to declining slightly around 6 weeks (Fig. 7).
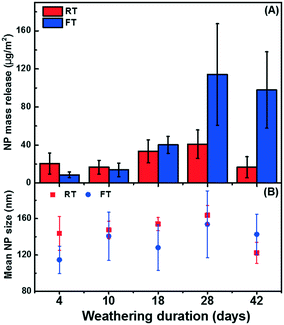 |
| Fig. 6 (A) NP mass release normalized by the exposure area of painted panels, for samples that were exposed to Milli-Q water (no NP background) under (wet) conditions of room temperature (RT) and freeze–thaw (FT) and (B) the corresponding mean NP sizes. The weathering duration of 4, 10, 18, 28 and 42 d correspond to 2, 5, 9, 14, and 21 FT cycles, respectively. | |
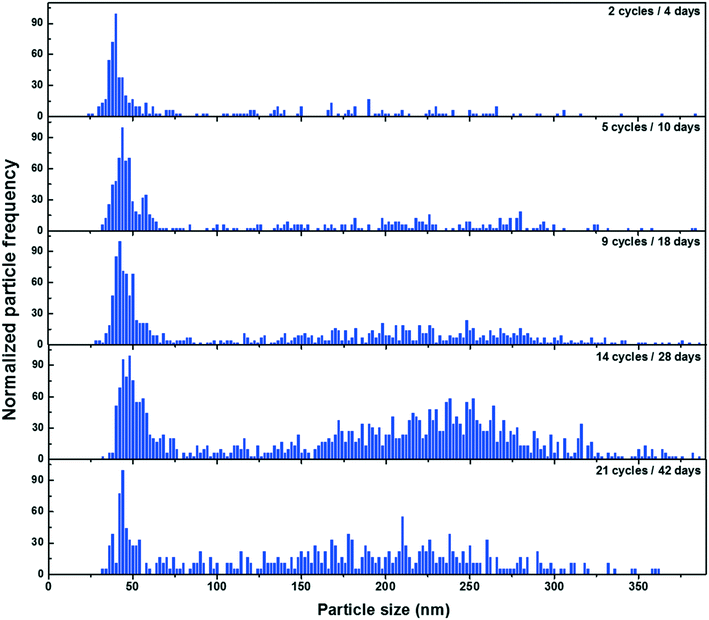 |
| Fig. 7 Particle size distributions for TiO2 NPs following their release from painted surfaces that were exposed to several weeks of FT cycles in Milli-Q water (no NP background). Each cycle consisted of 1 day of freezing and 1 day of thawing. Particle diameters were calculated on the assumption that particles were TiO2. | |
Are the sizes of weathered TiO2 NPs similar to those that were originally in the paint?
The particle size distributions of the weathered NPs (Fig. 4D) appeared to be smaller than sizes that were obtained in the original paint sample (Fig. S6A†). Two experiments were conducted to validate this observation: (i) first, a painted board was soaked in Milli-Q water in a closed container for 4 d at room temperature (20 °C), in order to more easily detect NP release by reducing the background signal; (ii) second, under the assumption that the NPs were originally agglomerated in the paint, the paint was diluted (2 × 107×), sonicated (30 min) and then stabilized through the addition of 5 mg L−1 of a fulvic acid.45
In Milli-Q water, a significant number of NPs were leached into solution and these NPs had a size distribution (Fig. S8†) that was very similar to what was observed under natural conditions, i.e. most of the NPs were smaller than 60 nm with a wide size distribution extending beyond 200 nm. Significantly more NPs were detected at the lower end of the size distribution ((8.4 ± 0.1) × 1015 NPs per kg-paint or 5.5 ± 0.1% by weight) as compared to the diluted (unsonicated) paint sample ((6.8 ± 0.1) × 1015 NPs per kg-paint or 5.0 ± 0.1% by weight). This observation suggests that the NPs in the paint were at least partially dispersed by the sonication and stabilization (Fig. S6B†), resulting in the detection of more primary particles in the smaller size ranges.
Environmental considerations and implications
The natural and lab weathering data present firm evidence that TiO2 NPs were leached out of painted surfaces, with the strongest release observed under wet and cold conditions – particularly, under conditions where freeze–thaw occurred. Although release escalated during the winter, the absolute quantity of release, even at its peak, was rather small, corresponding to <0.001% of total TiO2 NP load on the boards. While the weathering was fairly harsh, the study was conducted for 10 weeks only, and thus, given several years of life expectancy for painted façades (before renewal), increasingly more release could be expected, during long-term weathering and degradation of the surfaces. The results showed that environmental factors (e.g. temperature, moisture) will affect the quantity of NPs released in addition to influencing their fate through processes such as agglomeration, sedimentation and sorption.31,46–49 These processes will certainly have an impact on the mobility and bio-accessibility of these released NPs and will thus alter their environmental risk.
Conflicts of interest
The authors declare that they have no conflicts of interest.
Acknowledgements
This research was supported by the Natural Sciences and Engineering Research Council of Canada (NSERC), the Fonds du Recherche du Québec-Nature et Technologies (FRQNT), the Canadian Foundation for Innovation, Environment and Climate Change Canada, and McGill Engineering Doctoral Award (MEDA). We thank Prof. S. Ghoshal (McGill University) for helpful discussions.
References
- M. E. Vance, T. Kuiken, E. P. Vejerano, S. P. McGinnis, M. F. Hochella Jr, D. Rejeski and M. S. Hull, Nanotechnology in the real world: Redeveloping the nanomaterial consumer products inventory, Beilstein J. Nanotechnol., 2015, 6(1), 1769–1780 CrossRef CAS PubMed.
- F. Gottschalk and B. Nowack, The release of engineered nanomaterials to the environment, J. Environ. Monit., 2011, 13(5), 1145–1155 RSC.
- B. Nowack, J. F. Ranville, S. Diamond, J. A. Gallego-Urrea, C. Metcalfe, J. Rose, N. Horne, A. A. Koelmans and S. J. Klaine, Potential scenarios for nanomaterial release and subsequent alteration in the environment, Environ. Toxicol. Chem., 2012, 31(1), 50–59 CrossRef CAS PubMed.
- B. Giese, F. Klaessig, B. Park, R. Kaegi, M. Steinfeldt, H. Wigger, A. von Gleich and F. Gottschalk, Risks, release and concentrations of engineered nanomaterial in the environment, Sci. Rep., 2018, 8(1), 1565 CrossRef PubMed.
- T. Y. Sun, F. Gottschalk, K. Hungerbühler and B. Nowack, Comprehensive probabilistic modelling of environmental emissions of engineered nanomaterials, Environ. Pollut., 2014, 185, 69–76 CrossRef CAS PubMed.
- F. Loosli, J. Wang, S. Rothenberg, M. Bizimis, C. Winkler, O. Borovinskaya, L. Flamigni and M. Baalousha, Sewage spills are a major source of titanium dioxide engineered (nano)-particle release into the environment, Environ. Sci.: Nano, 2019, 6(3), 763–777 RSC.
- A. Weir, P. Westerhoff, L. Fabricius, K. Hristovski and N. Von Goetz, Titanium dioxide nanoparticles in food and personal care products, Environ. Sci. Technol., 2012, 46(4), 2242–2250 CrossRef CAS PubMed.
- I. Hincapié, A. Caballero-Guzman, D. Hiltbrunner and B. Nowack, Use of engineered nanomaterials in the construction industry with specific emphasis on paints and their flows in construction and demolition waste in Switzerland, Waste Manage., 2015, 43, 398–406 CrossRef PubMed.
- R. Hischier, B. Nowack, F. Gottschalk, I. Hincapie, M. Steinfeldt and C. Som, Life cycle assessment of façade coating systems containing manufactured nanomaterials, J. Nanopart. Res., 2015, 17(2), 68 CrossRef.
- P. Van Broekhuizen, F. van Broekhuizen, R. Cornelissen and L. Reijnders, Use of nanomaterials in the European construction industry and some occupational health aspects thereof, J. Nanopart. Res., 2011, 13(2), 447–462 CrossRef.
- A. Azimzada, N. Tufenkji and K. J. Wilkinson, Transformations of silver nanoparticles in wastewater effluents: links to Ag bioavailability, Environ. Sci.: Nano, 2017, 4(6), 1339–1349 RSC.
- C. Coll, D. Notter, F. Gottschalk, T. Sun, C. Som and B. Nowack, Probabilistic environmental risk assessment of five nanomaterials (nano-TiO2, nano-Ag, nano-ZnO, CNT, and fullerenes), Nanotoxicology, 2016, 10(4), 436–444 CrossRef CAS PubMed.
- R. Kaegi, B. Sinnet, S. Zuleeg, H. Hagendorfer, E. Mueller, R. Vonbank, M. Boller and M. Burkhardt, Release of silver nanoparticles from outdoor facades, Environ. Pollut., 2010, 158(9), 2900–2905 CrossRef CAS PubMed.
- A. Al-Kattan, A. Wichser, S. Zuin, Y. Arroyo, L. Golanski, A. Ulrich and B. Nowack, Behavior of TiO2 released from nano-TiO2-containing paint and comparison to pristine nano-TiO2, Environ. Sci. Technol., 2014, 48(12), 6710–6718 CrossRef CAS PubMed.
- J. Olabarrieta, S. Zorita, I. Peña, N. Rioja, O. Monzón, P. Benguria and L. Scifo, Aging of photocatalytic
coatings under a water flow: long run performance and TiO2 nanoparticles release, Appl. Catal., B, 2012, 123, 182–192 CrossRef.
- A. Al-Kattan, A. Wichser, R. Vonbank, S. Brunner, A. Ulrich, S. Zuin and B. Nowack, Release of TiO2 from paints containing pigment-TiO2 or nano-TiO2 by weathering, Environ. Sci.: Processes Impacts, 2013, 15(12), 2186–2193 RSC.
- X. Zhang, M. Wang, S. Guo, Z. Zhang and H. Li, Effects of weathering and rainfall conditions on the release of SiO2, Ag, and TiO2 engineered nanoparticles from paints, J. Nanopart. Res., 2017, 19(10), 338 CrossRef.
- S. Zuin, M. Gaiani, A. Ferrari and L. Golanski, Leaching of nanoparticles from experimental water-borne paints under laboratory test conditions, J. Nanopart. Res., 2014, 16(1), 2185 CrossRef.
- R. Kägi, A. Ulrich, B. Sinnet, R. Vonbank, A. Wichser, S. Zuleeg, H. Simmler, S. Brunner, H. Vonmont and M. Burkhardt, Synthetic TiO2 nanoparticle emission from exterior facades into the aquatic environment, Environ. Pollut., 2008, 156(2), 233–239 CrossRef PubMed.
- R. S. Lankone, K. E. Challis, Y. Bi, D. Hanigan, R. B. Reed, T. Zaikova, J. E. Hutchison, P. Westerhoff, J. Ranville and H. Fairbrother, Methodology for quantifying engineered nanomaterial release from diverse product matrices under outdoor weathering conditions and implications for life cycle assessment, Environ. Sci.: Nano, 2017, 4(9), 1784–1797 RSC.
- T. Künniger, A. C. Gerecke, A. Ulrich, A. Huch, R. Vonbank, M. Heeb, A. Wichser, R. Haag, P. Kunz and M. Faller, Release and environmental impact of silver nanoparticles and conventional organic biocides from coated wooden façades, Environ. Pollut., 2014, 184, 464–471 CrossRef PubMed.
- M. F. Hochella, D. W. Mogk, J. Ranville, I. C. Allen, G. W. Luther, L. C. Marr, B. P. McGrail, M. Murayama, N. P. Qafoku and K. M. Rosso, Natural, incidental, and engineered nanomaterials and their impacts on the Earth system, Science, 2019, 363(6434), eaau8299 CrossRef PubMed.
- M. F. Rahim, D. Pal and P. A. Ariya, Physicochemical studies of aerosols at Montreal Trudeau Airport: The importance of airborne nanoparticles containing metal contaminants, Environ. Pollut., 2019, 246, 734–744 CrossRef CAS PubMed.
- A. Gondikas, F. von der Kammer, R. Kaegi, O. Borovinskaya, E. Neubauer, J. Navratilova, A. Praetorius, G. Cornelis and T. Hofmann, Where is the nano? Analytical approaches for the detection and quantification of TiO2 engineered nanoparticles in surface waters, Environ. Sci.: Nano, 2018, 5(2), 313–326 RSC.
- M. Tharaud, A. P. Gondikas, M. F. Benedetti, F. von der Kammer, T. Hofmann and G. Cornelis, TiO2 nanomaterial detection in calcium rich matrices by spICPMS. A matter of resolution and treatment, J. Anal. At. Spectrom., 2017, 32(7), 1400–1411 RSC.
- L. Fréchette-Viens, M. Hadioui and K. J. Wilkinson, Practical limitations of single particle ICP-MS in the determination of nanoparticle size distributions and dissolution: case of rare earth oxides, Talanta, 2017, 163, 121–126 CrossRef PubMed.
- L. Fréchette-Viens, M. Hadioui and K. J. Wilkinson, Quantification of ZnO nanoparticles and other Zn containing colloids in natural waters using a high sensitivity single particle ICP-MS, Talanta, 2019, 200, 156–162 CrossRef PubMed.
- M. Hadioui, C. Peyrot and K. J. Wilkinson, Improvements to Single Particle ICPMS by the Online Coupling of Ion Exchange Resins, Anal. Chem., 2014, 86(10), 4668–4674 CrossRef CAS PubMed.
- B. Meermann and V. Nischwitz, ICP-MS for the analysis at the nanoscale–a
tutorial review, J. Anal. At. Spectrom., 2018, 33(9), 1432–1468 RSC.
- S. Lee, X. Bi, R. B. Reed, J. F. Ranville, P. Herckes and P. Westerhoff, Nanoparticle size detection limits by single particle ICP-MS for 40 elements, Environ. Sci. Technol., 2014, 48(17), 10291–10300 CrossRef CAS PubMed.
- A. P. Gondikas, F. von der Kammer, R. B. Reed, S. Wagner, J. F. Ranville and T. Hofmann, Release of TiO2 nanoparticles from sunscreens into surface waters: a one-year survey at the old Danube recreational Lake, Environ. Sci. Technol., 2014, 48(10), 5415–5422 CrossRef CAS PubMed.
- R. J. Peters, G. van Bemmel, N. B. Milani, G. C. den Hertog, A. K. Undas, M. van der Lee and H. Bouwmeester, Detection of nanoparticles in Dutch surface waters, Sci. Total Environ., 2018, 621, 210–218 CrossRef CAS PubMed.
- M. Auffan, J. Rose, J.-Y. Bottero, G. V. Lowry, J.-P. Jolivet and M. R. Wiesner, Towards a definition of inorganic nanoparticles from an environmental, health and safety perspective, Nat. Nanotechnol., 2009, 4(10), 634 CrossRef CAS PubMed.
- P. Shaw and A. Donard, Nano-particle analysis using dwell times between 10 μs and 70 μs with an upper counting limit of greater than 3× 107 cps and a gold nanoparticle detection limit of less than 10 nm diameter, J. Anal. At. Spectrom., 2016, 31(6), 1234–1242 RSC.
- M. Hadioui, G. Knapp, A. Azimzada, I. Jreije, L. Frechette-Viens and K. J. Wilkinson, Lowering the size detection limits of Ag and TiO2 nanoparticles by Single Particle ICP-MS, Anal. Chem., 2019, 91(20), 13275–13284 CrossRef CAS PubMed.
- I. De la Calle, M. Menta and F. Seby, Current trends and challenges in sample preparation for metallic nanoparticles analysis in daily products and environmental samples: A review, Spectrochim. Acta, Part B, 2016, 125, 66–96 CrossRef CAS.
- T. W. May and R. H. Wiedmeyer, A table of polyatomic interferences in ICP-MS, At. Spectrosc., 1998, 19, 150–155 CAS.
- M. F. Hochella, S. K. Lower, P. A. Maurice, R. L. Penn, N. Sahai, D. L. Sparks and B. S. Twining, Nanominerals, mineral nanoparticles, and earth systems, Science, 2008, 319(5870), 1631–1635 CrossRef CAS PubMed.
- S. Wagner, A. Gondikas, E. Neubauer, T. Hofmann and F. von der Kammer, Spot the difference: engineered and natural nanoparticles in the environment—release, behavior, and fate, Angew. Chem., Int. Ed., 2014, 53(46), 12398–12419 CAS.
- K. L. Plathe, F. von der Kammer, M. Hassellöv, J. N. Moore, M. Murayama, T. Hofmann and M. F. Hochella Jr, The role of nanominerals and mineral nanoparticles in the transport of toxic trace metals: Field-flow fractionation and analytical TEM analyses after nanoparticle isolation and density separation, Geochim. Cosmochim. Acta, 2013, 102, 213–225 CrossRef CAS.
- R. Hischier, B. Nowack, F. Gottschalk, I. Hincapie, M. Steinfeldt and C. Som, Life cycle assessment of façade coating systems containing manufactured nanomaterials, J. Nanopart. Res., 2015, 17(2), 68 CrossRef.
- M. Hadioui, V. Merdzan and K. J. Wilkinson, Detection and characterization of ZnO nanoparticles in surface and waste waters using single particle ICPMS, Environ. Sci. Technol., 2015, 49, 6141–6148 CrossRef CAS PubMed.
- S. Ottofuelling, F. von der Kammer and T. Hofmann, Commercial titanium dioxide nanoparticles in both natural and synthetic water: comprehensive multidimensional testing and prediction of aggregation behavior, Environ. Sci. Technol., 2011, 45(23), 10045–10052 CrossRef CAS PubMed.
- C. Botta, J. Labille, M. Auffan, D. Borschneck, H. Miche, M. Cabié, A. Masion, J. Rose and J.-Y. Bottero, TiO2-based nanoparticles released in water from commercialized sunscreens in a life-cycle perspective: Structures and quantities, Environ. Pollut., 2011, 159(6), 1543–1550 CrossRef CAS PubMed.
- R. F. Domingos, N. Tufenkji and K. J. Wilkinson, Aggregation of titanium dioxide nanoparticles: role of a fulvic acid, Environ. Sci. Technol., 2009, 43(5), 1282–1286 CrossRef CAS PubMed.
- D. Zhou, Z. Ji, X. Jiang, D. R. Dunphy, J. Brinker and A. A. Keller, Influence of material properties on TiO2 nanoparticle agglomeration, PLoS One, 2013, 8(11), e81239 CrossRef PubMed.
- R. Reed, D. Martin, A. Bednar, M. Montaño, P. Westerhoff and J. Ranville, Multi-day diurnal measurements of Ti-containing nanoparticle and organic sunscreen chemical release during recreational use of a natural surface water, Environ. Sci.: Nano, 2017, 4(1), 69–77 RSC.
- J. M. Farner, R. S. Cheong, E. Mahé, H. Anand and N. Tufenkji, Comparing TiO2 nanoparticle formulations: stability and photoreactivity are key factors in acute toxicity to Daphnia magna, Environ. Sci.: Nano, 2019, 6, 2532–2543 RSC.
- P. Christian, F. von der Kammer, M. Baalousha and T. Hofmann, Nanoparticles: structure, properties, preparation and behaviour in environmental media, Ecotoxicology, 2008, 17(5), 326–343 CrossRef CAS PubMed.
Footnote |
† Electronic supplementary information (ESI) available. See DOI: 10.1039/c9en00951e |
|
This journal is © The Royal Society of Chemistry 2020 |
Click here to see how this site uses Cookies. View our privacy policy here.