Graphite nanoparticle addition to fertilizers reduces nitrate leaching in growth of lettuce (Lactuca sativa)†
Received
4th August 2019
, Accepted 20th October 2019
First published on 21st October 2019
Abstract
Nitrogen leaching into groundwater occurs in nearly all intensively-fertilized agriculture applications and poses growing environmental and human health risks such as eutrophication and drinking water contamination. This potential for contamination will intensify as the population grows. This study focused on nitrate leaching through soil during the growth of romaine lettuce (Lactuca sativa), a high value crop in a region (Salinas Valley, CA) suffering from nitrate-contaminated water. 2-D graphite carbon nanoparticles (CNPs) produced via an electrochemical exfoliation process, resulting in ∼8 nm thickness and 250–850 nm width, were combined with fertilizer and applied to the lettuce in soil to test the CNP effect on yield, nitrate leaching, and plant nutrient uptake. Greenhouse experiments were conducted under different nutrient loadings and soil matrices. CNP addition did not inhibit the lettuce leaf yield, and decreased nitrate leaching in several scenarios. When fertilizer was reduced to 70% of the recommended dose and combined with less than 1% wt CNPs, nitrate leaching decreased by 57%. Furthermore, there was no significant difference in yield compared to the 100% recommended fertilizer dose without CNPs. Increasing the soil's hydraulic conductivity enhanced the ability of CNPs to reduce nitrate leaching and increase plant nitrogen uptake. CNP addition to mineral fertilizer blends may allow lower fertilizer doses and thus decrease nitrate infiltration through the soil without comprising yields.
Environmental significance
Agricultural runoff is a leading cause of nitrate contaminated water which can lead to eutrophication and human health impacts. Graphetic 2-D carbon nanoparticles combined with fertilizer were found to reduce the amount of nitrate being leached through the soil and increased the average lettuce yield over the non-nanoparticle dose in some of the treatments. Lettuce is a high value crop grown in areas such as the Salinas Valley, California, which have problems with nitrate contaminated groundwater and over fertilization. Additions of nanoparticles to fertilizer blends can allow for a reduction in nutrient leaching in turn preventing nutrient runoff and increasing nutrient availability to the plant.
|
1. Introduction
Population growth is increasing the demand for food, thereby increasing agricultural activities and putting greater stress on the environment. Currently, fertilizers are often over-applied to maximize yield, which deteriorates sensitive environments.1,2 Nitrogen loading to surface water causes eutrophication and toxic algae blooms throughout the USA, and it is also a factor in the hypoxic region in the Gulf of Mexico at the Mississippi River delta.3,4 For example, Salinas Valley (California, USA) cultivates numerous high-value crops, and the associated nitrate contamination from fertilizers is a leading cause of coastal eutrophication in Monterey Bay.5 Nitrate is regulated in drinking water to prevent methemoglobinemia, but recent studies associated lower nitrate levels (0.5 to 5 mg NO3-N per L) with a variety of cancers, and potentially in endocrine disruption.6,7 Nationally, adding nitrogen-based fertilizers for agriculture is also a primary factor for nitrate being the most frequently occurring groundwater pollutant. 20% of rural drinking water wells have nitrate above the Environmental Protection Agency's maximum contaminant level (MCL),8 and over 250
000 people in Salinas Valley and Tulare Lake Basin are at risk of nitrate contamination to their drinking water source, where croplands have contributed 90% of the nitrate groundwater loading.5
Harter and Lund (2012) estimate that nitrogen fertilizer additions would need to decrease by 443 kg N per ha per crop for field crops in the Salinas Valley in order to reduce groundwater nitrate leaching to benchmark levels (35 kg N per ha per crop).5 Several practices exist to mitigate nitrogen runoff such as constructed wetlands, bioretention facilities, cover crops, and conservation tillage.9,10 However, the current best management practices can require combining multiple techniques and can drastically range in their effectiveness of reducing nutrient leaching.
Nanotechnology is an alternative to nitrogen fertilizers that can potentially improve yields while also reducing the nutrient leaching.11,12 Nanotechnology has shown to improve crop growth, nutrient uptake, and seed germination.13–15 Nanomaterials are different from bulk material in both their physical and chemical properties and have large surface to volume ratios.16,17 The nanoparticle's effect on a plant will differ depending on the plant's growth stage, method of application, exposure time and concentration, and the physical and chemical composition of the nanoparticle.18 Carbon nanoparticles applied to plants can benefit or adversely influence soil microbes and crop yields.19–26 Many carbon nanoparticles have been studied for crop application, including carbon nanotubes, fullerene, fullerol, graphene, carbon nanohorns, and carbon nano-onions.19–21,25–27 Carbon nanotubes had a positive effect on tobacco, maize, and alfalfa when using a growth medium.24,26,28 However, negative effects on Arabidopsis growth and roots were observed with water soluble fullerene C70, revealing a disruption in cell division, mitochondrial activity, and microtubule organization.29
The effects of nanoparticles on crops are variable and require research to study specific nanoparticle and crop interactions. This paper focuses on carbon-based nanomaterials because there is limited work on the simultaneous effects of carbon nanoparticles on plant yield (i.e., increased food production) and nutrient leaching (i.e., environment impacts). Studies suggest carbon nanoparticle additions to an artificial growth medium, such as Murashige and Skoog medium, improve seed germination, but few studies use real soils or allow plants to grow for the full harvest period. Lettuce, a high value crop for the southwestern United States, was chosen due to its economic importance and nitrogen fertilizer requirements. The study focused on the effects of graphitic carbon nanoparticles (CNPs) on nitrate leaching and lettuce yield using local Arizona soil as the growth medium. The specific objectives were: (1) determine the effect of CNPs on lettuce yield, (2) use a nutrient balance to identify whether nanoparticles impact nutrient uptake and leaching, and (3) assess the effect of soil hydraulic conductivity on CNP and nutrient mobility. Three trials were conducted; trials 1 and 2 varied nutrient loads, and trial 3 varied the soil hydraulic conductivity. Lettuce leaf yield, nutrient leaching, and nutrient uptake into the plant tissue were collected for each trial.
2. Materials and methods
2.1. Planting strategy and harvesting
Lettuce seeds (Lactuca sativa, var. Green Towers; High Mowing Organic Seeds) were planted in 7.5 L plastic pots on June 13, 2017 (trial 1), September 8, 2017 (trial 2), and January 19, 2018 (trial 3) (Table S1†). Fig. 1 shows a photograph of the pots and diagram of the leachate collection system equipped to each pot. Pots were lined with fiberglass mesh (Saint-Gobain ADFORS) followed by 0.6 kg of gravel to filter soil from the leachate. Each pot received 3.3 kg of dry soil that was sieved using a U.S. Standard Sieve Series No. 10 (2000-micron mesh) for a homogenous soil composition. Soil was saturated prior to planting, and fertilizer was applied as a liquid to the soil surface (section 2.5). To address germination issues, four seeds were placed per pot in trial 1 and ten seeds were placed per pot in trial 2. In trial 2 due to ongoing germination issues, pots that did not germinate received one transplant that were grown in perlite three weeks after seeding. Seeds for trial 3 were grown in perlite (Vigoro Organic Perlite) and then transplanted one plant per pot on February 9, 2018. In trial 1 and trial 2 experiments, seeds were thinned to one seedling per pot approximately 10 days after planting, when the true leaves had developed. All trials had only one plant per pot that developed fully until harvest.
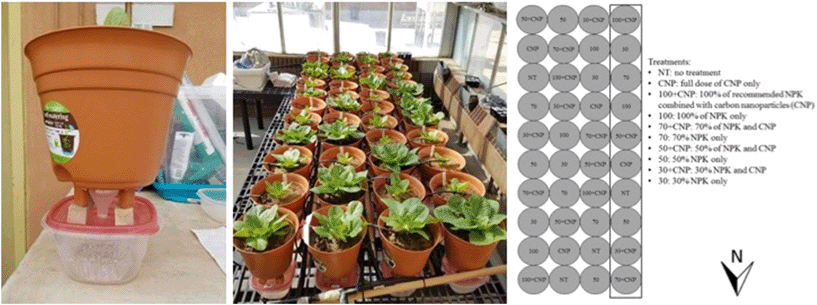 |
| Fig. 1 Photographs of the leachate collection system equipped on each pot and the pots arranged in the greenhouse (from left to right). On the right side, the layout of each treatment within a randomized block design is shown for trial 2. | |
Plants were grown in a temperature-controlled greenhouse (24 ± 2 °C) receiving natural light located at Arizona State University (33° 25′ 12.3′′ N and 111° 55′ 58.4′′ W). Temperature (Table S2†) and photosynthetic active radiation (PAR) (Fig. S1–S5†) were monitored continuously within the greenhouse, with the temperature controlled to remain in the optimum range for lettuce growth.30 To sufficiently saturate the soil near the root zone and to generate leachate, each lettuce plant was drip irrigated twice daily with tap water for 1–2 minutes using a poly tubing drip irrigation system and one Rain Bird emitter (3.79 L h−1) per pot. The lettuce was harvested after 7–8 weeks, and the wet and dry weight of the leaf and fully extracted root mass were recorded. The roots were rinsed thoroughly to remove attached soil.
2.2. Carbon nanoparticle preparation and characterization
The source of graphite carbon nanoparticles (CNPs) were based upon prior work by collaborators in China who observed improved crop production when CNPs were blended with fertilizers.37 The CNPs (Hualong Fertilizer Technology Company, China) were developed for agricultural use and produced via an electrochemical exfoliation process by applying an electric pulse of 3–5 V through an inert cathode and a pure graphite anode in an electrolyte solution.31 Nanoparticles as-received were characterized for size and shape using transmission electron microscopy (Philips CM200-FEG TEM). Energy dispersive spectroscopy (EDS) elemental mapping was used for surface composition (JEOL 2010 FEG TEM), and a carbon hydrogen nitrogen (CHN) analyzer (Perkin Elmer PE2400) was used for elemental composition. Pore size, pore volume, and surface area were determined by measuring and plotting the gas adsorbed versus the relative equilibrium (Micrometrics Tristar II 3020).32 The Brunauer–Emmett–Teller (BET) equation was used to determine surface area, and Barrett–Joyner–Halenda (BJH) methodology was used for pore size and volume. Surface thickness was analyzed by atomic force microscopy (Bruker MultiMode 8 AFM). The topographical images were taken in peak force tapping mode with non-contact cantilevers (NCHV) with a spring constant of 42 N m−1 (Bruker, Camarillo, CA). Image analysis used the Nanoscope Analysis version 1.7 software (Fig. S10†).
2.3. Soil types
Local Arizona top soil (15–30 cm from surface) collected from the same plot at the Maricopa Agricultural Center (33°04′ 22′′ N, 111° 58′ 26.5′′ W) was sieved and then used in all the experiments. In trial 3, two additional soil blends were created by blending the Arizona soil with sand to make a 30% sand (30S) and 70% sand (70S) by dry weight in order to increase the saturated hydraulic conductivity. The Arizona soil was characterized as a Casa Grande clay loam composed of 34.7% sand, 32.8% silt, and 32.5% clay using the United States Geological Survey (USGS) Web Soil survey. All three soils had pH between 8.4 and 8.9, and the Arizona soil had an organic matter content of 0.54%. General pre-planting soil sample compositions can be found in Table S3.† The Arizona soil, 30S, and 70S blends had characteristics similar to some of the soil properties found in the Salinas Valley (USGS Web Soil Survey). The saturated hydraulic conductivity for each soil type was determined using a UMS KSAT saturated hydraulic conductivity meter and the method from its Operation Manual.33 The saturated hydraulic conductivity (ksat) for Arizona soil, 30S, and 70S was 4.62 × 10−6 m s−1, 5.23 × 10−6 m s−1, and 3.35 × 10−5 m s−1, respectively (Table S3†). A 15 cm long tubular soil sampler was used to take ten to twelve soil cores from each pot post harvesting.
2.4. Fertilizer treatments
Fertilizer treatments were configured in a randomized complete block design (Fig. S6–S8†). Trials had a different number of treatments, dictated by the purpose of each trial: varying one fertilizer dose (trial 1), varying three fertilizer doses (trial 2), and varying soil hydraulic conductivity (trial 3). Supplied nutrients (ratio of N–P–K) were ammonium nitrate (34–0–0) from ESKS and triple superphosphate (0–45–0) and muriate potash (0–0–60) both from Fertizona. Micronutrients were in the form of zinc sulfate, supplied from Fertizona, and were applied separately to prevent precipitation with the NPK fertilizer.
Fertilizer application rates (196 kg N per ha (175 lbs per acre), 67 kg P2O5 kg ha−1 (60 lbs per acre), 135 kg K2O per ha (120 lbs per acre), and 3.4 kg ZnS per ha (3 lbs per acre)) were based on the Lettuce Production in California guidebook and applied proportionally to each pot (Table 1).34 Based on successful experiments in China,37 CNPs were dosed at 3000 mg CNP per kg fertilizer to treatments with a “+CNP” in the name. Fertilizer blends were applied as a liquid by dissolving the granular fertilizer into tap water. The CNPs were combined with the fertilizer nutrient blends using sonication and stir plates (details in the ESI† under Fertilizer treatments).
Table 1 Nutrient application rates (kg ha−1) for nitrogen, phosphorus, potassium, and CNPs for trial 1, trial 2, and trial 3 growing periods. To convert application rates from field relevant units (kg/ha) to actual mass added per pot (mg/pot), multiple values below by 4.1 ([mg per pot]/[kg ha−1]). Number of replicates per treatment that grew successfully were out of 6 plants for trial 1 and 4 plants for trails 2 and 3
Trial # |
Fertilizer treatment |
Experiment acronym |
Number of replicates of reps |
Application rates (kg ha−1) |
N |
P2O5 |
K2O |
CNP |
All fertilizer treatments in trial 3 were planted in 3 different soil types.
The CNP30S and 30–30S only had three replicates due to one plant dying mid-growing season.
|
1 |
No fertilizer |
NT |
6 |
0 |
0 |
0 |
0 |
1 |
CNP dosing only |
CNP |
6 |
0 |
0 |
0 |
2.85 |
1 |
100% recommended NPK dosing |
100 |
4 |
196 |
67 |
135 |
0 |
1 |
CNP plus 100% recommended NPK |
100 + CNP |
3 |
196 |
67 |
135 |
2.85 |
1 |
CNP plus 70% recommended NPK |
70 + CNP |
6 |
137 |
47 |
94 |
2.00 |
2 |
No fertilizer |
NT |
3 |
0 |
0 |
0 |
0 |
2 |
CNP dosing only |
CNP |
4 |
0 |
0 |
0 |
2.85 |
2 |
100% recommended NPK dosing |
100 |
2 |
196 |
67 |
135 |
0 |
2 |
CNP plus 100% recommended NPK |
100 + CNP |
3 |
196 |
67 |
135 |
2.85 |
2 |
70% recommended NPK dosing |
70 |
3 |
137 |
47 |
94 |
0 |
2 |
CNP plus 70% recommended NPK |
70 + CNP |
2 |
137 |
47 |
94 |
2.00 |
2 |
50% recommended NPK dosing |
50 |
3 |
98 |
34 |
68 |
0 |
2 |
CNP plus 50% recommended NPK |
50 + CNP |
3 |
98 |
34 |
68 |
1.44 |
2 |
30% recommended NPK dosing |
30 |
4 |
59 |
20 |
41 |
0 |
2 |
CNP plus 30% recommended NPK |
30 + CNP |
1 |
59 |
20 |
41 |
0.85 |
3 |
No fertilizer (AZ, 30S, 70S soil)a |
NT |
4 |
0 |
0 |
0 |
0 |
3 |
CNP dosing only (AZ, 30S, 70S soil)a |
CNP |
4b |
0 |
0 |
0 |
0.85 |
3 |
30% recommended NPK dosing (AZ, 30S, 70S soil)a |
30 |
4b |
59 |
20 |
41 |
0 |
3 |
CNP plus 30% recommended NPK (AZ, 30S, 70S soil)a |
30 + CNP |
4 |
59 |
20 |
41 |
0.85 |
2.4.1. Trial 1 & 2 experimental setup.
Trial 1 had five nutrient treatments replicated six times (Table 1) as a proof of concept experiment to evaluate if CNPs had an effect on plant growth and nitrogen leaching through the soil (Fig. S6†). Trial 2 had ten fertilizer treatments replicated four times to test the effect of varying the fertilizer dose (Fig. S7†). The fertilizer treatments were as follows: no fertilizer control (NT), carbon nanoparticles only (CNP), 100% mineral fertilizer (100), 100% mineral fertilizer with CNP (100 + CNP), 70% mineral fertilizer (70), 70% mineral fertilizer with CNP (70 + CNP), 50% mineral fertilizer (50), 50% mineral fertilizer with CNP (50 + CNP), 30% mineral fertilizer (30), and 30% mineral fertilizer with CNP (30 + CNP). The 70 + CNP, 50 + CNP, and 30 + CNP treatments received 30%, 50%, and 70% less nutrients and nanoparticles than 100 + CNP, respectively. The CNP-only dose received the same amount of carbon nanoparticles as 100 + CNP. Due to germination issues in trial 2, pots that did not germinate received one transplanted lettuce plant that were grown in perlite three weeks after initial seeding. Plants that were transplanted were staggered by three weeks (harvest 2) from the initial seedlings (harvest 1). Harvest 1 plants were all grown and harvested in the same greenhouse; however, harvest 2 plants were moved to another greenhouse due to greenhouse maintenance for the final three weeks of their growing cycle. No effect on yield from moving greenhouses was observed between harvest 1 and harvest 2 plants.
2.4.2. Trial 3 experimental setup.
Trial 3 tested the effect of hydraulic conductivity (increasing drainage by adding sand) on CNP performance using four fertilizer treatments (NT, CNP, 30, and 30 + CNP) and three soil blends. The three soil blends were 100% Arizona soil (AZ), Arizona soil blended with 30% sand by dry weight (30S), and Arizona soil blended with 70% sand by dry weight (70S). The sand (DecoRock Paver Sand) was dried and sieved through a 2000 micron mesh before blending. Thirty percent of the recommended nutrient requirements were used for all the mineral fertilizer doses. All fertilizer treatments were added at a 30% fertilization rate to examine if the effects on yield could be attributed to changes in hydraulic conductivity and CNP addition and not over fertilization. Trial 3 fertilizer treatments were: no treatment in Arizona soil (NT AZ), no treatment in 30% sand blend (NT 30S), no treatment in 70% sand blend (NT 70S), carbon nanoparticles only in Arizona soil (CNP AZ), carbon nanoparticles only in 30% sand blend (CNP 30S), carbon nanoparticles only in 70% sand blend (CNP 70S), 30% mineral fertilizer in Arizona soil (30 AZ), 30% mineral fertilizer with CNP in Arizona soil (30 + CNP AZ), 30% mineral fertilizer in 30% sand blend (30–30S), 30% mineral fertilizer with CNP in 30% sand blend (30 + CNP 30S), 30% mineral fertilizer in 70% sand blend (30–70S), and 30% mineral fertilizer with CNP in 70% sand blend (30 + CNP 70S).
2.4.3. Nitrogen mass balances.
The nitrogen in leachate, plant tissue, and soil were used to formulate a nutrient balance for each trial. Eqn (1) was used to calculate the percentage and mass of applied nitrogen from fertilizer that ended up in the leachate and plant tissue. |  | (1) |
where N is the amount of nitrogen in the leachate or plant tissue, No is the amount of nitrogen in the NT (i.e., no fertilizer treatment) leachate or NT plant tissue, and TF is the total amount of nitrogen applied from fertilizer. Details are provided in the ESI;† Tables S13–S20 contain the data used for the calculations.
2.5. Leachate collection and measurement
Lysimeters were used to collect leachate. The lysimeters used a funnel at the bottom of each pot that flowed into a leachate collection reservoir (Fig. S9†). Leachate was collected bi-weekly or as needed. Volume, conductivity, and pH were measured upon collection using a graduated cylinder, Oakton ECTestr 11+ meter, and Oakton pHTestr 30 meter, respectively. Both probes were calibrated prior to leachate collection. Leachate was then filtered using a 0.2 μm Nylon membrane filter (Environmental Express) and analyzed for anions and cations (Table S4†).
2.6. Analytical methods
The wet and dry weights of the lettuce leaf and extracted root mass were recorded for each pot. Lettuce leaf yield is the recorded wet weight of the leaves. Plant tissue was dried at 60 °C for one week, ground (Thomas Scientific 3383-L10 Wiley Mill), and sent to Waters Agricultural Labs, Inc. for nutrient analysis (Table S4†).35 The soil composition was digested using the Mehlich 3 acid extraction method by Waters Agricultural Labs, Inc. and analyzed for nutrients, metals, soil pH, and cation exchange capacity (CEC) (Table S4†). Metals and phosphorus in the plant tissue and soil were measured on a iCAP TQ ICP-MS (Thermo-Fisher Scientific) using a Mehlich 3 acid soil extraction method and a plant tissue wet digestion Digi Block 3000 method. Cations (ammonium, calcium, sodium, potassium, magnesium) and anions (nitrate, phosphate, chloride, sulfate) in the leachate aqueous solutions were measured at Arizona State University on an ion chromatograph (Dionex ICS-5000 DC) equipped with an IONPAC column AS18 for anion and CS12-A for cation (Table S4†).
2.7. Data analysis
Statistical analysis was conducted on the lettuce leaf yield for trial 1 and trial 2 combined and trial 3 experiments. Data was analyzed using IMB SPSS 24.0 software. A one-way ANOVA was used with treatment as the independent variable and yield as the dependent variable. A confidence interval of p < 0.10 was chosen due to variability within plant growth, which increased data uncertainty. The leaf yield for trial 1/trial 2 combined data and trial 3 data were log-transformed to a normal distribution. A Welch correction was used on the combined trial 1/trial 2 data due to a violation in the assumption of homogeneity of variances with analysis using a Games–Howell post hoc test (p < 0.10) for yields considered significantly different. Trial 3 data passed the Shapiro–Wilks and Levene's test, and a one-way ANOVA was then conducted with a Tukey's HSD post hoc test (p < 0.10).
3. Results
3.1. CNP characterization
SEM and TEM were used to characterize the CNP size and structure (Fig. S10–S12†). SEM images exhibited a range of aggregated and single particle sizes (Fig. S11†). The TEM images showed a combination of a crystalline structure made of parallel graphitic sheets and amorphous carbon defects (Fig. S10a and S12a–c†). The CNP sizes ranged from 250 to 850 nm wide and <5 to ∼40 nm in thickness (Fig. S10a and d and S11†). The average particle thickness was 8 nm determined from 100 particle cross sections using AMF in conjunction with the section function in the Nanoscale software (Fig. S10c and d†). The Raman spectrum (Fig. S10b†) showed a E2g peak at 1573 cm−1 (G-band), which indicates a graphitic composition, and a disorder-induced peak at 1354 cm−1 (D-band), which is attributed to defects in the structure.36,37 The defects are in the form of amorphous carbon and do not have crystalline structures seen in Fig. S12a.† The CHN results showed that the CNPs are approximately 96% carbon, primarily composed of graphite and amorphous carbon structures seen in the TEM images. Copper, calcium, potassium, sodium, oxygen, and other elements compose the remaining 4% (Fig. S12d†). The average BET surface area was 1.82 m2 g−1, and the Barrett–Joyner–Halenda (BJH) pore volume was 0.0072 cm3 g−1. The CNPs had negative zeta potentials between pH 4 to 10 (Table S5†).
3.2. Effect of NPK loading and hydraulic conductivity on leaf yield
3.2.1. Effect of nutrient loading on yield.
One hundred percent nutrient addition without CNP (labeled as “100”) and 70 + CNP treatments (i.e., 70% of the recommended nutrients plus CNP) produced the two highest average leaf yields (Fig. 2a and Tables S6 and S7†). Compared against 100 + CNP, the 70 + CNP treatment was the optimum treatment to lower fertilizer inputs with minimal reduction to leaf yield. Alone amongst the treatments, the 70 + CNP treatment enhanced the average leaf yield by 17% compared with the non-CNP treatment at the same fertilizer dose (Fig. 2a and Table S8†). The average leaf yield declined significantly with the 50 + CNP and 30% treatments compared to the 100%, 70%, and non-CNP 50% doses (Fig. 2a and Table S8†). In the combined trial 1 and 2 statistical analysis, there was no statistical difference in yield between plants fertilized with 100% and 70% treatments (p > 0.10), implying less fertilizer can be applied and still achieve adequate growth. There was no statistical difference (p > 0.10) in leaf yield between treatments with and without CNPs except for the 50% treatment (Table S8†). All plants treated with NPK had significantly higher leaf yields (p < 0.10) than the controls (no fertilizer added), showing that the soil alone is not supplying sufficient nutrients for optimal growth, and an additional nutrient source is necessary.
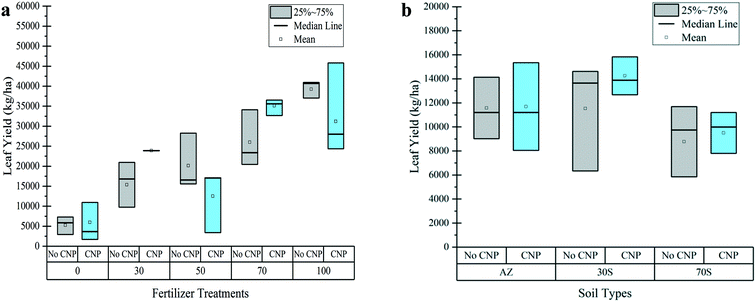 |
| Fig. 2 Aggregate leaf yield data across all growing seasons when varying a) fertilizer treatments as 0, 30%, 50%, 70% or 100% of the recommended NPK dose and, b) soil hydraulic conductivity with 0, 30% (30S) or 70% (70S) sand mixed into the soil. Bar and whisker diagrams show mean, median and quartile ranges (box). Blue boxes are for treatments that include nutrients and CNP; grey boxes are for nutrient addition without CNP. | |
3.2.2. Effect of hydraulic conductivity on yield.
A 30% fertilizer treatment for trial 3 was used to understand if changes in yield are due to differences in hydraulic conductivity and CNP addition and not from over fertilization. The 30 + CNP 30S and 30 + CNP AZ treatments produced the highest average leaf yields followed by 30 AZ and 30–30S (Fig. 2b and Table S9†). The 30 + CNP 30S treatment increased the average leaf yield by 24% over the non-CNP treatment, making it the optimum blend for CNPs to enhance growth. Increasing the hydraulic conductivity of the soil allowed for increased average leaf yield in the plants treated with CNPs versus the non-CNP plants (Fig. 2b). All fertilized treatments were significantly higher in leaf yield than the controls.
3.3. Nitrogen leaching
This study investigated whether CNP addition with fertilizers can reduce the amount of nutrients leaching from the soil with nitrogen being the main concern. Of the nitrogen species analyzed, >95% of the leached nitrogen was in the form of nitrate, and thus nitrate was the focus in the results. Leachate data for all other nutrients can be found in Tables S10–S12.† The 30 + CNP data was not included because only one replicate grew and was not considered a representative sample (Table 1).
There was a trend of lower nitrate leaching in the 70 + CNP treatment compared to the 70% fertilizer dose without CNP. In experiments where the nutrient loading varied (Fig. 3a and b), the 70 + CNP treatment on average had 43% less nitrate leached than the 70 treatment. This translates to an average of 19.7 kg NO3− per ha leached for the 70 treatment and 10.8 kg NO3− per ha leached for the 70 + CNP treatment in trial 2 (Table S11†). The CNPs did not have an effect on the 100% treatments possibly due to an oversaturation of nitrogen or phosphorous (Fig. 3a and Tables S10 and S11†). The 50% and 70% fertilizer treatments with CNPs had a lower average nitrate leached compared to non-CNP treatments (Fig. 3a and b).
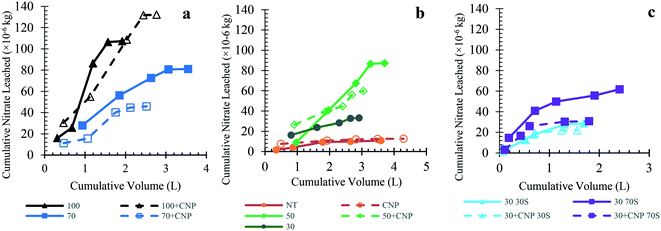 |
| Fig. 3 Cumulative nitrate leached (×10−6 kg) versus cumulative volume leached (L) for: a) 100, 100 + CNP, 70, 70 + CNP treatments (averages from trials 1 and 2), b) NT, CNP, 50, 50 + CNP, 30 treatments (averages from trials 1 and 2), and c) 30S and 70S soils in trial 3. | |
When the hydraulic conductivity varied (trial 3), the 30 + CNP 30S and 30 + CNP 70S treatments leached less nitrate compared to the non-CNP treatments, indicating the CNPs had a role in reducing nitrate leaching (Fig. 3c). The CNPs did not have an effect in the Arizona soil in trial 3 and leached more nitrate while having similar yields to the non-CNP treatment (Tables S12 and S20†). The amount of nitrate leached between the NT and CNP treatments was similar between all soil types. Furthermore, there was also less nitrogen leaching from the 70S soil type, because with 70% sand in the matrix the 70S initially contained less ambient or naturally-occurring nitrogen (Table S12†). In both the 30–30S and 30–70S treatments, the plants with CNP had a higher average yield and a lower amount of nitrate leached (Table S20†). There was a 22% reduction in nitrate leached between the 30–30S and 30 + CNP 30S treatments and a 55% reduction between the 30–70S and 30 + CNP 70S treatments, when comparing treatments with and without CNPs added to the fertilizer.
3.4. Nutrient balance
To understand nitrogen mobility and effect of CNPs, a nutrient balance was conducted. Nitrogen (N) was the only nutrient considered due to the concern of nitrate contamination in water bodies. In trial 1, over 80% of the applied nitrogen (from fertilizer) was recovered in the leachate, plant tissue (leaf and root), and soil (Table S13 part A and B†). Of the N supplied through fertilization, 14%, 17%, and 8% was recovered in the leachate, and 44%, 40%, and 44% was in the plant tissue (leaves and root) for treatments 100, 100 + CNP, and 70 + CNP, respectively (Fig. 4 and Table S13 part C). None of the treatments fell within the optimum nitrogen content range (33–48 g N per kg lettuce), according to Hartz and Johnstone (2007) for high lettuce leaf yield production (Table S14†).1 The 70 + CNP had approximately half of the average amount of applied nitrogen leached compared to the 100 + CNP and 100 fertilizer treatments (Fig. 4). All treatments had approximately the same amount of nitrogen uptake into the root tissue. For the 100% and 70% treatments, the amount of leached nitrogen decreased as plant yield increased (Fig. S13 and S14†).
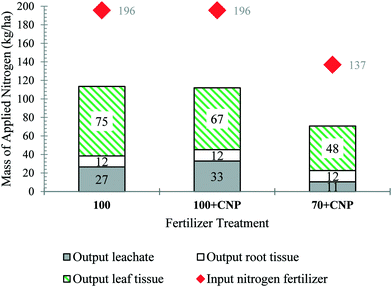 |
| Fig. 4 Trial 1 nutrient balance comparisons for the mass of applied nitrogen (kg ha−1) against the nitrogen outputs from the pot in the form of leachate (gray bar), leaf tissue (hashed-marked green bar), and root tissue (white bar). | |
The largest portion of applied nitrogen was found in the plant tissue for trial 2, which follows the trial 1 data trends (Fig. 4 and 5). The plant tissue nitrogen of CNP treatments was higher than the non-CNP treatments for the 70% treatment only (Fig. 5 and Table S15†). In Table S15,† the 100, 70, 70 + CNP, 50, and 30 experiments all had similar uptake efficiencies of applied nitrogen from fertilizer into the plant tissue averaging ∼25%. However, only treatments 100, 100 + CNP, 70 + CNP, and 50 fell within optimum nitrogen content concentrations for high yield lettuce production (Table S16†).1 The 100 + CNP showed an increase in nitrogen leached compared to the 100 treatment; in addition, the 100 + CNP nitrogen tissue content was lower than the 100 dose, indicating nitrogen was leached through the soil profile instead of being adsorbed by the roots. The 70 + CNP and 50 + CNP treatments reduced the average amount of applied nitrogen leached by half compared to the 70 and 50 treatments (Fig. 5). There was no consistent trend of CNP treatments having higher nitrogen uptake in the plant tissue, implying that for this trial CNPs played a larger role in nitrogen leaching rather than in uptake.
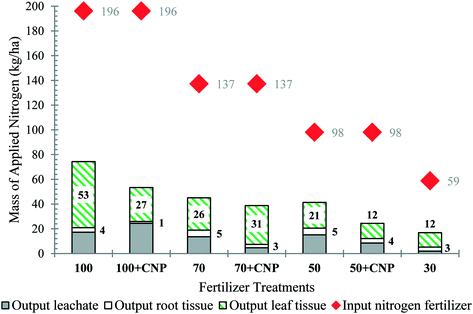 |
| Fig. 5 Trial 2 nutrient balance comparisons for the mass of applied nitrogen (kg ha−1) against the nitrogen outputs from the pot in the form of leachate (gray bar), leaf tissue (hashed-marked green bar), and root tissue (white bar). | |
In experiments with varying soil hydraulic conductivity (trial 3), less nitrogen leached and more nitrogen was in the plant tissue for the 30 + CNP 30S and 30 + CNP 70S treatments compared with the non-CNP treatments (Fig. 6 and Table S18†). In Fig. 4 through 6, less nitrogen was accounted for in the sum of leachate plus root and leaf tissue compared against the mass of nitrogen fertilizer added. Part of the unaccounted for nitrogen is due to biogeochemical processes that produce N-gases by soil microbes. Considering, the mass of nitrogen added as fertilizer, to the mass of soil, represented a small fraction of the nitrogen in the soil before or after the experiments, there were minimal differences in the soil nitrogen content across the duration of the growth experiment. Compared against experiments without CNP addition to the fertilizers, adding CNPs had on average 12–13% more nitrogen in the plant tissue in trial 3 (Table S19†). The 70S treatments had a lower nutrient content due to less naturally-available nutrients in the soil. The nitrogen leached was reduced by over half between the 30 + CNP and 30 treatments in the 30S and 70S soil compositions. In the soils with increased hydraulic conductivity, the CNPs increased yield and nitrogen in the plant tissue and decreased the amount of nitrogen being leached compared to non-CNP treatments (Fig. S15 and Table S20†).
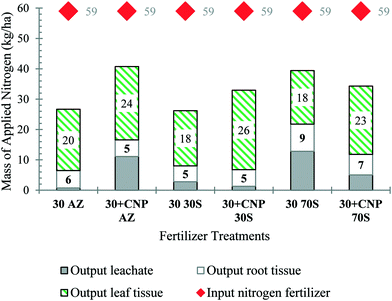 |
| Fig. 6 Trial 3 nutrient balance comparisons for the mass of applied nitrogen (kg ha−1) against the nitrogen outputs from the pot in the form of leachate (gray bar), leaf tissue (hashed-marked green bar), and root tissue (white bar). 30% recommended NPK fertilizer loading rates, both with (+CNP) or without graphitic nanoparticles, in three soil matrices: 1) Arizona soil (AZ), 2) 30% sand blended with 70% Arizona soil (30S), and 3) 70% sand blended with 30% Arizona soil (70S). | |
4. Discussion
4.1. Effect of nutrient loading and CNPs on yield
The 70 + CNP and 30 + CNP 30S treatments were the two optimal scenarios to increase lettuce yield compared with the non-CNP treatments (Fig. 2). There were no statistically lower leaf yields between CNP and non-CNP treated plants, except in the 50% treatment, implying that CNPs did not hinder growth (Tables S8 and S9†). The average leaf yields were highest in the 100% and 70% treatments and began to decline for the 50% and 30% treatments. This decline in average yield for lower nutrient doses implies that at least 70% of the recommended nutrients are needed for adequate growth. The 100 non-CNP and 70 + CNP treatments were the only treatments with average yields (39
227 and 35
085 kg ha−1, respectively) above the average romaine lettuce yield in the Salinas Valley (∼34
800 kg ha−1).38 The CNPs in this study have been used previously at the lab scale and in field trials and have shown a significant positive impact on growth through the following suggested mechanisms: increased nutrient adsorption and active transport of nutrients to the roots; enhanced synthesis of starch, which in turn increased the carbohydrate production and photosynthesis; and increased mitochondria within the plants.37 These mechanisms could be a contributing factor in the increase in average leaf yield found in some of the CNP fertilized plants in this study.
The Arizona soil structure and composition were one of the factors believed to hinder the effects of CNPs on yield due to germination issues caused by mechanical resistance where the seedling had difficulty emerging through the soil.39 The complexity of using a soil matrix as a growth medium could potentially also lower the CNP effectiveness. Other studies involving different types of carbon nanomaterials showed a positive effect on germination and yield when using an artificial growth medium or directly applying the CNPs to the seeds.40–42 A study on tomato plants found that nanoparticle size, shape, crystallinity, surface chemistry, and charge played an important role on physiological responses of tomato plants.41,43 The higher the negative surface charge and better dispersed the functionalized CNTs were, the larger the tomato growth.41 The CNPs used in our study did not stay well dispersed in water, and potentially favored instability and aggregation in the fertilizer solution, which may have impacted their effect on yield. The alkaline nature of Arizona soils may also impact attachment of negatively charged CNPs during infiltration. In addition, the CNPs in this study varied greatly in size and had crystalline and non-crystalline structure, which may have caused the variability in increasing yield in only some treatments.
4.2. Effect of soil properties on yield, nutrient mobility, and CNP effectiveness
Soil conductivity can affect CNP performance and nutrient availability. In addition, the high pH (pH 8.4) and calcareous nature of the Arizona soil are not conducive for plant growth because they can impede nutrient availability (Table S3†).44,45 Because CNPs did not consistently improve yield and lower nitrate leaching for all treatments in trials 1 and 2, it can be concluded that soil composition is an important factor in the effectiveness of CNPs. Soil properties, such as the high clay and silt contents in the Arizona soil, impact the behavior and mobility of nanoparticles and affect their homo- and hetero-aggregation due to their high surface area and small particle size.46–48 Nanoparticles can hetero-aggregate with natural soil colloids, which reduces their mobility within the soil matrix.46,47 The Arizona soil had a high clay and silt content that could bind the CNPs and therefore lower their effectiveness. This was evident in trial 3; the 30S and 70S soil blends, which had lower amounts of clay and silt particles and organic matter content, showed CNPs to have an improved effectiveness in leaf yield and nitrogen uptake and reduced nitrate leaching compared to the AZ soil with CNPs (Fig. 2b and Table S20†). This trend may indicate that the increase in hydraulic conductivity allowed for increased movement of the CNPs from the soil surface to the root zone and therefore enhanced plant growth and reduced leaching. However, the 70S soil blend also had less naturally-available nutrients, which may be why those treatments had a lower average leaf yield (8770–9500 kg ha−1) compared to the AZ (11500–11
700 kg ha−1) and 30S (11500–14
250 kg ha−1) soil types (Table S20†). The increase in hydraulic conductivity will also increase the leaching ability of nutrients; however, CNP adsorption properties can reduce nutrient leaching. This was observed in the 70S soil blend; the CNPs most likely increased adsorption of nitrogen species and therefore reduced the amount of nitrate being leached. This implies that increasing the saturated hydraulic conductivity through sand addition can allow for better mobility of the nanoparticles to adsorb ions and less hetero-aggregation with soil particles with the tradeoff of less naturally-available soil nutrients.
4.3. Effect of CNP properties on nutrient mobility
The nitrate leaching data between CNP and non-CNP treatments in trials 1 and 2 had varying results in which the 70 + CNP treatment was the optimal treatment for increasing yield while also lowering nitrate leached compared to the non-CNP treatment (Table S17 and Fig. S14†). The 70 + CNP results are important in that there was over a 56% reduction of the nitrate leached between the 70 + CNP and 100 treatments without compromising the leaf yield in both trial 1 and trial 2 (Fig. 2a). In addition, trial 3 showed that increasing the hydraulic conductivity (drainage) of the soil made CNPs more effective in reducing nitrate leaching, improving yield and nitrogen uptake into the plant (Fig. 2b and S15†). We hypothesize for future research that the improved CNP mobility can allow them to act as a potential slow-release nitrogen fertilizer that increases nutrient delivery to the plant's root zone and decreases nutrient leaching. In trial 3, the 30S soil blend showed the largest increase in leaf yield (24%) while the 70S soil blend had the largest reduction in nitrate leached (54% reduction) when comparing the CNP and non-CNP treatments (Table S20†).
The reduction in nitrate leached with CNP addition in this study could be due to the adsorption properties and surface characteristics of the CNPs, which can have a significant impact on the nutrient fate. The adsorptive properties of carbon materials for nutrients and contaminants are well established, hence the reason they are used in filters and in water and wastewater treatment processes.49 Many nutrient leaching studies using biochar and charcoal found that carbon additions reduced nutrient leaching.50–53 These conclusions were further established in a laboratory adsorption study that showed CNPs have an affinity for nitrogen species and removed ∼18% of ammonium and nitrate at the 1500 mg CNP per L dose (Fig. S16†). This translates to ∼0.035 mg N per mg CNP for the 300 mg CNP per L dose and ∼0.015 mg N per mg CNP for the 1500 mg CNP per L dose. This was also seen in a study of CNPs produced from biochar in which the presence of functional groups led to higher selectivity of ammonium over nitrate ions due to negative surface charge.54 Sonkar et al. (2012) investigated water-soluble carbon nano-onions and concluded they adsorb anions through hydrogen bonding and electrostatic interactions and then slowly release them.55 Charcoal addition to the soil reduced nitrogen leaching due to electrostatic adsorption of nutrients and retention of soil water containing nutrients.52 A study on biochar found it reduced the nitrate leached due to the adsorption of ammonium and other soluble compounds, which in turn prevented mineralization and nitrification of the ammonium.50 This could have been the case for CNPs that then acted as a slow-release fertilizer for nitrogen; therefore, decreasing the amount of nitrogen leaching into the water and instead adsorbed by the lettuce roots, however further investigation is needed.
This study showed mixed effects of CNPs on leaching and plant nutrient content due to germination issues, lower yields, variability between replicates, and properties of the Arizona soil (pH, calcareous, etc.). The yield, nutrient leaching, and plant tissue composition are interconnected and showed for some treatments that the smaller the yield, the less nutrient uptake and more nutrients leached (Fig. S13–15†). The problem of nitrate leaching can also be intensified when fertilizers are over-applied, which can be a contributing factor to the lowered effectiveness in the 100% mineral fertilizer treatments. Additionally, the amount of PAR sunlight reaching the plants was lower in trial 2, which can affect yields and nutrient uptake (Fig. S3 and S4†) because there is a direct correlation between nutrient uptake and solar radiation.56,57 Trial 2 data demonstrates this effect; the yields and plant nutrient content in the fertilized treatments were lower compared with trial 1 (Table S17†).
5. Conclusion
There is a need for new technologies, such as combining CNPs with mineral fertilizer, to help reduce the impacts that agricultural production has on the environment. Without fertilizer addition, lower plant yields were observed and clearly demonstrated the necessity to add nutrient-based fertilizers. We found that compared against fertilizer without CNP added, CNP fertilizer treatments did not have a negative impact on lettuce yield and decreased nitrate leaching in some fertilizer treatments. Two strategies were tested to reduce nitrate leaching: reducing the amount of fertilizer and changing the saturated hydraulic conductivity of the soil. To maintain a lettuce yield of over 34
000 kg ha−1 (i.e., similar to the average in the Salinas Valley38), the amount of mineral fertilizer could be reduced by 30% to result in 25% less nitrate leaching, or the amount of fertilizer could be reduced by 30% and combined with less than 1 wt% of CNPs to result in 57% less nitrate leaching compared with using 100% of the recommended fertilizer dose. Treatments using CNPs did not change the nutritional value of the leaf tissue, based upon elemental analysis (Tables S14–S19†). Additionally, increasing the soil hydraulic conductivity improved the effect of CNPs on reducing nitrate leaching and increasing plant nutrient uptake. Adding CNP to fertilizer blends could allow farmers to add less fertilizer while achieving high production yields and reducing the amount of nitrate reaching surrounding water bodies.
In addition to understanding the economic and life cycle implications of CNPs as fertilizer additives, future research should also consider the farm worker, environmental health, and safety precautions of working with CNPs, as could be leaching of CNPs into ground or surface waters or uptake into plants. To help explain the observations herein that CNPs have the potential to reduce nitrogen leaching from agricultural soils, research is needed to better understand how CNPs influence biogeochemical mechanisms (e.g., plant root microbiota, CNP-nitrogen adsorption) on nitrogen processes in the soil.
Conflicts of interest
There are no conflicts to declare.
Acknowledgements
This work was partially funded from the US Environmental Protection Agency through the STAR program (RD83558001) and the National Science Foundation (EEC-1449500) Nanosystems Engineering Research Center on Nanotechnology-Enabled Water Treatment. Technical assistance from Dr. Yuqiang Bi and technical editing by Laurel Passantino was greatly appreciated.
References
- T. K. Hartz, P. R. Johnstone, E. Williams and R. F. Smith, Establishing Lettuce Leaf Nutrient Optimum Ranges Through DRIS Analysis, Hortscience, 2007, 42(1), 143–146 CAS
.
- T. K. Hartz, W. E. Bendixen and L. Wierdsma, The Value of Presidedress Soil Nitrate Testing as a Nitrogen Management Tool in Irrigated Vegetable Production, Hortscience, 2000, 35(4), 651–656 CAS
.
-
N. R. Council, Nutrient control actions for improving water quality in the Mississippi River Basin and Northern Gulf of Mexico, National Academies Press, 2009 Search PubMed
.
-
N. R. Council, Improving water quality in the Mississippi river basin and northern Gulf of Mexico: Strategies and priorities, National Academies Press, 2012 Search PubMed
.
-
T. Harter and J. R. Lund, Addressing Nitrate in California's Drinking Water: With a Focus on Tulare Lake Basin and Salinas Valley Groundwater: Report for the State Water Resources Control Board Report to the Legislature, 2012 Search PubMed.
- M. J. Pennino, J. E. Compton and S. G. Leibowitz, Trends in Drinking Water Nitrate Violations Across the United States, Environ. Sci. Technol., 2017, 51(22), 13450–13460 CrossRef CAS
.
- M. H. Ward, R. R. Jones, J. D. Brender, T. M. de Kok, P. J. Weyer, B. T. Nolan, C. M. Villanueva and S. G. van Breda, Drinking Water Nitrate and Human Health: An Updated Review, Int. J. Environ. Res. Public Health, 2018, 15(7), 31 Search PubMed
.
- K. R. Burow, B. T. Nolan, M. G. Rupert and N. M. Dubrovsky, Nitrate in Groundwater of the United States, 1991–2003, Environ. Sci. Technol., 2010, 44(13), 4988–4997 CrossRef CAS
.
- J. Manuel, Nutrient Pollution: A Persistent Threat to Waterways, Environ. Health Perspect., 2014, 122(11), A304–A309 CrossRef
.
- J. Vymazal, Removal of nutrients in various types of constructed wetlands, Sci. Total Environ., 2007, 380(1), 48–65 CrossRef CAS
.
- S. M. Rodrigues, P. Demokritou, N. Dokoozlian, C. O. Hendren, B. Karn, M. S. Mauter, O. A. Sadik, M. Safarpour, J. M. Unrine, J. Viers, P. Welle, J. C. White, M. R. Wiesner and G. V. Lowry, Nanotechnology for sustainable food production: promising opportunities and scientific challenges, Environ. Sci.: Nano, 2017, 4(4), 767–781 RSC
.
- J. C. White and J. Gardea-Torresdey, Achieving food security through the very small, Nat. Nanotechnol., 2018, 13(8), 627–629 CrossRef CAS PubMed
.
- K. Pandey, M. H. Lahiani, V. K. Hicks, M. K. Hudson, M. J. Green and M. Khodakovskaya, Effects of carbon-based nanomaterials on seed germination, biomass accumulation and salt stress response of bioenergy crops, PLoS One, 2018, 13(8), e0202274 CrossRef
.
- S. C. Capaldi Arruda, A. L. Diniz Silva, R. Moretto Galazzi, R. Antunes Azevedo and M. A. Zezzi Arruda, Nanoparticles applied to plant science: A review, Talanta, 2015, 131, 693–705 CrossRef CAS
.
- A. Mukherjee, S. Majumdar, A. D. Servin, L. Pagano, O. P. Dhankher and J. C. White, Carbon Nanomaterials in Agriculture: A Critical Review, Front. Plant Sci., 2016, 7(172), 172 Search PubMed
.
- M. Rossi, F. Cubadda, L. Dini, M. Terranova, F. Aureli, A. Sorbo and D. Passeri, Scientific basis of nanotechnology, implications for the food sector and future trends, Trends Food Sci. Technol., 2014, 40(2), 127–148 CrossRef CAS
.
- E. Roduner, Size matters: why nanomaterials are different, Chem. Soc. Rev., 2006, 35(7), 583–592 RSC
.
- F. Aslani, S. Bagheri, N. Muhd Julkapli, A. S. Juraimi, F. S. G. Hashemi and A. Baghdadi, Effects of Engineered Nanomaterials on Plants Growth: An Overview, Sci. World J., 2014, 2014, 28 Search PubMed
.
- P. Begum, R. Ikhtiari and B. Fugetsu, Graphene phytotoxicity in the seedling stage of cabbage, tomato, red spinach, and lettuce, Carbon, 2011, 49(12), 3907–3919 CrossRef CAS
.
- J. E. Cañas, M. Long, S. Nations, R. Vadan, L. Dai, M. Luo, R. Ambikapathi, E. H. Lee and D. Olszyk, Effects of functionalized and nonfunctionalized single-walled carbon nanotubes on root elongation of select crop species, Environ. Toxicol. Chem., 2008, 27(9), 1922–1931 CrossRef
.
- C. Lin, B. Fugetsu, Y. Su and F. Watari, Studies on toxicity of multi-walled carbon nanotubes on Arabidopsis T87 suspension cells, J. Hazard. Mater., 2009, 170(2), 578–583 CrossRef CAS PubMed
.
- P. Jackson, N. R. Jacobsen, A. Baun, R. Birkedal, D. Kühnel, K. A. Jensen, U. Vogel and H. Wallin, Bioaccumulation and ecotoxicity of carbon nanotubes, Chem. Cent. J., 2013, 7(1), 154 CrossRef PubMed
.
- L. Jin, Y. Son, T. K. Yoon, Y. J. Kang, W. Kim and H. Chung, High concentrations of single-walled carbon nanotubes lower soil enzyme activity and microbial biomass, Ecotoxicol. Environ. Saf., 2013, 88, 9–15 CrossRef CAS
.
- M. V. Khodakovskaya, K. de Silva, A. S. Biris, E. Dervishi and H. Villagarcia, Carbon nanotubes induce growth enhancement of tobacco cells, ACS Nano, 2012, 6(3), 2128–2135 CrossRef CAS
.
-
D. Sawant, Effect of carbon nanotubes on seed germination, growth and yield of hybrid Bt cotton Var. 7383 BG II, 2016 Search PubMed.
- P. Miralles, E. Johnson, T. L. Church and A. T. Harris, Multiwalled carbon nanotubes in alfalfa and wheat: toxicology and uptake, J. R. Soc., Interface, 2012, 9(77), 3514–3527 CrossRef CAS
.
- R. De La Torre-Roche, J. Hawthorne, Y. Deng, B. Xing, W. Cai, L. A. Newman, C. Wang, X. Ma and J. C. White, Fullerene-enhanced accumulation of p, p'-DDE in agricultural crop species, Environ. Sci. Technol., 2012, 46(17), 9315–9323 CrossRef CAS
.
- D. K. Tiwari, N. Dasgupta-Schubert, L. M. Villaseñor Cendejas, J. Villegas, L. Carreto Montoya and S. E. Borjas García, Interfacing carbon nanotubes (CNT) with plants: enhancement of growth, water and ionic nutrient uptake in maize (Zea mays) and implications for nanoagriculture, Appl. Nanosci., 2014, 4(5), 577–591 CrossRef CAS
.
- Q. Liu, Y. Zhao, Y. Wan, J. Zheng, X. Zhang, C. Wang, X. Fang and J. Lin, Study of the Inhibitory Effect of Water-Soluble Fullerenes on Plant Growth at the Cellular Level, ACS Nano, 2010, 4(10), 5743–5748 CrossRef CAS
.
- I. Seginer, G. Shina, L. D. Albright and L. S. Marsh, Optimal temperature setpoints for greenhouse lettuce, J. Agric. Eng. Res., 1991, 49, 209–226 CrossRef
.
-
J. Liu, Preparation of nano graphite powder from nano-graphite sol, 2003 Search PubMed.
- A. Solanki and T. H. Boyer, Pharmaceutical removal in synthetic human urine using biochar, Environ. Sci.: Water Res. Technol., 2017, 3(3), 553–565 RSC
.
-
UMS Operation Manual KSAT, Germany, 2013 Search PubMed.
-
R. Smith, M. Cahn, O. Daugovish and S. Koike, Leaf lettuce production in California, UCANR Publications, 2011 Search PubMed.
- Waters Agricultural Laboratories, I, https://watersag.com/
.
- S. Reich and C. Thomsen, Raman spectroscopy of graphite, Philos. Trans. R. Soc., A, 2004, 362(1824), 2271–2288 CrossRef CAS
.
-
G. Song, M. Pandorf, P. Westerhoff and Y. Ma, Carbon Nanomaterial-Based Fertilizers Can Improve Plant Growthii, in Nanotechnology Applications in the Food Industry, ed. V. R. Rai and J. A. Bai, Taylor & Francis, 2018, pp. 22–44 Search PubMed
.
-
D. Geisseler and W. R. Horwath, Lettuce production in California, Fertilizer Research and Education Program, http://apps.cdfa.ca.gov/frep/docs/Lettuce_Production_CA.pdf, Accessed May, 2014 Search PubMed
.
-
J. Letey, Relationship between Soil Physical Properties and Crop Production, in Advances in Soil Science, ed. B. A. Stewart, Springer New York, New York, NY, 1985, pp. 277–294 Search PubMed
.
- C. Kole, P. Kole, K. M. Randunu, P. Choudhary, R. Podila, P. C. Ke, A. M. Rao and R. K. Marcus, Nanobiotechnology can boost crop production and quality: first evidence from increased plant biomass, fruit yield and phytomedicine content in bitter melon (Momordica charantia), BMC Biotechnol., 2013, 13(1), 1 CrossRef
.
- M. V. Khodakovskaya, B. S. Kim, J. N. Kim, M. Alimohammadi, E. Dervishi, T. Mustafa and C. E. Cernigla, Carbon nanotubes as plant growth regulators: effects on tomato growth, reproductive system, and soil microbial community, Small, 2013, 9(1), 115–123 CrossRef CAS PubMed
.
- M. H. Lahiani, J. Chen, F. Irin, A. A. Puretzky, M. J. Green and M. V. Khodakovskaya, Interaction of carbon nanohorns with plants: uptake and biological effects, Carbon, 2015, 81, 607–619 CrossRef CAS
.
- H. Villagarcia, E. Dervishi, K. d. Silva, A. S. Biris and M. V. Khodakovskaya, Surface Chemistry of Carbon Nanotubes Impacts the Growth and Expression of Water Channel Protein in Tomato Plants, Small, 2012, 8(15), 2328–2334 CrossRef CAS
.
-
B. Hopkins and J. Ellsworth in Phosphorus availability with alkaline/calcareous soil, Western Nutrient Management Conference, 2005, 2005, pp. 88–93 Search PubMed.
-
G. Thomas, Soil pH and soil acidity, Methods of Soil Analysis Part 3—Chemical Methods, 1996, (methodsofsoilan3), pp. 475–490 Search PubMed
.
- C. Ma, J. C. White, J. Zhao, Q. Zhao and B. Xing, Uptake of Engineered Nanoparticles by Food Crops: Characterization, Mechanisms, and Implications, Annu. Rev. Food Sci. Technol., 2018, 9(1), 129–153 CrossRef CAS
.
- S. M. Rodrigues, T. Trindade, A. C. Duarte, E. Pereira, G. F. Koopmans and P. F. A. M. Römkens, A framework to measure the availability of engineered nanoparticles in soils: Trends in soil tests and analytical tools, TrAC, Trends Anal. Chem., 2016, 75, 129–140 CrossRef CAS
.
- G. Cornelis, K. Hund-Rinke, T. Kuhlbusch, N. Van den Brink and C. Nickel, Fate and Bioavailability of Engineered Nanoparticles in Soils: A Review, Crit. Rev. Environ. Sci. Technol., 2014, 44(24), 2720–2764 CrossRef CAS
.
-
J. Przepiórski, Chapter 9 Activated carbon filters and their industrial applications, in Interface Science and Technology, ed. T. J. Bandosz, Elsevier, 2006, vol. 7, pp. 421–474 Search PubMed
.
- D. Laird, P. Fleming, B. Wang, R. Horton and D. Karlen, Biochar impact on nutrient leaching from a Midwestern agricultural soil, Geoderma, 2010, 158(3), 436–442 CrossRef CAS
.
- Y. Yao, B. Gao, M. Zhang, M. Inyang and A. R. Zimmerman, Effect of biochar amendment on sorption and leaching of nitrate, ammonium, and phosphate in a sandy soil, Chemosphere, 2012, 89(11), 1467–1471 CrossRef CAS
.
- J. Lehmann, J. Pereira da Silva, C. Steiner, T. Nehls, W. Zech and B. Glaser, Nutrient availability and leaching in an archaeological Anthrosol and a Ferralsol of the Central Amazon basin: fertilizer, manure and charcoal amendments, Plant Soil, 2003, 249(2), 343–357 CrossRef CAS
.
- J. Major, M. Rondon, D. Molina, S. J. Riha and J. Lehmann, Maize yield and nutrition during 4 years after biochar application to a Colombian savanna oxisol, Plant Soil, 2010, 333(1), 117–128 CrossRef CAS
.
- M. Saxena, S. Maity and S. Sarkar, Carbon nanoparticles in ‘biochar’boost wheat (Triticum aestivum) plant growth, RSC Adv., 2014, 4(75), 39948–39954 RSC
.
- S. K. Sonkar, M. Roy, D. G. Babar and S. Sarkar, Water soluble carbon nano-onions from wood wool as growth promoters for gram plants, Nanoscale, 2012, 4(24), 7670–7675 RSC
.
- D. C. E. Wurr and J. R. Fellows, The influence of solar radiation and temperature on the head weight of crisp lettuce, J. Hortic. Sci., 1991, 66(2), 183–190 CrossRef
.
-
C. Campillo, R. Fortes and M. del Henar Prieto, Solar radiation effect on crop production, in Solar Radiation, InTech, 2012 Search PubMed
.
Footnote |
† Electronic supplementary information (ESI) available. See DOI: 10.1039/c9en00890j |
|
This journal is © The Royal Society of Chemistry 2020 |