Band engineered p-type RGO–CdS–PANI ternary nanocomposites for thermoelectric applications†
Received
14th June 2017
, Accepted 6th August 2017
First published on 7th August 2017
Abstract
A ternary hybrid nanocomposite of RGO–CdS–polyaniline (PANI) is prepared by a simple two stage in situ method for its thermoelectric studies. For this purpose, CdS quantum dots were first prepared with varying concentrations in the presence of reduced graphene oxide to form RGO–CdS nanocomposites using 3-mercaptopropionic acid as a linker. Polyaniline was then prepared in situ in the presence of RGO–CdS nanocomposites to eventually obtain RGO–CdS–PANI nanocomposites. The electrical conductivity, Seebeck coefficient and power factor of the RGO–CdS–PANI nanocomposites were calculated with various loadings of RGO–CdS nanocomposites in PANI. The final RGO–CdS–PANI nanocomposites delivered a high electric conductivity in the order of 105 S m−1 and showed strong re-dispersion in DMF and ethanol. The effective band alignment and decreased thermal conductivity in RGO–CdS–PANI nanocomposites resulted in p-type behaviour and a high power factor value.
Introduction
Nanomaterials possess unique chemical, physical, and mechanical properties and thus find applications in a wide variety of devices like photovoltaics, transistors, LEDs, sensors, etc.1,2 Similarly, nanomaterials showing thermoelectric (TE) properties have attracted growing attention due to direct conversion between thermal energy and electrical energy.3,4 Thermoelectric devices have been recognized for reducing global energy consumption, a long time solution and highly promising transformative technology. These energy utilizations are eco-friendly and non-polluting, and thus, such devices attract great attention of the global research and industrial communities.5–8 However, the relatively high cost and poor processability of inorganic semiconductor TE materials are impeding their applications in advanced thermoelectric systems.9 The efficiency of thermoelectric materials and devices is characterized by the dimensionless figure of merit (ZT), which contains three physical quantities i.e. the Seebeck coefficient (S), electrical conductivity (σ) and thermal conductivity (k). The figure of merit can be expressed by the equation ZT = (S2σ/κ)T, where T is the absolute temperature.10,11 These parameters are correlated with each other and are relatively difficult to optimize at once. Nowadays, in the field of materials technology, combining various materials through synergy is one of the most successful approaches to achieve specific goals with great efficiency in properties and cost effectiveness.12–15 In this respect, graphene and its composites are one of the classes of materials which have great applications in various fields including thermoelectrics. Due to the incredible properties of graphene, along with its relatively low cost, facile processing and recyclability, it can be utilized in various advanced applications.16 Graphene has zero band gap and high electrical conductivity, and mixing it with semiconducting nanomaterials results in inter-material charge transfer. Thus, when graphene combines with nanomaterials like, PbSe, PbTe, CoSb3, etc. the electrical conductivity of the resultant composite increases significantly.17
Nanocomposites of conducting polymers with an inorganic material like graphene are also a topic of interest among researchers because of their resultant low thermal conductivity and high electrical conductivity.18,19 The addition of a conducting polymer imparts easy processing of the composite and reduces thermal conductivity while graphene improves the electrical conductivity.20,21 Conducting polymers such as polyaniline (PANI), polythiophene, PEDOT–PSS, polyacetylene, and polypyrrole, and their derivatives have great potential for use in thermoelectric energy applications.22–25 Conducting polymers possess unique features like high mechanical flexibility, light weight, low-cost synthesis, solution processability, and printability which can be highly advantageous for thermoelectric applications.26–30 However, it is observed that the use of conducting polymers along with graphene/CNTs failed to deliver high thermoelectric power factors. Also, the processing of these composites is difficult due to their low re-dispersion in solvents.
Thus, in the present work, we have typically combined RGO with CdS QDs and PANI to enhance the thermoelectric properties and improve the re-dispersion. For this purpose, we first performed the in situ synthesis of RGO–CdS nanocomposites with three different ratios of RGO to CdS. These RGO–CdS nanocomposites were thoroughly characterized and then combined with polyaniline by polymerizing aniline in situ in their presence to form ternary RGO–CdS–PANI nanocomposites. The as-formed RGO–CdS–PANI nanocomposites with varied concentrations of RGO–CdS nanocomposites were then used for thermoelectric studies by forming thick pellets. The measurement of the Seebeck coefficient, thermal conductivity, and electrical conductivity was performed experimentally and the power factor (PF) was calculated from these values. The effect of variation in CdS concentration on thermoelectric properties of RGO–CdS–PANI nanocomposites was studied in detail.
Experimental
Chemicals and methods
All chemicals and solvents were purchased commercially. Cadmium chloride (CdCl2), sodium sulphide (Na2S) and other solvents were obtained from Sigma Aldrich India Ltd. All chemicals and solvents were of reagent or analytical grade and were used as received. Graphitic flakes (Reinste Nano ventures) and aniline (Merck, India) were also purchased commercially. UV-visible spectra were recorded in toluene at room temperature using a SPECORD 210 PLUS UV spectrophotometer (Analytik Jena, Germany). Photoluminescence (PL) measurements of solutions were performed using a Cary Eclipse Fluorescence Spectrophotometer G9800A (Agilent Technologies, USA). The samples were excited at 350 nm and emissions were monitored between 370 and 800 nm. Powder X-ray diffraction patterns were recorded using Cu-Kα (λ = 1.5406 Å) radiation on a Mini Flex Rigaku X-ray diffractometer.
SEM images and elemental analysis were performed on a Carl Zeiss Scanning electron microscope. Infrared (FTIR) spectra were recorded at room temperature from 4000 cm−1 to 400 cm−1 with an FTIR Perkin Elmer spectrum two (USA). TEM images were obtained using an FEI-TECNAI G2 (Czech Republic).
Synthesis of RGO–CdS nanocomposites
Graphene was prepared in three steps as reported in the literature.31 In the first step, graphite oxide was prepared from graphitic flakes by the Hummers method. In the second step, thermally expanded graphene oxide (TEGO) was prepared by thermal expansion/exfoliation at 1050 °C (Ar, 30 s). Finally, RGO was obtained by the hydrogen reduction of TEGO at 400 °C for 2 h. In the second stage, we adopted the in situ synthesis method to prepare RGO–CdS nanocomposites where RGO nanoplatelets were first re-dispersed in DMF. CdS QDs were then grown in this solution by using 2-mercaptopropionic acid (MPA) as a capping agent and sodium sulphide as a sulphur source. We varied the ratio of RGO to CdS (w/w%) as 1
:
0.1 (G2), 1
:
0.25 (G3) and 1
:
0.5 (G4) by varying the concentration of cadmium and sulphur sources during the synthesis.
Synthesis of RGO–CdS–PANI nanocomposites for thermoelectric applications
In the last stage, the as-prepared RGO–CdS nanocomposites with varied concentrations (G1, G2 and G3) were dispersed in HCl solution separately and polyaniline was grown in situ by polymerisation of aniline using ammonium sulphate as an oxidizing agent. The final RGO–CdS–PANI nanocomposite samples with various loadings and ratios were subjected to cold pressing to form thick pellets for further testing of thermoelectric properties. The overall synthesis process for the formation of RGO–CdS–PANI nanocomposites is mentioned in Scheme 1. The loading of the as-prepared RGO–CdS nanocomposite in PANI was varied from 0.1 to 0.4 wt% to observe its influence on thermoelectric properties. The as-prepared RGO–CdS nanocomposite was thoroughly characterized to know the degree of CdS formation on RGO nanoplatelets.
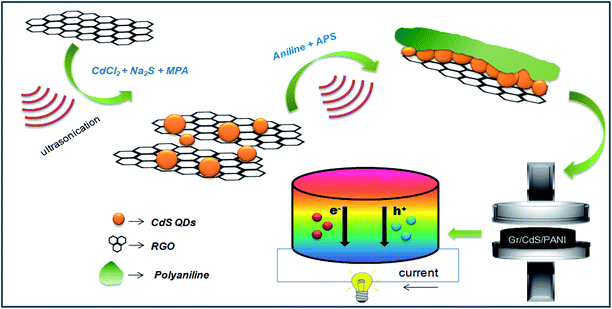 |
| Scheme 1 Schematic representation of RGO–CdS–PANI preparation nanocomposites for thermoelectric applications. | |
Results and discussion
The presence of RGO and CdS QDs in the RGO–CdS nanocomposite was confirmed by UV-visible and PL spectroscopy (Fig. 1a and b). For this purpose, we separately synthesized CdS QDs alone by a similar method using MPA as the capping agent. The UV-visible spectrum of CdS alone (see Fig. SI. 1†) showed an absorption peak at 370 nm confirming the formation of CdS QDs, as the obtained band gap (∼3.35 eV) was much higher than the bulk band gap of CdS (∼2.2 eV). The observed absorption profiles of G2, G3 and G4 samples were typically similar to the absorption profile of CdS QDs. Since, RGO is not expected to show any absorbance in the visible region the only absorption possible for these samples was due to the presence of CdS QDs in the nanocomposites. Thus, the presence of CdS QDs was confirmed in the as-prepared G2, G3 and G4 nanocomposites. However, in the case of the RGO–CdS–PANI nanocomposite with a 0.4 wt% loading of the G4 sample, two broad peaks covering the entire long-UV and visible region of the spectrum were observed. These broad peaks were signature peaks of emeraldine base polyaniline confirming the formation of polyaniline in the RGO–CdS–PANI nanocomposite. In addition to UV-visible spectroscopy, we also performed photoluminescence (PL) spectroscopy for the as-prepared CdS QDs, G2, G3 and G4 and RGO–CdS–PANI nanocomposites. The emission profile of CdS QDs showed a typical broad peak centred at 525 nm which is due to the surface related defects created by the thiol group of MPA. However, the PL spectra of all the G2, G3 and G4 nanocomposite samples showed a featureless profile with little or no photoluminescence. A similar PL profile featuring quenching of photoluminescence was observed for the RGO–CdS–PANI nanocomposite with a 0.4 wt% loading of the G4 sample. This observation was found to be highly satisfactory, as complete quenching of the PL peak highlights the effective charge transfer between CdS QDs, RGO sheets and PANI. The excited electrons are expected to be trapped by the surface related defects created by the MPA capping ligand and the holes are expected to enter the Fermi level of RGO. Such efficient transfer of charges from CdS to RGO makes the as-prepared nanocomposites a highly promising candidate for electronic applications.
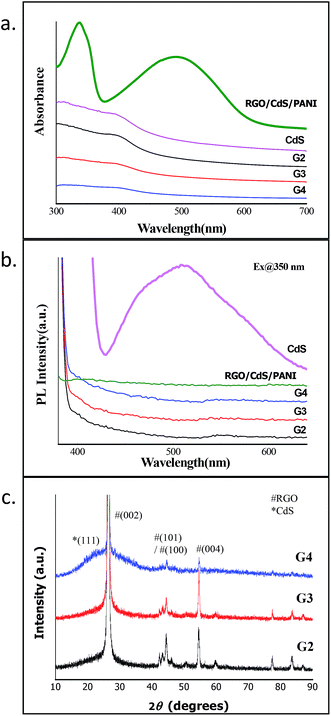 |
| Fig. 1 (a) UV-visible, (b) PL spectra and (c) XRD of RGO–CdS nanocomposites with different concentrations of CdS QDs. | |
The XRD studies of all the RGO–CdS samples showed sharp characteristic peaks corresponding to (002), (100), (101), (004), (103) and (110) crystal planes of graphite (Fig. 1c). The dominance of graphitic peaks was expected due to the high crystalline nature of the stacked RGO sheets. The CdS QDs when synthesized alone showed the broad peak profile highlighting their small size and amorphous nature (Fig. SI. 1†). The broad peak of CdS QDs was evident in the G4 sample where the ratio of RGO
:
CdS was 1
:
0.5 (w/w%). It was obvious that the broad peak profile of CdS QDs was evident in the G4 sample due to the high concentration of CdS QDs present in this sample. The broad peak that was obtained from 20 to 35° for this sample highlights the merging of peaks (111) and (220) of cubic CdS crystal planes. For further confirming the presence of CdS QDs in the nanocomposites, we performed Raman spectroscopy for samples.
In bulk CdS crystals, the rule for the selection of Raman scattering is q ≈ 0, where q is the wave vector. However, in CdS QDs, the selection rule (q ≈ 0) is relaxed due to interference of lattice periodicity. The group theory predicts seven optically active branches at the zone centre in the CdS crystal structure. These optical branches can be classified as symmetric A1 and doubly degenerate E1, both active in Raman and infrared spectra, and two doubly degenerate E2 branches (Raman active only).32,33 In the present study, we observed six broad optical vibrational Raman peaks between approximately 290–480, 750–1000, 1100–1380, 1600–1850, 2000–2200 and 2500–2700 cm−1 in G2, G3 and G4 samples as shown in Fig. 2a. The peaks obtained are broad in nature and hence reflecting the presence of high density photons in the nanocomposites. The presence of E-modes represents high phonon scattering in the sample which may reduce the thermal conductivity in the present nanocomposite system. The RGO–CdS–PANI nanocomposite with a 0.4 wt% loading of the G4 samples showed domination of PANI in the nanocomposite. The ν(CC) band at ca. 1600 cm−1, the imine ν(C
N) and δ(NH) vibration band near 1500 cm−1, the semibenzenoid polaronic ν(C–N+˙) band at 1350 cm−1, and the band of C–H in plane bending vibration around 1200 cm−1 representing PANI were observed.
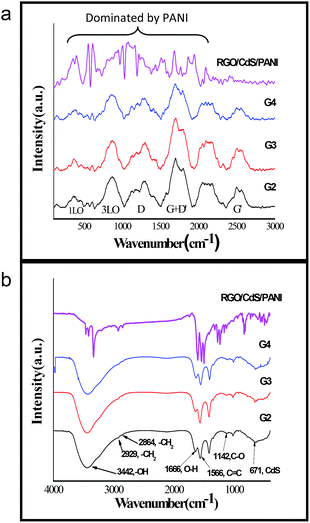 |
| Fig. 2 (a) FTIR and (b) Raman spectra of the as-prepared nanocomposites confirming the presence of CdS, RGO, PANI and MPA. | |
The prime objective of preparing such nanocomposites was to create a linkage between CdS and RGO surfaces which may hold the CdS QDs over RGO sheets. MPA may play a role in such linkage as it has two functional groups in its structure (thiol and carboxylic groups) resulting in a uniform coverage of CdS QDs over RGO. The thiol group is known to form a strong bond with surface Cd atoms on CdS QDs and we assume that the carboxylic group may attach to the RGO surface to deliver such linkage. The FTIR spectra confirmed the presence of MPA in all the samples (Fig. 2a). The relative peaks of carboxylic acids i.e. 1720 cm−1 and two peaks between 1210 and 1320 cm−1 resembling C
O and C–O stretching vibrations were also clearly observed for all the samples. The peak of S–H from thiol (2500–2600 cm−1) was absent in all the samples confirming the bond between the S atom from thiol and the Cd atom from CdS QDs. The FESEM images shown in Fig. 3a–c of the RGO–CdS nanocomposite confirmed the uniform coverage of CdS quantum dots over RGO as the ratio of CdS was increased from G2 to G4. The coverage of CdS QDs over RGO sheets was found to be high in the G4 sample as observed from the SEM image.
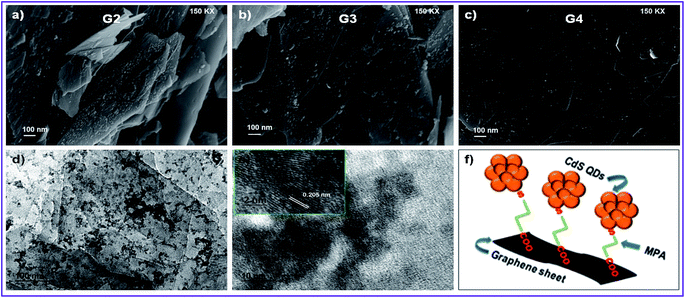 |
| Fig. 3 (a–c) FESEM images of the RGO–CdS nanocomposite with different ratios of CdS and RGO, (d) TEM images of the G4 sample with a scale bar of 100 nm (e) TEM images of the G4 sample with scale bars of 10 nm and 2 nm for the inset, and (f) schematic representation showing MPA linkage between graphene sheets and CdS QDs. | |
The presence of CdS QDs was observed on RGO sheets. The EDX analysis confirmed the increase of elemental Cd and S from G2 to G4 samples (Fig. S4†). The HRTEM images of the G4 sample further confirmed the presence of CdS QDs on RGO sheets and also the exfoliation of RGO sheets (see Fig. 3). It was observed that small aggregates of CdS QDs were uniformly decorated over plane of wrecked RGO nanosheets. The lattice fringes were clearly visible and the lattice spacing of d = 0.205 nm for the (220) crystal plane. The SEM and TEM images of the RGO–CdS–PANI nanocomposite with a 0.4 wt% loading of the G4 sample visibly showed the presence of globular PANI aggregates along with CdS coated RGO sheets in the sample (Fig. 4a). The lattice fringes of CdS QDs were clearly visible in the TEM images which confirmed their existence over RGO sheets (Fig. SI. 5†).
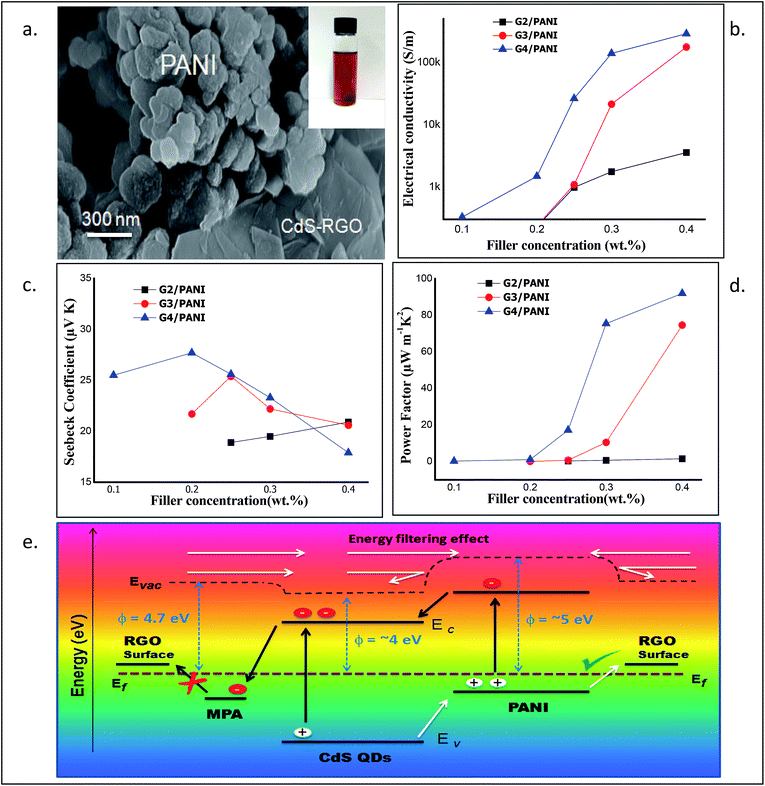 |
| Fig. 4 (a) SEM image of the RGO–CdS–PANI nanocomposite with a 0.4 wt% loading of the G4 sample plus photograph of its re-dispersion in ethanol (inset), plots of (b) electrical conductivity, (c) Seebeck coefficient, (d) power factor versus filler concentration and (e) graphical representation for energy levels of the RGO–CdS–PANI nanocomposite promoting p-type charge transport and energy filtering effect. | |
Thermoelectric application of RGO–CdS in the polyaniline matrix
The electrical conductivity, Seebeck coefficient/thermopower and power factor are the three main components which describe thermoelectric properties. The measurement of electrical conductivity and thermopower was carried out experimentally at room temperature (RT) and under ambient conditions. The power factor (PF) was calculated from the obtained values of S and σ (PF = S2σ). Interestingly, we observed that the electrical conductivity of RGO–CdS–PANI nanocomposites increased with the increase in the filler (G2, G3 & G4) concentration (Fig. 4b). The increase in electrical conductivity was mainly due to addition of RGO–CdS nanocomposites, as pristine PANI showed low electrical conductivity in the range of 10−4 S m−1. The Seebeck coefficient also exhibited an interesting trend as the maximum value was obtained for a sample with 0.2 wt% of G4 loading while the lowest value was obtained for a sample with 0.4 wt% of G4 loading (see Fig. 4c). The PF of the RGO–CdS–PANI nanocomposites increased as the filler concentration increased and reached a very high value (91.95 μW m−1 K−2) for 0.4 wt% loading of G4 (Fig. 4d). This PF value was found to be slightly lower than the highest ever reported value for the PANI based system. Recently, Wang et al. reported a PF of 101 μW m−1 K−2 using a composite of PANI, SWNT's and Te nanorods.43 The present nanocomposite system however, can be more advantageous as a low thermal conductivity (0.14 for 0.4 wt% G4 loading) was obtained as compared to 0.3 for the above-reported material. The low thermal conductivity and high electrical conductivity of the present RGO–CdS–PANI nanocomposite (with 0.4 wt% loading G4) yielded an excellent ZT value of 1.97.
The electrical conductivity in the present nanocomposite system may have followed the percolation law which helps to predict an increase in electrical conductivity till it reaches a critical concentration level of the filler. Generally, when two different materials with a large difference in electrical conductivities are mixed, such a phenomenon is observed. Interestingly, the role of CdS QDs was vital in obtaining high PF values. It should be noted that the concentration of RGO and PANI was kept constant in RGO–CdS and RGO–CdS–PANI nanocomposites respectively. Thus, only the CdS QD concentration was systematically increased in the final RGO–CdS–PANI nanocomposites as the loading of G2, G3 and G4 was increased. The PF value of RGO–CdS–PANI nanocomposites with a 0.4 wt% G3 loading was ∼75 while with a similar G4 loading was ∼92 (Fig. 4d). Thus, the increase in CdS QD concentration (0.25 to 0.5 wt% with respect to RGO) in the as-prepared RGO–CdS–PANI nanocomposites played a vital role in increasing the PF value. Additionally, the increase in CdS QD concentrations (from G2 to G4) also leads to the increase in electrical conductivities of the nanocomposites i.e. samples with G4 loading have the highest values followed by G3 and then G2.
Mechanism and role of various interfaces in charge transport
The most peculiar part of thermoelectric properties is the interdependency of electrical conductivity, Seebeck coefficient, and thermal conductivity. The electrical and thermal conductivity of the thermoelectric material enhances and reduces simultaneously. RGO possess high electrical and thermal conductivity hence; it is not an ideal candidate for thermoelectric applications. In the present case, the decoration with CdS QDs over RGO and the PANI matrix helps to decouple electrical and thermal conductivity in the as-prepared nanocomposites. Since, the thermal conductivities of both CdS and PANI are more than 10 times lower than that of graphene, the present nanocomposites may offer high electrical conductivity but low thermal conductivity. The broadness of the RAMAN peaks of these nanocomposites hinted high scattering of phonons which may lower the thermal conductivity.
In the present study, CdS QDs were prepared over and in between the RGO layers during processing. As a result, the tendency of graphitic stacking in the as-prepared nanocomposite may have reduced. The decoration of CdS QDs obstructs the phonon transport but permits charge transport which further results in a decrease of thermal conductivity. The FESEM images revealed the uniform presence of RGO in all the samples creating a good network of exfoliated RGO sheets. The uniformly distributed RGO increases the interfacial area which facilitates high charge mobility and high carrier transfer between PANI–RGO and CdS–PANI interfaces. However, it has been reported previously that a thiol based capping ligand may form an electron trapping centre by creating an energy level near the valence band of CdS QDs.44 Such an electron trapping centre can be formed due to the MPA capping ligand blocking the electron transport from CdS QDs to RGO. Furthermore, the conduction band of CdS QDs is assumed to be below the LUMO of PANI while the valence band of PANI is situated above the valence band of CdS QDs. The band alignment in the present nanocomposites favours the electron transfer from PANI to CdS QDs and hole transfer from CdS QDs to PANI after thermal or visible light excitation. Such charge transfer will increase the electron and hole density in CdS QDs (conduction band) and PANI (HOMO) respectively. Since, the MPA ligand binds the CdS and RGO together; the excited electrons will not transfer from CdS to RGO due to their difference in energy levels. However, the energy level of RGO is expected to be above the HOMO of PANI which will favour the hole transfer from PANI to RGO (Fig. 4a). Hence, the as-prepared RGO–CdS–PANI nanocomposite behaves like a p-type material which was evident from the positive Seebeck coefficient values.45 Furthermore, the work function of PANI (emeraldine base) is known to be near 5 eV while the work function of CdS and RGO is ∼3.9 and 4.7 eV respectively.46 We assume that a small energy barrier may be present at the PANI/RGO interface. It has been reported that the inorganic/organic hybrid nanocomposites exhibit an energy filtering effect where the carriers possessing high energy cross the energy barrier at the interface. The low energy carriers are not allowed to pass through the interface and thus, leading to enhancement of low electrical conductivity and high Seebeck coefficient.47 In the present ternary nanocomposites, two different interfaces (PANI–RGO and PANI–CdS) are active for the passage of charge carriers. The obvious enhancement in the Seebeck coefficient for the low concentrations of G2, G3 and G4 in RGO–CdS–PANI nanocomposite films may be attributed to the synergetic energy filtering effect at these two interfaces of PANI–RGO and CdS–PANI. However, when the concentration of G2, G3 and G4 was increased in the RGO–CdS–PANI nanocomposite, the Seebeck coefficient decreased. The decreased Seebeck coefficient could be a result of increase in population of high energy charge carriers resulting in higher electrical conductivity. The RGO–CdS–PANI nanocomposites represent a brilliant description in which temperature gradients have been maintained between two edges of the material resulting in generation of electric potential. The schematic representation of the charge transport and the energy filtering effect in the present RGO–CdS–PANI nanocomposite is shown in Fig. 4e. Overall, such a favourable network of the as-prepared RGO–CdS–PANI nanocomposite may promote high electrical conductivity but reduce the thermal conductivity.
To justify the claims of the present work, a summary of the latest thermoelectric reports based on the PANI matrix has been highlighted in Table 1. The as-synthesized RGO–CdS–PANI nanocomposites showed strong re-dispersion in common solvents such as DMF and ethanol which was stable for more than 08 weeks (Fig. SI. 2†). We believe that the strong re-dispersion could be due to the high exfoliation of RGO resulting in small sub-micron sized RGO–CdS nanocomposites (Fig. SI. 3†). These small sized RGO–CdS sheets along with globules of PANI could easily disperse in the solvent medium. The high re-dispersibility of these nanocomposites may be significant for their application in flexible thermoelectric devices where solution processed or printed devices are fabricated. Additionally, the high electrical conductivities (in order of 105 S m−1) and suitable energy band positioning can be vital for utilizing these nanocomposites for various energy and electronic applications.
Table 1 Compilation of the recent reports on thermoelectric properties of PANI based composite materials
PANI based composites for thermoelectric studies |
σ, S m−1 |
S, μV k−1 |
κ, W mK−1 |
Calculated PF (S2σ), μW m−1 K2 |
PANI + SWCNT composites with different SWCNTs34 |
10–125 (RT) |
11–40 (RT) |
0.5–1 (RT) |
0.5–5 (300 K) |
PANI + unoxidized SWCNTs35 |
5.30 × 104 |
33 |
— |
0.6 |
PANI + HCl + MWCNTs (40%)36 |
1.71 × 103 |
10 |
— |
0.17 |
PANI + CNTs (15.8%)37 |
6.1 × 103 |
29 |
0.4–0.5 |
5 |
PANI + HCl + graphene (50%)38 |
123 |
34 |
3.3 |
14 |
PANI + GNP (in situ polymerization with protonation ratio – 0.2) |
|
|
|
ZT (300 K) = |
Neat PANI |
150 |
7 |
0.6 |
3.68 × 10−6 |
Neat GNP |
2 × 104 |
5 |
74 |
3.04 × 10−6 |
PANI/GNP (50 mM, as made) |
5900 |
33 |
13 |
1.51 × 10−4 |
PANI/GNP (50 mM, reprotonated)39 |
1.74 × 104 |
19 |
15 |
1.26 × 10−4 |
PANI + 30% graphene (in situ polymerization)40 |
5 × 103 at 323 K |
12 |
— |
ZT = 1.95 × 10−3 at 453 K |
PANI at 420 K |
500 |
13 |
— |
0.1 |
PANI + 5–30% graphene41 |
700–4.0 × 103 |
28–32 |
— |
0.4–2.6 |
PANI + HClO4 + graphite (50 wt%)42 |
1.2 × 104 |
19 |
1.2 |
1.2 |
PANI/SWNTs/Te nanorods43 |
3.45 × 102 |
54 |
0.3 |
101 |
Present work
|
|
|
|
|
PANI + 0.4 wt% RGO–CdS |
|
|
|
|
G2 |
3.6 × 103 |
20 |
0.58 |
1.6 (ZT = 8.4 × 10−3) |
G3 |
1.7 × 105 |
21 |
0.27 |
∼75 (ZT = 0.833) |
G4 |
2.9 × 105 |
18 |
0.14 |
∼92 (ZT = 1.97) |
Conclusion
RGO–CdS–PANI nanocomposites with varying ratios were prepared by two step method where CdS QDs were first prepared in presence of RGO. Later, PANI–RGO–CdS nanocomposites were obtained by varying the ratio of RGO–CdS with respect to PANI. The characterization techniques revealed the uniform coverage of CdS QDs on RGO sheets, as MPA facilitated a linkage between RGO and CdS QDs. The Raman analysis of the nanocomposites hinted at a high phonon scattering supporting a low thermal conductivity. The band alignment between RGO, CdS QDs and PANI promoted p-type electrical conductivity and demonstrated a synergetic energy effect for charge transport. We observed that the increase in concentration of CdS QDs and RGO in the final RGO–CdS–PANI nanocomposites resulted in enhanced electrical conductivity and thermopower (power factor). The role of MPA capped CdS QDs was vital in facilitating hole transport (and prohibiting electron transport) to impart p-type behaviour to the nanocomposites. The obtained power factor values (at RT) for 0.4 wt% loading of the RGO–CdS nanocomposite in the PANI matrix are among the highest reported values for PANI based TE materials. The present RGO–CdS–PANI nanocomposites can be considered a highly suitable material for flexible thermoelectric devices or fabrics due to their high re-dispersion and easy processing.
Conflicts of interest
There are no conflicts of interest to declare.
Acknowledgements
We thank the Vice Chancellor (DIAT) for support. PM thanks the DST, Govt. of India for a research grant through DST INSPIRE AWARD project no. IFA-13-PH-68.
Notes and references
- H. J. Goldsmid, Materials, 2014, 7, 2577–2592 CrossRef CAS PubMed.
- L. E. Bell, Science, 2008, 321, 1457–1461 CrossRef CAS PubMed.
- G. Chen, M. Dresselhaus, G. Dresselhaus, J. Fleurial and T. Caillat, Int. Mater. Rev., 2013, 48, 45–66 CrossRef.
-
D. M. Rowe, CRC Handbook of Thermoelectrics, CRC Taylor and Francis, Boca Raton, FL, USA, 2012, vol. 16, pp. 1–33 Search PubMed.
- L. D. Zhao, J. He, D. Berardan, Y. Lin, J. F. Li, C. W. Nanc and N. Dragoe, Energy Environ. Sci., 2014, 7, 2900–2924 CAS.
- M. Zebarjadi, K. Esfarjani, M. S. Dresselhaus, Z. F. Ren and G. Chen, Energy Environ. Sci., 2012, 5, 5147–5162 Search PubMed.
- C. J. Vineis, A. Shakouri, A. Majumdar and M. G. Kanatzidis, Adv. Mater., 2010, 22, 3970–3980 CrossRef CAS PubMed.
- S. K. Yee, S. LeBlanc, K. E. Goodson and C. Dames, Energy Environ. Sci., 2013, 6, 2561–2571 Search PubMed.
- N. Toshima, Macromol. Symp., 2002, 186, 81–86 CrossRef CAS.
-
(a) M. G. Kanatzidis, Chem. Mater., 2010, 22, 648 CrossRef CAS;
(b) J. R. Sootsman, D. Y. Chung and M. G. Kanatzidis, Angew. Chem., Int. Ed., 2009, 48, 8616 CrossRef CAS PubMed;
(c) M. G. Kanatzidis, Semicond. Semimetals, 2000, 69, 51 Search PubMed;
(d) C. J. Vineis, A. Shakouri, A. Majumdar and M. G. Kanatzidis, Adv. Mater., 2010, 22, 3970 CrossRef CAS PubMed.
-
(a) J. G. Snyder and E. S. Toberer, Nat. Mater., 2008, 7, 105 CrossRef PubMed;
(b) M. S. Dresselhuas, G. Chen, M. Tang, R. Yang, H. Lee, D. Wang, Z. Ren, J. Fleurial and P. Gogna, Adv. Mater., 2007, 19, 1043 CrossRef;
(c) M. Zebarjadi, K. Esfarjani, M. S. Dresselhaus, Z. F. Ren and G. Chen, Energy Environ. Sci., 2012, 5, 5147 RSC;
(d) D. Bilc, S. D. Mahanti, E. Quarez, K. F. Hsu, R. Pcionek and M. G. Kanatzidis, Phys. Rev. Lett., 2004, 93, 146403 CrossRef PubMed.
- V. M. Agranovich, Y. N. Gartstein and M. Litinskaya, Chem. Rev., 2011, 111, 5179–5214 CrossRef CAS PubMed.
- B. T. McGrail, A. Sehirlioglu and E. Pentzer, Angew. Chem., Int. Ed., 2015, 54, 1710–1723 CrossRef CAS PubMed.
- C. Janáky and K. Rajeshwar, Prog. Polym. Sci., 2015, 43, 96–135 CrossRef.
- Y. Du, S. Z. Shen, K. Cai and P. S. Casey, Prog. Polym. Sci., 2012, 37, 820–841 CrossRef CAS.
- J. Dong, W. Liu, H. Li, X. Su, X. Tang and C. Uher, J. Mater. Chem. A, 2013, 1, 12503–12511 CAS.
- B. Feng, J. Xie, G. Cao, T. Zhu and X. Zhao, J. Mater. Chem. A, 2013, 1, 13111–13119 CAS.
- M. Jorgensen, K. Norrman, S. A. Gevorgyan, T. Tromholt, B. Andreasen and F. C. Krebs, Adv. Mater., 2012, 24, 580–612 CrossRef PubMed.
- G. Qian, Z. Zhong, M. Luo, D. Yu, Z. Zhang, Z. Y. Wang and D. Ma, Adv. Mater., 2009, 21, 111–116 CrossRef CAS.
- L. Wang, A. Perez, S. N. Patel, L. Ying, E. J. Kramer, T. Q. Nguyen and G. C. Bazan,
et al.
, Adv. Mater., 2014, 26, 2993–2998 CrossRef PubMed.
- L. H. Xie, Q. D. Ling, X. Y. Hou and W. Huang, J. Am. Chem. Soc., 2008, 130, 2120–2121 CrossRef CAS PubMed.
- J. Wu, Y. Sun, W. B. Pei, L. Huang, W. Xu and Q. Zhang, Synth. Met., 2014, 196, 173–177 CrossRef CAS.
- C. T. Hong, W. Lee, Y. H. Kang, Y. Yoo, J. Ryu, S. Y. Cho and K. S. Jang, J. Mater. Chem. A, 2015, 3, 12314–12319 CAS.
- Q. Yao, L. Chen, W. Zhang, S. Liufu and X. Chen, ACS Nano, 2010, 4, 2445–2451 CrossRef CAS PubMed.
- D. Kim, Y. Kim, K. Choi, J. D. Grunlan and C. Yu, ACS Nano, 2010, 4, 513–523 CrossRef CAS PubMed.
- M. Jorgensen, K. Norrman, S. A. Gevorgyan, T. Tromholt, B. Andreasen and F. C. Krebs, Adv. Mater., 2012, 24, 580–612 CrossRef PubMed.
- G. Qian, Z. Zhong, M. Luo, D. Yu, Z. Zhang, Z. Y. Wang and D. Ma, Adv. Mater., 2009, 21, 111–116 CrossRef CAS.
- H. R. Tseng, H. Phan, C. Luo, M. Wang, L. A. Perez, S. N. Patel, L. Ying, E. J. Kramer, T. Q. Nguyen and G. C. Bazan,
et al.
, Adv. Mater., 2014, 26, 2993–2998 CrossRef CAS PubMed.
- L. H. Xie, Q. D. Ling, X. Y. Hou and W. Huang, J. Am. Chem. Soc., 2008, 130, 2120–2121 CrossRef CAS PubMed.
- M. He, F. Qiu and Z. Lin, Energy Environ. Sci., 2013, 6, 1352–1361 Search PubMed.
- A. Dey, J. Athar, P. Varma, H. Prasant, A. K. Sikder and S. Chattopadhyay, RSC Adv., 2015, 5, 1950 RSC.
- R. J. Briggs and A. K. Ramdas, Phys. Rev. B: Solid State, 1976, 13, 5518–5529 CrossRef CAS.
- B. Tell, T. C. Damen and S. P. S. Porto, Phys. Rev., 1966, 144, 771–774 CrossRef CAS.
- G. P. Moriarty, J. N. Wheeler, C. Yu and J. C. Grunlan, Carbon, 2012, 50, 885 CrossRef CAS.
- Q. Yao, L. D. Chen, W. Q. Zhang, S. C. Liufu and X. H. Chen, ACS Nano, 2010, 4, 2445 CrossRef CAS PubMed.
- R. C. Y. King, F. Roussel, J. F. Brun and C. Gors, Synth. Met., 2012, 162, 1348 CrossRef CAS.
- Q. Wang, Q. Yao, J. Chang and L. Chen, J. Mater. Chem. A, 2012, 22, 17612 RSC.
- Y. Du, S. Z. Shen, W. Yang, R. Donelson, K. Cai and P. S. Casey, Synth. Met., 2012, 161, 2688–2692 CrossRef.
- B. Abad, I. Alda, P. D. Chao, H. A. Kawakami, D. Almarza, D. L. Amantia, D. Gutierrez, L. Aubouy and M. Martin-Gonzalez, J. Mater. Chem. A, 2013, 1, 10450 CAS.
- J. Xiang and T. D. Lawrence, Polymer, 2012, 53, 4202 CrossRef CAS.
- Y. Lu, Y. Song and F. Wang, Mater. Chem. Phys., 2013, 138, 238–244 CrossRef CAS.
- L. Wang, D. Wang, G. Zhu, J. Li and F. Pan, Mater. Lett., 2011, 65, 1086 CrossRef CAS.
- L. Wang, Q. Yao, W. Shi, S. Qu and L. Chen, Materials Chemistry Frontiers, 2017, 1, 741 RSC.
- S. F. Wuister, C. deMello Donegá and A. Meijerink, J. Phys. Chem. B, 2004, 108, 17393–17397 CrossRef CAS.
- J. P. Moore, J. Appl. Phys., 1973, 44(3), 1174 CrossRef CAS.
- J. Park, D. Bang, K. Jang, S. Haam, J. Yang and S. Na, Nanotechnology, 2012, 23, 365705 CrossRef PubMed.
- K. C. See, J. P. Feser, C. E. Chen, A. Majumdar, J. J. Urban and R. A. Segalman, Nano Lett., 2010, 10, 4664 CrossRef CAS PubMed.
Footnote |
† Electronic supplementary information (ESI) available: Measurement methods for thermoelectric and electrical properties of these nanocomposites, the XRD spectrum of CdS QDs alone, photograph of DMF re-dispersion and SEM image of an RGO–CdS–PANI nanocomposite with a 0.4 wt% loading of G4. See DOI: 10.1039/c7se00290d |
|
This journal is © The Royal Society of Chemistry 2017 |